DOI:
10.1039/D0RA00732C
(Paper)
RSC Adv., 2020,
10, 7004-7010
Two 1D homochiral heterometallic chains: crystal structures, spectra, ferroelectricity and ferromagnetic properties†
Received
23rd January 2020
, Accepted 9th February 2020
First published on 14th February 2020
Abstract
Two new homo chiral Cu–Ln (Ln = Gd and Ho) compounds bearing a chiral Schiff base ligand (1R,3S)-N′,N′′-bis[3-methoxysalicylidene]-1,3-diamino-1,2,2-trimethylcyclopentane (H2L) have been synthesized and characterized by elemental analysis, IR spectroscopic and single-crystal X-ray diffraction techniques. The compounds were found to exhibit 1D zig-zag skeletons with double μ-1,5 bridging dicyanamide anions. Circular dichroism (CD) spectra have been used to verify their chiroptical activities. Magnetic studies suggest that 1 and 2 hold the same magnetic behavior with the dinuclear compounds presenting ferromagnetic interaction. Furthermore, both compounds show ferroelectricity with the remnant polarization (Pr) value of 0.23 and 0.18 μC cm−2 at room temperature, respectively.
Introduction
The rational design and synthesis of heterometallic 3d–4f compounds have attracted wide spread attention in the field of coordination chemistry owing to their fascinating architectures and potential applications in luminescence, catalysis, and molecular magnetism.1–3 In molecular magnetism, the intermediate magnetic coupling between 3d–4f ions may effectively suppress the zero-field quantum tunneling mechanism (QTM) thereby improving the energy barrier for spin reversal.4 Thus, single-molecule magnets (SMMs) with large energy barriers can be easily obtained by combining paramagnetic 3d-metal ions with highly anisotropic lanthanide ions,5 as in the case of [Mn6IIIO3(saO)6(OCH3)6Tb2(CH3OH)4(H2O)2] (saOH2 = salicylaldoxime) and [Dy2Co2(L)4(NO3)2(DMF)2]·2DMF (H2L = (E)-2-ethoxy-6-(((2-hydroxyphenyl)imino)methyl)phenol) with energy gaps of 103 K and 125 K, respectively.6 On the other hand, considering the ferromagnetic exchange interaction between CuII and LnIII ions, their compounds (Cu–Gd) have generated special interest in magnetic refrigerants.7 Furthermore, much higher effective energy barriers and blocking temperatures have been achieved in lanthanide-containing SMMs as compared to most discovered transition metal-based SMMs.3 There are several reports on multitudinous molecular structures of 3d–4f heterometallic compounds with attractive topologies and multifarious interesting magnetic properties to date,8 with relatively few studies devoted to the exploration of multifunctional 3d–4f compounds combining ferroelectricity, optical activity, and ferromagnetic properties.9
It is generally accepted that chiral metal coordination compounds with large net dipole moments might possess desirable ferroelectric properties, when crystallized in polar space groups.10 In order to synthesize optically active compounds, researchers have widely use chiral Schiff-base ligands to transfer chiral information to the assemblies.11 In our previous works, we have reported on a series of copper compounds with chiral Schiff-base ligands derived from camphoric diamine and different aromatic aldehydes which revealed that homochirality can be kept in 1D chain and 2D network by changing the substituent groups on aromatic rings of aldehydes.12,13 In continuation of our studies on homochiral coordination polymers based on camphoric diamine Schiff-base ligands, we strive to exploit new multifunctional molecular-based materials exhibiting optical activity, magnetism and ferroelectricity in one molecule. In the present work, we report on two novel homochiral 1D heterometallic Cu–Ln coordination polymers namely, [LCuLn(dca)2(NO3)]n [dca = dicyanamide anion, Ln = Gd (1) and Ho (2)] using a new chiral Schiff base ligand (1R,3S)-N′,N′′-bis[3-methoxysalicylidene]-1,3-diamino-1,2,2-trimethyl-cyclopentane (H2L) (Scheme 1) and a dicyanamide anion linkage exhibiting optical activity, ferroelectricity and ferromagnetic properties.
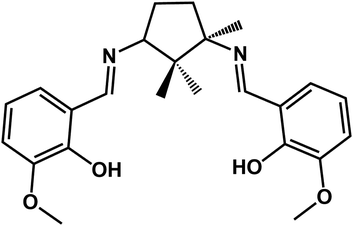 |
| Scheme 1 Chemical structure of H2L. | |
Experimental
Materials and methods
All chemicals used were of analytical grade and obtained from commercial sources without further purification. Schiff base ligand H2L was prepared according to the literature methods using D-(+)-camphor as the starting materials.14 Building blocks [LCuLn(NO3)3]·Me2CO were prepared according to the literature methods.15 FT-IR spectra were recorded on a Nicolet Avatar A370 spectrometer using KBr pellets in the 400–4000 cm−1 region. Elemental analyses for carbon, hydrogen and nitrogen were carried out on a Vario EL III elemental analyzer. UV-vis spectra were performed with a Puxi TU-1900 spectrometer with a 1.0 cm quartz cell in DMSO solvent. Circular dichroism spectra were measured with a JASCO J-815 spectro polarimeter using the same solutions as those for the UV-vis determination. The P–E hysteresis loops of 1 and 2 were measured on a Ferroelectric Tester Precision Premier II made by Radiant Technologies Inc. at room temperature based on the compressed powder sample. Variable-temperature magnetic susceptibility measurements were taken at an applied field of 100 Oe on a Quantum Design MPMS-XL7 SQUID magnetometer working in the temperature range of 300–1.8 K. The molar magnetic susceptibilities were corrected for the diamagnetism estimated from Pascal's tables and for sample holder by previous calibration.
Synthetic procedures
Synthesis of [LCuLn(dca)2(NO3)]n [Ln = Gd (1) and Ho (2)]. A similar procedure was adopted to prepare compounds 1 and 2.
[LCuGd(dca)2(NO3)]n (1). Na(dca) (0.7 mmol, 62.4 mg) solid was slowly added to a 10 mL methanolic solution [LCuGd(NO3)3]·Me2CO (0.1 mmol, 87.3 mg) with continuous stirring for 30 min. The mixture was then filtered and kept at room temperature undisturbed for slow evaporation. After two days, green crystals of 1 were obtained and washed with ether. The yield was about 42% based on Gd(III). Elemental analysis calcd (%) for C28H28CuGdN9O7: C, 40.81; H, 3.401; N, 15.30. Found: C, 40.42; H, 3.388; N, 15.28. Selected IR data (KBr, cm−1): 3440m, 3065w, 2977w, 2294s, 2226s, 2171s, 1616s, 1564m, 1478s, 1351m, 1296s, 1246s, 1223s, 1172w, 1081w, 975w, 854w, 784w, 743m.
[LCuHo(dca)2(NO3)]n (2). The synthetic procedure of compound 2 was similar to compound 1, except that [LCuHo(NO3)3]·Me2CO was used. The yield was 24% based on Ho(III). Elemental analysis calcd (%) for C28H28CuHoN9O7: 40.43; H, 3.369; N, 15.16. Found: C, 40.38; H, 3.384; N, 15.48. Selected IR data (KBr, cm−1): 3440m, 3065w, 2978w, 2294s, 2225s, 2170s, 1616s, 1563m, 1477s, 1351m, 196s, 1245s, 1223s, 1172w, 1081w, 974w, 854w, 784w, 743m.
Crystallography
The perfect crystals of compounds 1 and 2 were carefully chosen to determine the X-ray diffraction. The crystal data were collected on a Bruker Smart Apex-II CCD diffractometer at room temperature. Intensities were collected with graphite monochromatized Mo-Kα radiation (λ = 0.71073 Å), using the φ and ω scan technique. The data reduction was made with SAINT package. Absorption corrections were performed using SADABS program. The structures were solved by the direct methods and refined on F2 by full-matrix least-squares using SHELXTL-2014 program package with anisotropic displacement parameters for all non-hydrogen atoms. Hydrogen atoms were introduced in calculations using the riding model. The crystal data and structural refinement results are summarized in Table 1.
Table 1 Crystallographic data and details of refinements for 1 and 2
R1 = ∑||Fo| − |Fc||/∑|Fo|. wR2 = [∑w(|Fo2| − |Fc2|)2/∑w(|Fo2|)2]1/2. |
Compounds |
1 (CuGd) |
2 (CuHo) |
Empirical formula |
C28H28CuGdN9O7 |
C28H28CuHoN9O7 |
Formula weight |
823.38 |
831.06 |
Crystal system |
Monoclinic |
Monoclinic |
Space group |
P21 |
P21 |
a (Å) |
9.8268(7) |
9.862(3) |
b (Å) |
15.4881(11) |
15.473(5) |
c (Å) |
10.5137(7) |
10.493(3) |
β (°) |
101.4330(10) |
101.542(4) |
V (Å3) |
1568.42(19) |
1568.8(8) |
Z |
2 |
2 |
ρcalcd (g cm−3) |
1.743 |
1.759 |
μ (mm−1) |
2.834 |
3.241 |
F(000) |
816 |
822 |
GOF (F2) |
1.083 |
1.075 |
Flack parameter |
0.012(14) |
0.015(7) |
R1a [I > 2σ(I)] |
0.0237 |
0.0166 |
wR2b [I > 2σ(I)] |
0.0738 |
0.0460 |
Results and discussion
Synthesis
For the design of multiferroic compounds 1 and 2, the ferroelectric property in the chiral ferromagnetic CuIILnIII complexes, was induced by utilizing camphoric diamine Schiff base ligands. To retain the ferromagnetic properties in compounds, dicyanamide (dca) anions were employed as bridges, due to their weak magnetic exchange interaction in μ-1,5 coordination mode.16 Additionally, the dca anions were used to replace parts of coordinated nitrate anions for generating electric dipolar moments. With these considerations in mind, the molar ratio of [LCuGd(NO3)3]·Me2CO and Na(dca) were controlled in the reaction. Compounds 1 and 2 were obtained, when the metal–ligand ratios ranged from 1
:
4 to 1
:
8. When the metal–ligand ratio was greater than 1
:
9, 2D achiral compounds were obtained, as such the metal–ligand ratios of 1
:
7 were selected for synthesis of 1 and 2.
Crystal structures of 1 and 2
The single crystal X-ray diffraction analyses revealed that compounds 1 and 2 are isomorphous and as such, only the structure of 1 is discussed in detail herein. Compound 1 crystallizes in the monoclinic P21 space group and consists of a 1D infinite chain. As shown in Fig. 1, the asymmetric unit is composed of a dinuclear [LCuGd]3+ moiety, one nitrate anion, and two bridgings dca. The [LCuGd] unit preserves the structural features of the whole [CuLn] family of binuclear complexes in which Cu(II) and Gd(III) ions are connected by two μ2-phenoxo oxygen atoms (O2 and O3).15,17 The copper(II) ion with a distorted square pyramidal geometry is hosted within the inner N2O2 compartment which formed the basal plane. The apical site is occupied by nitrogen atom from dca group (Cu1–N5A 2.280(8) Å, Table 2). While the Gd(III) ion occupies the outer O4 cavity surrounded by six oxygen and three nitrogen atoms arising from the L2− ligand, from one nitrate, and from the three dca anions, respectively. The Cu–Gd separation of 3.4884(9) Å is same as that in previously reported works.18 The two Gd–O bonds involving the –OMe side arms (2.591(5) and 2.518(6) Å) are longer than the ones from the phenolate oxygens (2.305(4) and 2.369(6) Å). The two Gd–O(nitrate) bonds have lengths of 2.518(7) and 2.510(7) Å, whereas the Gd–N bond lengths vary from of 2.436(7) to 2.485(8) Å.
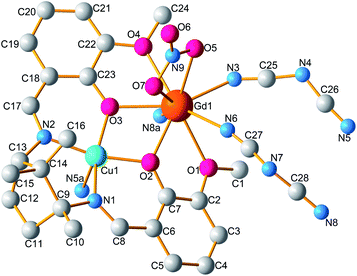 |
| Fig. 1 Molecular structure of 1. For clarity, hydrogen atoms are omitted. (Symmetry codes: (a) 1 − x, 1/2 + y, 2 − z). | |
Table 2 Selected bond distances (Å) and bond angles (°) for 1 and 2a
1 |
2 |
Symmetry codes: (A) 1 − x, 1/2 + y, 2 − z. |
Cu1–N1 |
2.047(7) |
Cu1–N1 |
2.040(5) |
Cu1–N2 |
1.962(7) |
Cu1–N2 |
1.964(5) |
Cu1–N5A |
2.280(8) |
Cu1–N5A |
2.295(6) |
Cu1–O2 |
1.946(7) |
Cu1–O2 |
1.946(5) |
Cu1–O3 |
1.976(5) |
Cu1–O3 |
1.970(4) |
Gd1–N3 |
2.436(7) |
Ho1–N3 |
2.399(5) |
Gd1–N6 |
2.485(8) |
Ho1–N6 |
2.465(6) |
Gd1–N8A |
2.461(8) |
Ho1–N8A |
2.436(6) |
Gd1–O1 |
2.591(5) |
Ho1–O1 |
2.578(4) |
Gd1–O2 |
2.305(4) |
Ho1–O2 |
2.272(3) |
Gd1–O3 |
2.369(6) |
Ho1–O3 |
2.340(4) |
Gd1–O4 |
2.518(6) |
Ho1–O4 |
2.506(4) |
Gd1–O5 |
2.518(7) |
Ho1–O5 |
2.483(5) |
Gd1–O7 |
2.510(7) |
Ho1–O7 |
2.479(5) |
Cu1–Gd1 |
3.4884(9) |
Cu1–Ho1 |
3.4538(10) |
Cu1–O2–Gd1 |
110.0(2) |
Cu1–O2–Ho1 |
109.7(2) |
Cu1–O3–Gd1 |
106.5(2) |
Cu1–O3–Ho1 |
106.19(18) |
Each [LCuGd(NO3)]2+ unit connects other symmetry-related dinuclear centres by two dca groups with μ-1,5 bridge mode, forming a 1D infinite zig-zag chain along b-axis (Fig. 2). In the chain, the neighbouring [LCuGd(NO3)]2+ separations are 8.7690(5), and 7.8696(4) Å for Gd⋯Gd, and Gd⋯Cu, respectively. While, the nitrate anions act as a chelating bidentate mode with one terminal oxygen atom toward outside of the chain. Adjacent chains are expended into a 2D layer through weak hydrogen bonds (Fig. S1, ESI†). Furthermore, the 2D layer is linked each other to form a 3D supramolecular framework by weak interaction (Fig. S2, ESI†).
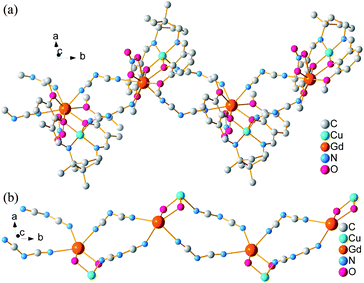 |
| Fig. 2 Zig-zag chain (a) and skeleton (b) of 1 along b-axis. Hydrogen atoms are deleted for clarity. | |
Spectra characterizations
The infrared spectra of 1 and 2 (Fig. S3, ESI†) are both in accordance with their structural characteristics as revealed by single-crystal X-ray diffraction. Three strong peaks of dicyanamide ligand at 2294, 2226, and 2171 cm−1 are observed in compound 1, corresponding to the νas + νs combination modes, νas and νs of –C
N fragments, respectively. The spectra bands displaying at 3065, 1616 and 784 cm−1 are respectively assigned to ν(C–H), ν(C
C) stretching vibrations and δ(C–H) out-of-plane bending vibration of phenyl groups.19 Whereas, weak ν(C–H) stretching vibrations of methyl groups appear near 2977 cm−1. The band at 1564 cm−1 is designated to the –C
N stretching vibration of the Schiff base ligand. In addition, there are four absorptions of coordinated nitrate in 1478 (ν4), 1296 (ν1), 975 (ν2), and 854 cm−1 (ν3). The peak near 1296 cm−1 is the superposition of bands of phenoxy and nitrate. The difference between ν1 and ν4 is about 200 cm−1 (182 cm−1), which indicates that nitrate adopted a chelating bidentate coordination mode,20 in agreement with the crystal structure of 1.
The UV-vis spectra of free ligand H2L and compound 1 in DMSO solutions are shown in Fig. 3. The sharp band at 267 cm−1 of L2− is attributed to aromatic π–π* intraligand charge transfer transition which are shifted down to 280 cm−1 in 1. Another typical band appeared at 329 cm−1 considered as L → M charge transfer transition band which is increased to 358 cm−1 due to metal-ion complexation.21 To certify the optical activity of 1, circular dichroism (CD) spectra were carried out in DMSO solution. In the CD spectra (the inset in Fig. 3), negative Cotton effects at 288 and 390 cm−1 have been observed in the UV-vis region, which is a good agreement between the CD and UV-vis spectra. The CD spectra confirm the homochirality of 1.22
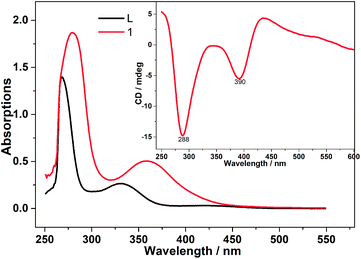 |
| Fig. 3 UV-vis spectra of the Schiff-base ligands (H2L) and compound 1 (4.93 × 10−4 mM) in DMSO solutions together with the corresponding CD spectra (the inset). | |
Magnetic properties
Temperature-dependence molar susceptibility measurements of the crystalline sample of 1 and 2 were carried out on a Quantum Design MPMS-XL7 SQUID magnetometer in an applied magnetic field of 100 Oe over the temperature range 1.8–300 K. Plots of χMT versus T for 1 and 2 are depicted in Fig. 4. At room temperature, the χMT value of 1 is 8.62 emu K mol−1, which is slightly higher than the calculated spin-only value of 8.25 emu K mol−1 based on uncoupled one copper(II) and one gadolinium(III) cations (assuming SCu(II) = 1/2, SGd(III) = 7/2, and g = 2.0). With the system cooling, the χMT product steadily increases until it reaches a maximum value of 10.09 emu K mol−1 at 8 K. This magnetic behaviour indicates a ferromagnetic coupling between copper(II) and gadolinium(III) metal centers through double μ2-phenoxo oxygen bridges.15,17 On further lowering of the temperature, the χMT plot undergoes a slightly decrease with a value of 10.00 emu K mol−1 at 1.8 K closed to the expected value (10 emu K mol−1) for total spin state ST = 4, which may be due to magnetic saturation and/or crystal field splitting of the GdIII ion.23 For 2, the χMT value is 11.26 emu K mol−1, which is lower than the theoretical value of 14.42 emu K mol−1 for one CuII and one HoIII ions. As the temperatures decrease, the χMT slowly decreases down to the minimum values of 6.09 emu K mol−1 at 1.8 K, which may be caused by antiferromagnetic couplings between the CuII and HoIII ions and/or the spin–orbit couplings of the HoIII ions. The magnetic behaviour of 2 is same as those of previously reported Cu–Ho dinuclear complexes with other Schiff-base ligands.24
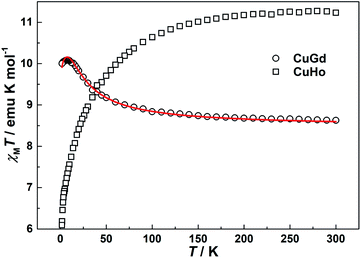 |
| Fig. 4 Temperature dependence of magnetic susceptibilities of 1 (CuGd) and 2 (CuHo) under an applied field of 100 Oe. Solid line represents the best fit of the data. | |
In the two compounds, the dca anion adopts bidentate μ-1,5 bridging mode, which is the most frequently observed coordination mode. Because of its long five-atomic exchange pathway, the μ-1,5 bridging dca anion exhibits only weak magnetic exchange interactions and cooperative phenomena are not observed in any of these compounds in documents.16 Thus, the magnetic properties of 1 and 2 can be considered as dinuclear units. Based on the isotropic Hamiltonian Ĥ = −2JŜCu·ŜGd, where J is the coupling constants mediated by phenoxo in the dinuclear, the experimental data of 1 in the whole temperature range were fitted to the Bleaney–Bowers equation modified by Kahn and co-workers.25 The expression is written as follows when the mean-field corrections zj′ is considered:
χM = χ/[1 − (2zj′/Ng2β2)χ] |
Using this rough model, the magnetic susceptibilities of 1 was simulated, giving the best fit with parameters g = 2.026(1), J = 3.39(9) cm−1, zj′ = −0.0034(2) cm−1, with R = ∑[(χMT)calc − (χMT)obs]2/∑(χMT)obs2 = 1.5 × 10−3. The results indicate the ferromagnetic coupling between metal ions mediated double μ2-phenoxo oxygen bridges. The value of J is comparable with those reported previously in dinuclear CuII–GdIII systems.15,17
Ferroelectric properties
It is well known that compounds crystallizing in the polar point groups may display ferroelectric behaviour.26 Compounds 1 and 2 crystallize in the chiral space group P21 belonging to C2 polar point group, which meets the basic requirement of ferroelectric properties. The ferroelectric data of 1 and 2 were collected at room temperature with powder pellet samples. As shown in Fig. 5, both compounds exhibit well-shaped P–E hysteresis loop which is the ferroelectric features. For 1, the remnant polarization (Pr) value is about 0.23 μC cm−2 at an applied electric field of 18.18 kV cm−1 with a coercitive field (Ec) value of about 9.50 kV cm−1. And the saturation value of spontaneous polarization (PS) is about 0.35 μC cm−2. Whilst, we obtained Pr = 0.18 μC cm−2, Ec = 5.75 kV cm−1, and PS = 0.30 μC cm−2 for 2. By comparison with those of other recently reported molecular ferroelectrics, the PS of 1 and 2 are mediocre,27 but slightly larger than that of classical organic–inorganic ferroelectrics NaKC4H4O6·4H2O (PS = 0.25 μC cm−2). According to the structures of 1 and 2, the ferroelectricity may originate from the off-centering of charges between [LCuLn]3+ dinuclear and nitrate anion, which leads to spontaneous electric dipolar moments occurring. Under an applied electric field, these dipolar moments are arranged in the same direction, which results in the appearance of ferroelectric behaviour in 1 and 2.
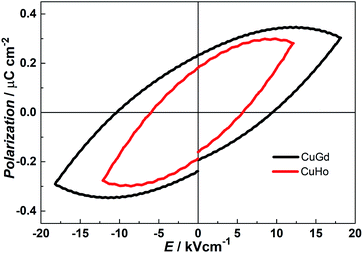 |
| Fig. 5 P–E hysteresis loops of 1 (CuGd) and 2 (CuHo) based on a compressed powder sample at room temperature. | |
Conclusions
In conclusion, we have designed, synthesized, and successfully characterized two new one-dimensional homochiral Cu–Ln (Ln = Gd and Ho) compounds bearing a chiral Schiff base ligand. Both the compounds exhibit ferroelectric and magnetic properties indicating that, the homochiral 1 and 2 are potential molecule-based multifunctional materials coexisting optical activity, ferromagnetic and ferroelectric properties in one molecule. With this synthetic strategy, molecule-based materials with fascinating multifunctionality can be obtained conveniently.
Conflicts of interest
There are no conflicts to declare.
Acknowledgements
This work was supported by Natural Science Foundation of Shanghai (16ZR1411400 and 17ZR1410600) and National Natural Science Foundation of China (21361012 and 21661016).
Notes and references
-
(a) C. Y. Chow, E. R. Trivedi, V. Pecoraro and C. M. Zaleski, Comments Inorg. Chem., 2015, 35, 214–253 CrossRef CAS;
(b) N. Dwivedia, S. K. Panja, A. Verma, T. Takaya, K. Iwata, S. S. Sunkari and S. Saha, J. Lumin., 2017, 192, 156–165 CrossRef;
(c) L. Li, J.-Y. Zou, S.-Y. You, H.-M. Cui, G.-P. Zeng and J.-Z. Cui, Dalton Trans., 2017, 46, 16432–16438 RSC;
(d) W. Huang, S. Huang, M. Zhang, Y. Chen, G.-L. Zhuang, Y. Li, M.-L. Tong, J. Yong, Y. Lia and D. Wu, Chem. Commun., 2018, 54, 4104–4107 RSC;
(e) R. Zhang, L. Wang, C. Xu, H. Yang, W. Chen, G. Gao and W. Liu, Dalton Trans., 2018, 47, 7159–7165 RSC;
(f) L. Zhong, M. Liu, B. Zhang, Y. Sun and Y. Xu, Chem. Res. Chin. Univ., 2019, 35, 693–699 CrossRef CAS.
-
(a) F. Evangelisti, R. Moré, F. Hodel, S. Luber and G. R. Patzke, J. Am. Chem. Soc., 2015, 137, 11076–11084 CrossRef CAS PubMed;
(b) K. Griffiths, A. C. Tsipis, P. Kumar, O. P. E. Townrow, A. Abdul-Sada, G. R. Akien, A. Baldansuren, A. C. Spivey and G. E. Kostakis, Inorg. Chem., 2017, 56, 9563–9573 CrossRef CAS PubMed;
(c) S. I. Sampani, S. Aubert, M. Cattoen, K. Griffiths, A. Abdul-Sada, G. R. Akien, G. J. Tizzard, S. J. Coles, S. Arseniyadis and G. E. Kostakis, Dalton Trans., 2018, 47, 4486–4493 RSC;
(d) L. Wang, C. Xu, Q. Han, X. Tang, P. Zhou, R. Zhang, G. Gao, B. Xu, W. Qin and W. Liu, Chem. Commun., 2018, 54, 2212–2215 RSC;
(e) K. Griffiths and G. E. Kostakis, Dalton Trans., 2018, 47, 12011–12034 RSC;
(f) N. V. T. S. M. Gorantla, P. G. Reddy, S. M. A. Shakoor, R. Mandal, S. Roy and K. C. Mondal, ChemistrySelect, 2019, 4, 7722–7727 CrossRef.
-
(a) S. Reinoso, Dalton Trans., 2011, 40, 6610–6615 RSC;
(b) J. W. Sharples and D. Collison, Coord. Chem. Rev., 2014, 260, 1–20 CrossRef CAS PubMed;
(c) K. Liu, W. Shi and P. Cheng, Coord. Chem. Rev., 2015, 289–290, 74–122 CrossRef CAS;
(d) M. Andruh, Chem. Commun., 2018, 54, 3559–3577 RSC;
(e) S.-J. Liu, S.-D. Han, J.-P. Zhao, J. Xu and X.-H. Bu, Coord. Chem. Rev., 2019, 394, 39–52 CrossRef CAS;
(f) J. Wang, M. Feng, M. N. Akhtar and M. L. Tong, Coord. Chem. Rev., 2019, 387, 129–153 CrossRef CAS;
(g) R. O. Fuller, G. A. Koutsantonis and M. I. Ogden, Coord. Chem. Rev., 2020, 402, 213066 CrossRef CAS.
-
(a) S. Goswami, A. K. Mondal and S. Konar, Inorg. Chem. Front., 2015, 2, 687–712 RSC;
(b) S. Xue, Y.-N. Guo, L. Zhao, H. Zhang and J. Tang, Inorg. Chem., 2014, 53, 8165–8171 CrossRef CAS PubMed;
(c) E. M. Pineda, N. F. Chilton, F. Tuna, R. E. P. Winpenny and E. J. L. McInnes, Inorg. Chem., 2015, 54, 5930–5941 CrossRef PubMed;
(d) M. Ferbinteanu, A. Stroppa, M. Scarrozza, I. Humelnicu, D. Maftei, B. Frecus and F. Cimpoesu, Inorg. Chem., 2017, 56, 9474–9485 CrossRef CAS PubMed.
-
(a) L. R. Piquer and E. C. Sañudo, Dalton Trans., 2015, 44, 8771–8780 RSC;
(b) A. Dey, J. Acharya and V. Chandrasekhar, Chem.–Asian J., 2019, 14, 4433–4453 CrossRef CAS PubMed;
(c) J. Sun, Z. Sun, K. Wang, L. Xi, Y. Ma and L. Li, J. Mater. Chem. C, 2019, 7, 9057–9064 RSC.
-
(a) M. Hołyńska, D. Premužić, I.-R. Jeon, W. Wernsdorfer, R. Clérac and S. Dehnen, Chem.–Eur. J., 2011, 17, 9605–9610 CrossRef PubMed;
(b) J. Li, R.-M. Wei, T.-C. Pu, F. Cao, L. Yang, Y. Han, Y.-Q. Zhang, J.-L. Zuo and Y. Song, Inorg. Chem. Front., 2017, 4, 114–122 RSC.
-
(a) Z.-Y. Li, C. Zhang, B. Zhai, J.-C. Han, M.-C. Pei, J.-J. Zhang, F.-L. Zhang, S.-Z. Li and G.-X. Cao, CrystEngComm, 2017, 19, 2702–2708 RSC;
(b) D. I. Alexandropoulos, L. Cunha-Silva, J. Tang and T. C. Stamatatos, Dalton Trans., 2018, 47, 11934–11941 RSC;
(c) S. Maity, A. Mondal, S. Konar and A. Ghosh, Dalton Trans., 2019, 48, 15170–15183 RSC.
-
(a) M.-J. Liu, K.-Q. Hu, C.-M. Liu, A.-L. Cui and H.-Z. Kou, New J. Chem., 2016, 40, 8643–8649 RSC;
(b) H.-S. Wang, F.-J. Yang, Q.-Q. Long, Z.-Y. Huang, W. Chen and Z.-Q. Pan, New J. Chem., 2017, 41, 5884–5892 RSC;
(c) Z.-X. Zhu, L.-Z. Cai, X.-W. Deng, Y.-L. Zhou and M.-X. Yao, New J. Chem., 2017, 41, 11097–11103 RSC;
(d) B. Dey, S. Roy, A. K. Mondal, A. Santra and S. Konar, Eur. J. Inorg. Chem., 2018, 2429–2436 CrossRef CAS;
(e) C.-M. Liu, D.-Q. Zhang, X. Hao and D.-B. Zhu, Inorg. Chem., 2018, 57, 6803–6806 CrossRef CAS PubMed.
-
(a) F.-H. Zhao, S.-H. Liang, S. Jing, Y. Wang, Y.-X. Che and J.-M. Zheng, Inorg. Chem. Commun., 2012, 21, 109–113 CrossRef CAS;
(b) J. Long, J. Rouquette, J.-M. Thibaud, R. A. S. Ferreira, L. D. Carlos, B. Donnadieu, V. Vieru, L. F. Chibotaru, L. Konczewicz, J. Haines, Y. Guari and J. Larionova, Angew. Chem., Int. Ed., 2015, 54, 2236–2240 CrossRef CAS PubMed;
(c) S. Chattopadhyay, S. Petit, E. Ressouche, S. Raymond, V. Balédent, G. Yahia, W. Peng, J. Robert, M.-B. Lepetit, M. Greenblatt and P. Foury-Leylekian, Sci. Rep., 2017, 7, 14506 CrossRef CAS PubMed.
-
(a) J. Liu, X.-P. Zhang, T. Wu, B.-B. Ma, T.-W. Wang, C.-H. Li, Y.-Z. Li and X.-Z. You, Inorg. Chem., 2012, 51, 8649–8651 CrossRef CAS PubMed;
(b) A. Yadav, P. Kulkarni, B. Praveenkumar, A. Steiner and R. Boomishankar, Chem.–Eur. J., 2018, 24, 14639–14643 CrossRef CAS PubMed;
(c) H. L. B. Boström, M. S. Senn and A. L. Goodwin, Nat. Commun., 2018, 9, 2380 CrossRef PubMed;
(d) J.-C. Liu, W.-Q. Liao, P.-F. Li, Y.-Y. Tang, X.-G. Chen, X.-J. Song, H.-Y. Zhang, Y. Zhang, Y.-M. You and R.-G. Xiong, Angew. Chem., Int. Ed., 2020 DOI:10.1002/anie.201914193.
-
(a) C.-M. Liu, R.-G. Xiong, D.-Q. Zhang and D.-B. Zhu, J. Am. Chem. Soc., 2010, 132, 4044–4045 CrossRef CAS PubMed;
(b) M. Ren, Z.-L. Xu, T.-T. Wang, S.-S. Bao, Z.-H. Zheng, Z.-C. Zhang and L.-M. Zheng, Dalton Trans., 2016, 45, 690–695 RSC;
(c) J. Mayans, M. Font-Bardia and A. Escuer, Inorg. Chem., 2018, 57, 926–929 CrossRef CAS PubMed;
(d) R. Akiyoshi, Y. Hirota, D. Kosumi, M. Tsutsumi, M. Nakamura, L. F. Lindoy and S. Hayami, Chem. Sci., 2019, 10, 5843–5848 RSC;
(e) M. Liu, H. Yu and Z. Liu, CrystEngComm, 2019, 21, 2355–2361 RSC.
-
(a) X.-K. Hou, L.-F. Wu, H.-P. Xiao, M.-X. Li, S.-R. Zhu and Z.-X. Wang, Z. Anorg. Allg. Chem., 2013, 639, 633–636 CrossRef CAS;
(b) Z. Zhou, X.-K. Hou, C.-C. Xue and Z.-X. Wang, Russ. J. Coord. Chem., 2015, 41, 240–245 CrossRef.
- Z.-X. Wang, L.-F. Wu, X.-K. Hou, M. Shao, H.-P. Xiao and M.-X. Li, Z. Anorg. Allg. Chem., 2014, 640, 229–235 CrossRef CAS.
-
(a) Z.-H. Yang, L.-X. Wang, Z.-H. Zhou, Q.-L. Zhou and C.-C. Tang, Tetrahedron: Asymmetry, 2001, 12, 1579–1582 CrossRef CAS;
(b) M. E. S. Serra, D. Murtinho, A. Goth, A. M. D. ’A. Rocha Gonsalves, P. E. Abreu and A. A. C. C. Pais, Chirality, 2010, 22, 425–431 CAS.
- A. Jana, S. Majumder, L. Carrella, M. Nayak, T. Weyhermueller, S. Dutta, D. Schollmeyer, E. Rentschler, R. Koner and S. Mohanta, Inorg. Chem., 2010, 49, 9012–9025 CrossRef CAS PubMed.
-
(a) S. R. Batten and K. S. Murray, Coord. Chem. Rev., 2003, 246, 103–130 CrossRef CAS;
(b) C.-F. Sheu, S. b. Pillet, Y.-C. Lin, S.-M. Chen, I. J. Hsu, C. Lecomte and Y. Wang, Inorg. Chem., 2008, 47, 10866–10874 CrossRef CAS PubMed;
(c) M. Wriedt and C. Näther, Dalton Trans., 2011, 40, 886–898 RSC.
-
(a) R. Koner, H.-H. Lin, H.-H. Wei and S. Mohanta, Inorg. Chem., 2005, 44, 3524–3536 CrossRef CAS PubMed;
(b) R. Koner, G.-H. Lee, Y. Wang, H.-H. Wei and S. Mohanta, Eur. J. Inorg. Chem., 2005, 1500–1505 CrossRef CAS;
(c) T. Ueno, T. Fujinami, N. Matsumoto, M. Furusawa, R. Irie, N. Re, T. Kanetomo, T. Ishida and Y. Sunatsuki, Inorg. Chem., 2017, 56, 1679–1695 CrossRef CAS PubMed.
-
(a) F. Z. C. Fellah, S. Boulefred, A. C. Fellah, B. El Rez, C. Duhayon and J.-P. Sutter, Inorg. Chim. Acta, 2016, 439, 24–29 CrossRef;
(b) V. Chandrasekhar, A. Dey, S. Das, M. Rouzières and R. Clérac, Inorg. Chem., 2013, 52, 2588–2598 CrossRef CAS PubMed.
-
(a) F. A. Mautner, J. H. Albering, M. Mikuriya and S. S. Massoud, Inorg. Chem. Commun., 2010, 13, 796–799 CrossRef CAS;
(b) P. Talukder, S. Shit, A. Sasmal, S. R. Batten, B. Moubaraki, K. S. Murray and S. Mitra, Polyhedron, 2011, 30, 1767–1773 CrossRef CAS.
- J. P. Costes, J. P. Laussac and F. Nicodeme, J. Chem. Soc., Dalton Trans., 2002, 2731–2736 RSC.
- Y. Fan, W. You, W. Huang, J.-L. Liu and Y.-N. Wang, Polyhedron, 2010, 29, 1149–1155 CrossRef CAS.
-
(a) J. Mayans, M. Font-Bardia, L. D. Bari, M. Górecki and A. Escuer, Chem.–Eur. J., 2018, 24, 18705–18717 CrossRef CAS PubMed;
(b) D. Zhang, J. Cano, W. Lan, H. Liu, F. Sun, Y. Dong, Z. Zhou, L. Yang, Q. Liu and J. Jiang, J. Mater. Chem. C, 2019, 7, 3623–3633 RSC;
(c) T. Bereta, A. Mondal, K. Ślepokura, Y. Peng, A. K. Powell and J. Lisowski, Inorg. Chem., 2019, 58, 4201–4213 CrossRef CAS PubMed.
- Y. Sui, D.-S. Liu, R.-H. Hua and J.-G. Huang, Inorg. Chim. Acta, 2013, 395, 225–229 CrossRef CAS.
- H.-R. Wen, J. Bao, S.-J. Liu, C.-M. Liu, C.-W. Zhang and Y.-Z. Tang, Dalton Trans., 2015, 44, 11191–11201 RSC.
-
(a) B. Bleaney and K. D. Bowers, Proc. R. Soc. London, Ser. A, 1952, 214, 451–465 CrossRef CAS;
(b) Y. Journaux, J. Sletten and O. Kahn, Inorg. Chem., 1985, 24, 4063–4069 CrossRef CAS;
(c) J. Pierre Costes, F. Dahan, A. Dupuis and J. P. Laurent, Inorg. Chem., 1996, 35, 2400–2402 CrossRef PubMed.
-
(a) X.-L. Li, C.-L. Chen, L.-F. Han, C.-M. Liu, Y. Song, X.-G. Yang and S.-M. Fang, Dalton Trans., 2013, 42, 5036–5041 RSC;
(b) X.-L. Li, M. Hu, Z. Yin, C. Zhu, C.-M. Liu, H.-P. Xiao and S. Fang, Chem. Commun., 2017, 53, 3998–4001 RSC;
(c) Y.-L. Liu, J.-Z. Ge, Z.-X. Wang and R.-G. Xiong, Inorg. Chem. Front., 2020, 7, 128–133 RSC.
-
(a) S. Bhattacharya, S. Pal and S. Natarajan, ChemPlusChem, 2016, 81, 733–742 CrossRef CAS PubMed;
(b) W.-J. Xu, P.-F. Li, Y.-Y. Tang, W.-X. Zhang, R.-G. Xiong and X.-M. Chen, J. Am. Chem. Soc., 2017, 139, 6369–6375 CrossRef CAS PubMed;
(c) B. Huang, L.-Y. Sun, S.-S. Wang, J.-Y. Zhang, C.-M. Ji, J.-H. Luo, W.-X. Zhang and X.-M. Chen, Chem. Commun., 2017, 53, 5764–5766 RSC;
(d) C. Xu, W.-Y. Zhang, Q. Ye and D.-W. Fu, Inorg. Chem., 2017, 56, 14477–14485 CrossRef CAS PubMed;
(e) K. Pasińska, A. Piecha-Bisiorek, V. Kinzhybalo, A. Ciżman, C. A. Gągora and A. Pietraszkoa, Dalton Trans., 2018, 47, 11308–11312 RSC.
Footnote |
† Electronic supplementary information (ESI) available: 2D layer and 3D supramolecular structures, X-ray crystallographic information files, and IR spectra are available for 1 and 2. CCDC 1973619 and 1973620 for 1 and 2. For ESI and crystallographic data in CIF or other electronic format see DOI: 10.1039/d0ra00732c |
|
This journal is © The Royal Society of Chemistry 2020 |
Click here to see how this site uses Cookies. View our privacy policy here.