DOI:
10.1039/D0RA01420F
(Paper)
RSC Adv., 2020,
10, 11046-11053
Two rare {M2(MoO4)2}n chain-containing molybdate-based metal–organic complexes with a bis-pyrazole-bis-amide ligand: fluorescent sensing and photocatalysis performance†
Received
14th February 2020
, Accepted 11th March 2020
First published on 17th March 2020
Abstract
By introducing a bis-pyrazole-bis-amide ligand, N,N′-bis(1H-pyrazole-4-carboxamide)-1,4-benzene (L), two molybdate-based metal–organic complexes containing {M2(MoO4)2}n (M = Co, Zn), [Co2L2(MoO4)2]·H2O (1), [Zn2L2(MoO4)2]·H2O (2), have been prepared under hydrothermal/solvothermal conditions. X-ray diffraction analyses reveal that both 1 and 2 are isostructural. An interesting structural feature is that a kind of {M2(MoO4)2}n chain could be found in 1 and 2, although different raw materials [Mo7O24]6− and [PMo12O40]3− anions were utilized. Then these chains are further linked by L ligands into a two dimensional (2D) structure. The title complexes represent the first examples containing {MoO4} units and pyrazole-/or amide-derivative ligands. Complexes 1 and 2 exhibit distinct performances due to different metal centers, with 2 acting as a fluorescent sensor for Fe3+, MnO4−, CrO42− and Cr2O72−, but 1 being a better photocatalyst towards degradation of cationic dyes methylene blue (MB) and neutral red (NR).
Introduction
Currently, as an important research branch of polyoxometalate (POM) chemistry, polyoxomolybdate-based metal–organic complexes (MOCs) have attracted numerous researchers' attention, not only due to their diverse structures caused by different amounts of molybdenum centers,1–7 but also their outstanding properties in the fields of electrochemistry, medicine, fluorescence, materials and photocatalysis, et al.8,9 During the synthesis of these MOCs, the polyoxomolybdates, including [MoO4]2−, [Mo3O10]2−, [Mo7O24]6−, [Mo8O26]4− and so on,10–13 can be usually produced by changing reaction conditions or raw materials. Among them, the {MoO4} unit-containing MOCs are of interest, which can be converted from different raw materials.14 However, reports on the {MoO4}-based MOCs are still very limited. In 1998, Zubieta's group synthesized a Cu-based complex [Cu(dpe)(MoO4)] (dpe = 1,2-trans-(4-pyridyl)ethane),15 where the [MoO4]2− unit was derived from a MoO3 raw material. The same Zubieta's group used NiII, di-4-pyridylamine (dpa) ligand and appropriate oxides (Et4NOH) to produce a NiII-organic diamine-EO42− (E = S or Mo) complex [Ni(dpa)2(MoO4)] through hydrothermal reaction in 1999.16 Using Na2MoO4·2H2O as a raw material, a complex [Cu(4,4′-bpy)]2[MoO4]·2H2O containing [MoO4]2− units was obtained by Huang's group.17 In complex [Zn2(H2O)L(MoO4)]n (L = 2,2′-bipyridine-6,6′-dicarboxylic acid) prepared by Niu's group in 2011, the [MoO4]2− unit came of Na2H[PMo12O40]·14H2O.18 In addition, some inorganic functional materials constructed with {MoO4} units and metals have also been reported, which have a wide range of applications.19,20 However, as mentioned above, most of the [MoO4]2−-containing MOCs are constructed from pyridyl derivated ligands, the use of pyrazole-/or amide-derivative ligands in the preparing molybdate-based MOCs has not been reported yet.
Here, we try to introduce bis-pyrazole-bis-amide ligand, N,N′-bis(1H-pyrazole-4-carboxamide)-1,4-benzene (L) (Scheme 1) into the reaction system containing [Mo7O24]6− and [PMo12O40]3− anions to synthesize POM-based cobalt(II) or zinc(II) complexes. Fortunately, two isomorphic MOCs [Co2L2(MoO4)2]·H2O (1) and [Zn2L2(MoO4)2]·H2O (2) were obtained under different reaction conditions, both of which consist of a kind of rare {M2(MoO4)2}n chain and L ligands. To the best of our knowledge, the title complexes represent the first examples of MOCs combining MoO42− units and pyrazole-/or amide-derivative ligands, especially using different molybdate-containing raw materials. The fluorescence and photocatalytic properties of complexes 1 and 2 were investigated further.
 |
| Scheme 1 The synthesis process of the ligand L in this work. | |
Experimental
Materials and methods
All chemicals and solvents were purchased from commercial sources, and used without further purification before the experiment. The synthesis method of ligand L was obtained according to previous literature.21 The FT-IR spectra were collected on a Varian 640 FT-IR spectrometer. Powder X-ray diffraction (PXRD) data were taken on a D/teX Ultra diffractometer by Cu Kα radiation. The collection of fluorescence experimental data was obtained by using Hitachi F-4500 fluorescence spectrometer. A diffuse reflectivity spectrum were collected with a spectrophotometer (Lambda, Model 750). The UV-vis absorption spectra was measured by a SP-1901 UV-vis spectrophotometer.
Synthesis of complexes 1–2
Synthesis of [Co2L2(MoO4)2]·H2O (1). A mixture of CoCl2·6H2O (0.048 g, 0.2 mmol), L (0.025 g, 0.1 mmol), (NH4)6Mo7O24·4H2O (0.124 g, 0.1 mmol) in a mixed-solvent of 6 mL water and 4 mL DMF (N,N-dimethylformamide) was transferred into a Teflon-lined autoclave and kept at 120 °C for 4 days. After slow cooling to room temperature, pink block crystals of 1 were obtained in a yield of 45% based on Co. Anal calcd for C28H26Co2Mo2N12O13 (%): C 32.08, H 2.50, N 13.03; found: C 32.01, H 2.42, N 12.98. IR (KBr pellet, cm−1): 3414 (s), 3294 (w), 3119 (w), 2354 (w), 1628 (s), 1540 (s), 1409 (w), 1340 (s), 1246 (m), 1183 (w), 1114 (m), 1064 (m), 1007 (m), 914 (m), 870 (m), 832 (w), 763 (m), 682 (m), 644 (w), 600 (w).
Synthesis of [Zn2L2(MoO4)2]·H2O (2). A mixture of ZnNO3·6H2O (0.060 g, 0.2 mmol), L (0.025 g, 0.1 mmol), H3PMo12O40·12H2O (0.183 g, 0.1 mmol) in a mixed-solvent of 6 mL water and 4 mL NaOH (0.1 mol L−1) was transferred into a Teflon-lined autoclave and kept at 120 °C for 4 days. After slow cooling to room temperature, yellow block crystals of 2 were obtained in a yield of 28% based on Zn. Anal calcd for C28H26Zn2Mo2N12O13 (%): C 31.69, H 2.47, N 15.84; found: C 31.62, H 2.41, N 15.78. IR (KBr pellet, cm−1): 3395 (w), 3289 (w), 3119 (w), 2361 (w), 1641 (s), 1540 (s), 1459 (w), 1409 (w), 1371 (w), 1340 (s), 1283 (w), 1252 (m), 1177 (w), 1114 (m), 1064 (w), 1007 (w), 870 (s), 776 (m), 694 (w), 650 (w), 600 (w).
X-ray crystallography
X-ray crystallographic data for complexes 1–2 were collected at 293 K on a Bruker SMART APEX II CCD diffractometer equipped with MoKα monochromatic radiation (λ = 0.71069 Å) by φ and ω scanning methods. The crystal structure of the title complexes was solved by direct method using SHELXTL-2014 program, and further refined on F2 by full matrix least square method.22 The crystallographic data and detailed parameters of complexes 1–2 reported in this paper are listed in Table 1. The selected bond length (Å) and angle (°) of complexes 1–2 are provided in Table S1.† The complexes 1–2 have been recorded at the Cambridge Crystallographic Data Center under the numbers 1972119 and 1972120.
Table 1 Crystallographic data for complexes 1–2
R1 = ∑|Fo| − |Fc|/∑|Fo|. wR2 = {∑[w(Fo2 − Fc2)2]/∑[w(Fo2)2]}1/2. |
Complex |
1 |
2 |
Empirical formula |
C28H26Co2Mo2N12O13 |
C28H26Zn2Mo2N12O13 |
Formula weight |
1048.35 |
1061.23 |
Temperature/K |
293(2) K |
293(2) K |
Crystal system |
Monoclinic |
Monoclinic |
Space group |
C2/c |
C2/c |
a/Å |
32.758(5) |
32.805(4) |
b/Å |
6.9308(10) |
7.0020(8) |
c/Å |
15.972(2) |
15.9094(19) |
α/° |
90 |
90 |
β/° |
113.128(5) |
112.537(4) |
γ/° |
90 |
90 |
V/Å3 |
3334.9(8) |
3375.3(7) |
Z |
4 |
4 |
Dc (g cm−3) |
2.088 |
2.088 |
μ/mm−1 |
1.801 |
2.218 |
F(000) |
2080 |
2104 |
Reflection collected |
10 970 |
11 936 |
Unique reflections |
3759 |
4165 |
Parameters |
258 |
258 |
Rint |
0.0648 |
0.0581 |
Goodness on F2 |
0.991 |
0.943 |
R1a [I > 2σ(I)] |
0.0434 |
0.0383 |
wR2b (all data) |
0.0957 |
0.0846 |
Result and discussion
Syntheses of complexes 1 and 2
In previous reports, most {MoO4}-based complexes were obtained from raw materials MoO3, Na2MoO4·2H2O etc.14,15 In this work, an interesting feature is that the raw materials [Mo7O24]6− and [PMo12O40]3− anions were transformed into {MoO4} units in final structures of complexes 1 and 2, which have rarely been observed in previous reports. During the synthesis, we also used other raw materials, such as Na2MoO4·2H2O to synthesize the title complexes, however, no single crystal complexes could be obtained. In addition, for complex 1, [PMo12O40]3− anions was also introduced into the synthesis system in place of [Mo7O24]6−, as a result, only some precipitates could be obtained and no crystals could be formed; for complex 2, when the ZnII and [Mo7O24]6− are used as raw materials, and no single crystal products could be synthesized. It can be concluded that the conversion of {MoO4} structural units requires specific reaction conditions and raw materials. That is to say, the metals and polyoxometalate, as well as solvent system, have a significant effect on the synthesis of the target products.
Crystal structure of 1 and 2
Single crystal X-ray diffraction shows that complexes 1 and 2 are isostructural, both of which are 2D networks and belong to the C2/c space group in the monoclinic system. Here, only the structure of complex 1 is described in details as a representative. As shown in Fig. 1a, the asymmetric unit of 1 contains one crystallographically independent CoII cation, one L ligand, one [MoO4]2− anion and half of water molecule. The valence sum calculations display that the Mo atoms are in the +VI oxidation state, and the Co atom are in the +II oxidation state.23 The CoII center is six-coordinated and displays a twisted octahedral coordination geometry, surrounded by two pyrazole N atoms (N1 and N2) from two L ligands and one O atom (O1) from the amide group in the ligand, three terminal O atoms (O3, O4 and O5) belonging to three [MoO4]2− anions, respectively. The bond lengths of Co–N and Co–O are in the range of 2.111(4) to 2.128(4) Å and 2.054(4) to 2.184(3) Å, respectively.
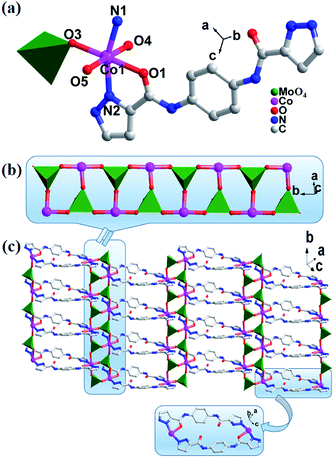 |
| Fig. 1 (a) Ball/stick/polyhedron view of the asymmetric unit of 1. The hydrogen atoms are omitted for clarity; (b) the view of polyoxometalate chain {Co/MoO4}n; (c) the 2D network structure of 1. | |
In complex 1, each of Mo atoms is a tetrahedral configuration, surrounded by four oxygen atoms, and further aggregated by the six-coordinated Co atoms via sharing three oxygen atoms (O3, O4, O5) to give a {Co2(MoO4)2}n chain (Fig. 1b). These {Co2(MoO4)2}n chains are extended by L ligands into a 2D layer (Fig. 1c). On one hand, the L ligands acting as bridging linkers are arranged in pair and in an inverted mode. On the other hand, each of L ligands shows a tridentate coordination mode, namely, both N1 atom of pyrazolyl and O1 atom of amide at one end of L ligand chelate together one CoII ion, but only one N2 atom of pyrazolyl at another end of L ligand coordinates with one CoII center. In fact, each CoII ion is surrounded with such two kinds of L ligands. Complex 2 also has a 2D structure, which is similar to that of complex 1, and the corresponding structure is shown in Fig. S1.†
PXRD and IR
As shown in Fig. S2,† the PXRD results of the complexes 1–2 at room temperature basically coincide with the simulated diffraction peaks of the complexes 1–2, and the results show that the phase purity and stability of the complexes are good. The slight difference in intensity between the diffraction peaks may be due to the orientation of the crystallites in the powder sample tested.24
The IR test results of complexes 1–2 are shown in Fig. S3.† Because complexes 1 and 2 are isomorphic complexes, their infrared peak positions are generally the same, except that the peak strength is slightly different. The characteristic absorption peaks of 1064 cm−1 for 1 and 2 are attributed to the ν(Mo
Ot), but the peak intensities are different. And the ν(M–O–Mo) (M = Co or Zn) vibration peak in complexes 1–2 is at 870 cm−1.18 Bands at 1628 cm−1 for 1, 1641 cm−1 for 2, can be attributed to the ν(C
O), respectively. The ν(N–H) vibrations peak in complexes 1–2 is at 1409 cm−1.25 In addition, the characteristic bands from –OH groups of water molecules can be observed in the vicinity of 3414 cm−1 for 1, 3395 cm−1 for 2.26
Fluorescence properties
Generally, polyoxomolybdate-based MOCs based on ZnII have shown good properties and applications as fluorescent materials.27–29 Therefore, the solid-state fluorescent properties of complexes 1–2, were also investigated at room temperature, as well as L, H3PMo12O40·12H2O and (NH4)6Mo7O24·4H2O. As shown in Fig. 2a, the free L ligand shows strong emission band at 401 nm (λex = 300 nm), such transitions are π* → π in nature.30 As we expected, only the emission spectrum of complex 2 based on ZnII ion is observed at approximately 428 nm (λex = 300 nm), and the similarity to the free L ligand may be owing to the intraligand transition of the ligands.31,32 However, complex 1, H3PMo12O40·12H2O and (NH4)6Mo7O24·4H2O have no obvious emission peaks, compared to that of complex 2.
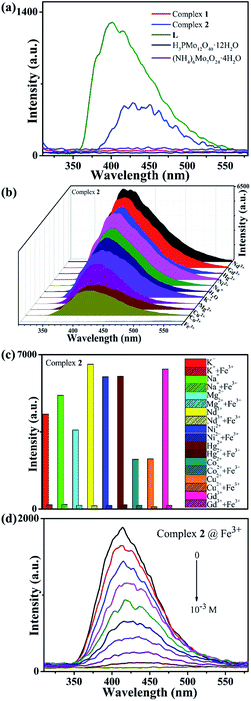 |
| Fig. 2 (a) Fluorescence spectra of complexes 1–2, L, H3PMo12O40·12H2O and (NH4)6Mo7O24·4H2O at solid state; (b) fluorescence spectra of Mn+ @ 2; (c) fluorescence emission intensities of 2 at room temperature upon the addition of Fe3+ or Fe3+ + Mn+ ions; (d) emission spectra of the suspension of 2 with the Fe3+ aqueous concentration from 0 to 10−3 M. | |
In general, hydrothermally or solvothermally synthesized complexes have good stability. In this work, complex 2 is insoluble in water and other salt solutions. Therefore, its abilities to detect metal cations in aqueous solution were explored. As shown in Fig. 2b, the fluorescence response experiments of complex 2 containing different metal cations with 10−3 M (Na+, K+, Hg2+, Ni2+, Mg2+, Cu2+, Co2+, Gd3+, Nd3+ and Fe3+) were performed.33 It can be observed clearly that the fluorescence intensity of the suspension of complex 2 containing only Fe3+ ions is significantly quenched. In comparison, other metal cations have no significant effect on the emission intensity. The results indicate that complex 2 may have a good ability to fluorescence detect Fe3+ ion in aqueous solutions. Thus, the anti-interference experiments were also studied. Fig. 2c shows that the fluorescence quenching activities of suspensions in the presence of other cations can be still observed with the addition of Fe3+ ions, confirms that complex 2 has a high selectivity for detecting Fe3+ ions.
In order to further explore the sensitivity of complex 2 to detect Fe3+ ions, the quantitative fluorescence titration experiment was performed. As shown in Fig. 2d, with the quantitative addition of Fe3+ ions (concentration changed from 0 to 10−3 M), the emission intensity of the suspension of complex 2 are weakened gradually, and the fluorescence quenching efficiency reaches to as high as 99.1% (Fig. S4†). Based on the good linear relationship at lower concentrations between the fluorescence intensity and the concentration of Fe3+ ions, the Stern–Volmer equation (I0/I = 1 + KSV[C]) is used to describe the fluorescence quenching effect,34 where KSV is the quenching constant (M−1), [C] is Fe3+ ions concentration, I0 and I are the emission intensities before and after Fe3+ ions were added. As shown in Fig. S5,† the KSV value of Fe3+ is calculated as 2.53 × 104 M−1. The results indicate that complex 2 has excellent sensitivity to detect Fe3+ ions in aqueous solution.
Further, different anions including Br−, NO2−, Cl−, OH−, I−, Ac−, MnO4−, CrO42−, Cr2O72−, were selected to explore the fluorescence detection abilities for anions of complex 2. The experiment method was similar to that of cation involved. As shown in Fig. 3a, when the MnO4−, CrO42− or Cr2O72− anions are added, the emission peak of the suspension containing complex 2 undergoes significant quenching behaviours, while the fluorescence intensities have only slight changes for other anions. When the MnO4− (CrO42− and Cr2O72−) anion is added to suspension of complex 2 containing other anions, the fluorescence intensity of the suspension can still be effectively quenched, indicating that complex 2 has high selectivity for fluorescence detection MnO4− (CrO42− and Cr2O72−) anion (Fig. S6†). In addition, we quantitatively added MnO4− (CrO42− and Cr2O72−) anion aqueous solution to the suspension of complex 2 at a concentration from 0 to 10−3 M. As shown in Fig. 3b–d, as the MnO4− (CrO42− and Cr2O72−) anion is quantitatively increased, the fluorescence intensity of the mixture suspension gradually decreases until it is completely quenched, which proves that complex 2 has good sensitivity for fluorescence detection of MnO4− (CrO42− and Cr2O72−) anion aqueous solution. The KSV values of 1.97 × 104 M−1 (MnO4−) and 2.31 × 104 M−1 (CrO42−) show strong quenching effects on these anions for complex 2, while the fluorescence intensity has an exponential relationship with the concentration of Cr2O72− anion at lower concentrations (Fig. S5†). Meanwhile, the fluorescence quenching efficiency is as high as 99.3% for MnO4−, 99.2% for CrO42−, 99.4% for Cr2O72−, respectively (Fig. S4†).
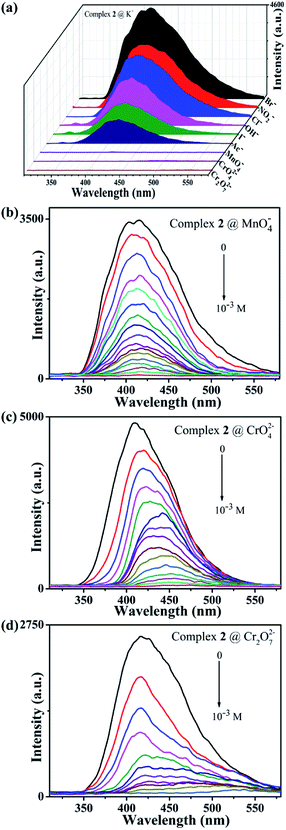 |
| Fig. 3 (a) Fluorescence spectra of 2 immersed into aqueous solution containing various anions; (b) fluorescence spectra of quantitatively titrating the MnO42−, (c) CrO42− and (d) Cr2O72− anion aqueous solution into the suspension of complex 2 (concentration range is from 0 to 10−3 M). | |
Furthermore, the quenching mechanism was explored to understand the quenching effect of complex 2 on Fe3+, MnO4−, CrO42− and Cr2O72− ions. Firstly, after being immersed in these ions aqueous solution for 24 hours, both IR and PXRD spectra of the sample confirm that the structure of complex 2 can be remained well in the process of fluorescence experiment (Fig. S2 and S3†). Secondly, complex 2 exhibits excellent fluorescence sensing behaviors towards Fe3+, MnO4−, CrO42− and Cr2O72− ions, and the quenching mechanism may be similar to those previous reports,35,36 which was further confirmed by UV-vis spectra (Fig. S7†). Namely, both complexes and Fe3+, MnO4−, CrO42−, Cr2O72− ions have overlap absorption bands in the 300–700 nm, which gives rise to the competition for excitation light absorption between the complexes and Fe3+, MnO4−, CrO42−, Cr2O72− ions,37 finally leading to the fluorescence quenching performance, which are similar to these previous reports.38,39
Optical band gaps and photocatalytic activity
As we know, many Co-based polyoxomolybdate-based metal organic framework exhibit usually good photocatalytic activities for the degradation of organic dyes under UV irradiation.40–42 Therefore, in order to evaluate the semiconductor behaviors and photocatalytic activities of Co-based complex 1, its diffuse reflection spectrum were measured in crystalline state at room temperature, as shown in Fig. 4. The band gap (Eg) of complex 1 is obtained by Kubelka–Munk (K–M) function,43 where complex 1 is estimated at 2.00 eV. The results indicate that complex 1 can be used as potential semiconductor materials for photocatalytic reactions.44
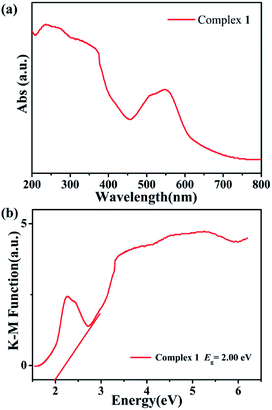 |
| Fig. 4 (a) Diffuse-reflectance spectra (Abs) of complex 1. (b) Diffuse reflection spectra of Kubelka–Munk (K–M) function versus energy (eV) of complex 1. | |
The photocatalytic degradation activities of complex 1 for cationic dyes methylene blue (MB), neutral red (NR) and anionic dyes malachite green (MG), methyl orange (MO) were investigated here. The detail experimental operations were as follows: 50 mg of complex 1 was dispersed in 90 mL of a 0.02 mM aqueous solution containing different dyes. The mixtures were then stirred in the dark for about 30 minutes to ensure the adsorption–desorption equilibrium of the working solution. Next, the mixed solutions were continuously stirred under UV radiation, during which 4 mL samples were taken every 30 minutes for MG and MO, 5 minutes for MB and NR, and analyzed by SP-1901 UV-vis spectrophotometer.45
As can be clearly shown in Fig. 5a and b, the absorption peaks of the cationic dyes (MB and NR) gradually decrease with the increase of UV irradiation time in the presence of complex 1 used as photocatalyst. After 30 minutes, the degradation rate reaches to as high as 93.6% for MB, 89.0% for NR, respectively. In contrast, no significant degradation towards anionic dyes MG and MO are observed at the same time, even after exposed to UV light for 150 minutes (Fig. 5c and d). Such catalytic efficiency is better than those in the previously reported literature. For example, Peter's group reported two new isostructural polyoxomolybdate-based metal organic complexes whose photocatalytic degradation efficiency for MB was 57% and 83%, respectively.46
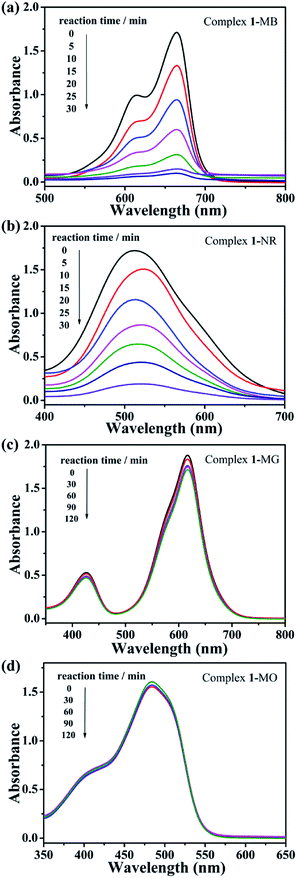 |
| Fig. 5 (a) Absorption spectrum of MB, (b) NR, (c) MG and (d) MO solution during photocatalytic degradation reaction under UV radiation in the presence of complex 1. | |
The possible mechanism of the photocatalytic activity was derived as follows: POM was induced to shift from the highest occupied molecular orbital (HOMO) to the lowest unoccupied molecular orbital (LUMO) under UV irradiation. The excited state of POM* was generated during this period, accompanied by the formation of H+, ·OH, and ·O2−, which can inactivate the organic dye during the oxidation process, thereby realizing the photocatalytic process.47,48 In order to certificate the above mechanism, we introduced benzoquinone (BQ) as the quencher of ·O2−, isopropyl alcohol (IPA) as the quencher of ·OH, and triethanolamine (TEOA) as the quencher of H+ to the photocatalytic MB and NR system. For the MB degradation reaction system, when IPA and TEOA were added separately, the photocatalytic degradation rate of complex 1 did not decrease significantly, and the presence of ·OH and H+ was suppressed after the quencher being added, which indicates that ·OH and H+ play a key role in the photocatalytic degradation of MB. After the addition of BQ, complex 1 can still effectively degrade MB, indicating that ·O2− does not affect MB degradation (Fig. 6). As shown in Fig. S8,† in the NR degradation reaction system, the addition of BQ and IPA, respectively, did not reduced significantly the photocatalytic degradation rate of complex 1, which indicates that ·O2− and ·OH do appear and play a key role in oxidizing NR.
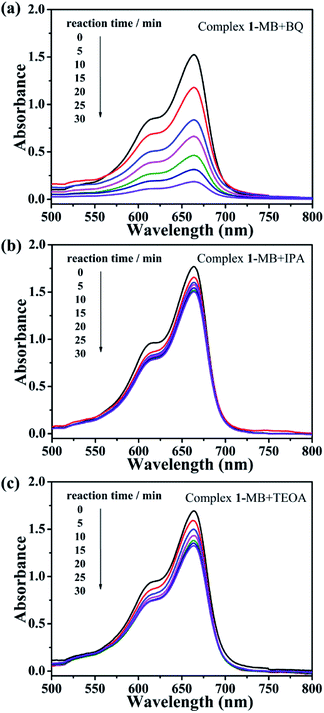 |
| Fig. 6 Trapping experiments of active species (a) BQ, (b) IPA and (c) TEOA during the photocatalytic degradation MB reaction for complex 1. | |
In addition, the stability of complex 1 during photocatalytic dye degradation was further measured. As shown in Fig. S2,† the PXRD spectra of both the experimental diffraction peaks of complex 1 after being used as photocatalyst and the simulated one are consistent, indicating that complex 1 is stable in photocatalytic experiments.49 That is to say, complex 1 has excellent photocatalytic effect for the degradation of cationic dyes MB and NR under UV light, which may be good photocatalyst for degradation of the pollutants containing MB and NR.
Conclusions
In summary, we successfully synthesized two molybdate-based MOCs with bis-pyrazole-bis-amide ligand as organic linkers. A kind of rare {M2(MoO4)2}n (M = Co or Zn) chain in 1 and 2 were constructed from different raw materials. Two complexes have distinct performances because of different metal centers, 1 can be selected as fluorescent sensors for detecting Fe3+, MnO4−, CrO42− and Cr2O72− ion, while 2 was better photocatalyst for degradation of cationic dyes MB and NR, which may be significant to exploit molybdate-based complexes with designated performance in the future.
Conflicts of interest
There are no conflicts to declare.
Acknowledgements
This work was financially supported by the National Natural Science Foundation of China (No. 21971024, 21671025 and 21771025) and supported by Liao Ning Revitalization Talents Program (XLYC1902011).
Notes and references
- P. J. Zapf, D. Hagrman and J. Zubieta, Angew. Chem., Int. Ed., 1999, 38, 2638 CrossRef.
- Q. F. Yang, J. Wang, X. Y. Chen, W. X. Yang, H. N. Pei, N. Hu, Z. H. Li, Y. R. Suo, T. Li and J. L. Wang, J. Mater. Chem. A, 2018, 6, 2184 RSC.
- L. Kan, J. Cai, Z. W. Jin, G. H. Li, Y. L. Liu and L. R. Xu, Inorg. Chem., 2019, 58, 391 CrossRef CAS PubMed.
- M. I. Khan, Q. Chen and J. Zubieta, Inorg. Chim. Acta, 1993, 213, 325 CrossRef CAS.
- V. Damjanović, J. Pisk, D. Kuzman, D. Agustin, V. Vrdoljak, V. Stilinović and M. Cindrić, Dalton Trans., 2019, 48, 9974 RSC.
- Y. R. Liu, J. X. Gou, X. Li, B. Dong, G. Q. Han, W. H. Hu, X. Shang, Y. M. Chai, Y. Q. Liu and C. G. Liu, Electrochim. Acta, 2016, 216, 397 CrossRef CAS.
- L. Buzzetti, G. E. M. Crisenza and P. Melchiorre, Angew. Chem., Int. Ed., 2019, 58, 3730 CrossRef CAS PubMed.
- Y. Wang, X. P. Kong, W. Xu, F. R. Jiang, B. Li and L. X. Wu, Inorg. Chem., 2018, 57, 3731 CrossRef CAS PubMed.
- K. B. Wang, Z. K. Wang, S. E. Wang, Y. Chu, R. Xi, X. Y. Zhang and H. Wu, Chem. Eng. J., 2019, 367, 239 CrossRef CAS.
- D. Hagrman, C. J. Warren, R. C. Haushalter, C. Seip, C. J. O'Connor, R. S. Rarig, K. M. Johnson, R. L. LaDuca and J. Zubieta, Chem. Mater., 1998, 10, 3294 CrossRef CAS.
- A. X. Tian, Y. B. Fu, H. T. Cui, J. Ying, M. L. Yang, Y. Yang and X. L. Wang, New J. Chem., 2019, 43, 9980 RSC.
- D. Hagrman and J. Zubieta, Chem. Commun., 1998, 2005–2006 RSC.
- D. J. Allis, R. S. Rarig, E. Burkholder and J. Zubieta, J. Mol. Struct., 2004, 688, 11 CrossRef CAS.
- R. S. Rarig, R. Lam, P. Y. Zavalij, J. K. Ngala, R. L. LaDuca, J. E. Greedan and J. Zubieta, Inorg. Chem., 2002, 41, 2124 CrossRef CAS PubMed.
- D. Hagrman, R. C. Haushalter and J. Zubieta, Chem. Mater., 1998, 10, 361 CrossRef CAS.
- C. Matthew Laskoski, L. Robert LaDuca, Jr., S. Randy Rarig, Jr. and J. Zubieta, J. Chem. Soc., Dalton Trans., 1999, 3467 RSC.
- C. Z. Lu, C. D. Wu, H. H. Zhuang and J. S. Huang, Chem. Mater., 2002, 14, 2649 CrossRef CAS.
- Y. Y. Guo, P. T. Ma, J. P. Wang and J. Y. Niu, J. Solid State Chem., 2011, 184, 3121 CrossRef CAS.
- V. V. Atuchin, A. S. Aleksandrovsky, B. G. Bazarov, J. G. Bazarova, O. D. Chimitova, Y. G. Denisenko, T. A. Gavrilova, A. S. Krylov, E. A. Maximovskiy, M. S. Molokeev, A. S. Oreshonkov, A. M. Pugachev and N. V. Surovtsev, J. Alloys Compd., 2019, 785, 692 CrossRef CAS.
- Y. B. Hua, S. K. Hussain and J. S. Yu, New J. Chem., 2019, 43, 10645 RSC.
- M. Sarkar and K. Biradha, Cryst. Growth Des., 2006, 6, 202 CrossRef CAS.
- G. M. Sheldrick, Acta Crystallogr., Sect. A: Found. Crystallogr., 2008, 64, 112 CrossRef CAS PubMed.
- I. D. Brown and D. Altermatt, Acta Crystallogr., Sect. B: Struct. Sci., 1985, 41, 244 CrossRef.
- S. Nam, A. D. French, B. D. Condon and M. Concha, Carbohydr. Polym., 2016, 135, 1 CrossRef CAS PubMed.
- S. Z. Ashraf, M. F. Erben and J. Simpson, J. Mol. Struct., 2017, 1129, 283 CrossRef.
- J. Sárkány, Appl. Catal., A, 1999, 188, 369 CrossRef.
- Y. Shen, J. Peng, C. Y. Chen, H. Q. Zhang, C. L. Meng and X. L. Li, Inorg. Chem. Commun., 2011, 14, 221 CrossRef CAS.
- X. L. Wang, J. J. Sun, H. Y. Lin, Z. H. Chang, A. X. Tian, G. C. Liu and X. Wang, Dalton Trans., 2016, 45, 2709 RSC.
- Y. Q. Lan, S. L. Li, X. L. Wang, K. Z. Shao, Z. M. Su and E. B. Wang, Inorg. Chem., 2008, 47, 529 CrossRef CAS PubMed.
- X. L. Wang, Y. Xiong, G. C. Liu, H. Y. Lin and X. Wang, Dalton Trans., 2018, 47, 9903 RSC.
- L. N. Wang, Y. H. Zhang, S. Jiang and Z. Z. Liu, CrystEngComm, 2019, 21, 4557 RSC.
- G. C. Liu, Y. Li, J. Chi, N. Xu, X. L. Wang, H. Y. Lin and Y. Q. Chen, Dyes Pigm., 2020, 174, 108064 CrossRef.
- C. B. Yang, C. B. Jiang, M. Y. Zhang, X. Chen, P. Zou, R. W. Yang, H. B. Rao and G. T. Wang, Polyhedron, 2020, 175, 114216 CrossRef CAS.
- Y. Q. Chen, Y. Tian, S. L. Yao, J. Zhang, R. Y. Feng, Y. J. Bian and S. J. Liu, Chem.–Asian J., 2019, 14, 4420 CrossRef CAS PubMed.
- L. Wang, W. Xu, W. Y. Li, M. Xie and Y. Q. Zheng, Chem.–Asian J., 2019, 14, 4246 CrossRef CAS PubMed.
- Q. Q. Xiao, G. Y. Dong, Y. H. Li and G. H. Cui, Inorg. Chem., 2019, 58, 15696 CrossRef CAS PubMed.
- X. S. Li, J. D. An, H. M. Zhang, J. J. Liu, Y. Li, G. X. Du, X. X. Wu, L. Fei, J. D. Lacoste, Z. Cai, Y. Y. Liu, J. Z. Huo and B. Ding, Dyes Pigm., 2019, 170, 107631 CrossRef CAS.
- L. Wang, T. T. Guo, J. C. Ma, Y. Y. Liu, G. H. Xu and J. F. Ma, ChemistrySelect, 2019, 4, 7351 CrossRef CAS.
- W. Q. Kan and S. Z. Wen, Dyes Pigm., 2017, 139, 372 CrossRef CAS.
- A. X. Tian, J. N. Liu, X. B. Ji, G. Y. Liu, T. T. Li, Y. Tian, H. P. Ni, G. C. Liu and J. Ying, J. Mol. Struct., 2018, 1155, 371 CrossRef CAS.
- H. Ramezanalizadeh and F. Manteghi, J. Photochem. Photobiol., A, 2017, 346, 89 CrossRef CAS.
- X. Pan, X. L. Wang, X. Wang, Y. Li, G. C. Liu and H. Y. Lin, CrystEngComm, 2019, 21, 6472 RSC.
- H. J. Du, C. H. Wang, Y. Li, Y. Y. Niu and H. W. Hou, RSC Adv., 2015, 5, 74065 RSC.
- H. Y. Liu, L. Bo, J. Yang, Y. Y. Liu, J. F. Ma and H. Wu, Dalton Trans., 2011, 40, 9782 RSC.
- X. L. Wang, R. Zhang, X. Wang, H. Y. Lin, G. C. Liu and H. X. Zhang, Dalton Trans., 2017, 46, 1965 RSC.
- S. Roy, V. Vemuri, S. Maiti, K. S. Manoj, U. Subbarao and S. C. Peter, Inorg. Chem., 2018, 57, 12078 CrossRef CAS PubMed.
- X. X. Zhao, S. W. Zhang, J. Q. Yan, L. D. Li, G. J. Wu, W. Shi, G. M. Yang, N. J. Guan and P. Cheng, Inorg. Chem., 2018, 57, 5030 CrossRef CAS PubMed.
- S. M. Wang, D. L. Li, C. Sun, S. G. Yang, Y. Guan and H. He, Appl. Catal., B, 2014, 144, 885 CrossRef CAS.
- X. L. Wang, R. Zhang, X. Wang, H. Y. Lin and G. C. Liu, Inorg. Chem., 2016, 55, 6384 CrossRef CAS PubMed.
Footnotes |
† Electronic supplementary information (ESI) available: IR, PXRD and additional figures. CCDC supplementary crystallographic data for the two molybdate-based metal–organic complexes synthesized in this article, numbered 1972119 and 1972120. For ESI and crystallographic data in CIF or other electronic format see DOI: 10.1039/d0ra01420f |
‡ These authors contributed equally to this work. |
|
This journal is © The Royal Society of Chemistry 2020 |
Click here to see how this site uses Cookies. View our privacy policy here.