DOI:
10.1039/D0RA02478C
(Paper)
RSC Adv., 2020,
10, 14451-14457
Inorganic/organic nanocomposite ion gels with well dispersed secondary silica nanoparticles†
Received
17th March 2020
, Accepted 27th March 2020
First published on 9th April 2020
Abstract
We have previously reported tough inorganic/organic nanocomposite (NC) ion gels composed of silica particles and poly(N,N-dimethylacrylamide) (PDMAAm) networks and a large amount of ionic liquid. In this study, the network structure and toughening mechanism of NC ion gels were investigated. The NC ion gels showed characteristic mechanical properties; i.e. the stress was significantly increased at a highly elongated state. In addition, the NC ion gels showed an almost elastic mechanical property, which was completely different from that of our other developed inorganic/organic tough ion gels named double-network (DN) ion gels. It was found from structural observation that secondary silica nanoparticles dispersed well in the NC ion gel. It was also found that some of the secondary silica nanoparticles had a ring-like structure which would incorporate PDMAAm chains. From the silica particle content dependency on stress–strain curves of inorganic/organic NC ion gels, it was inferred that the secondary silica particles could serve as a movable cross-linker of PDMAAm chains in the NC ion gel.
Introduction
Ion gels are gels containing ionic liquids (ILs) that exhibit gel-based quasi-solid properties in addition to IL-based properties, such as nonvolatility, nonflammability, high ionic conductivity, high CO2 solubility, and high thermal, chemical, and electrochemical stabilities. Ion gels are expected to be applied in electrochemical devices, actuators, and gas separation membranes.1–14 However, the practical applications of ion gels are limited because of their low mechanical strength. Therefore, the development of tough ion gels has attracted increasing interest.
Several types of tough ion gels have been prepared using different concepts, such as tetra-polyethylene glycol (PEG) network-based ion gels,15 triblock copolymer-based ion gels,16 and organic/organic double network (DN)-based ion gels.13,17–20 These tough ion gels showed not only excellent mechanical strength but also the unique IL properties.
Recently, we reported two types of ion gels prepared via one-pot/two-step network formation of inorganic/organic network, which were composed of inorganic silica particle aggregates and poly(N,N-dimethylacrylamide) (PDMAAm) organic networks.21 When the inorganic aggregates were formed before the organic network formation, the DN ion gels having interpenetrating inorganic/organic network were formed. Meanwhile, inorganic/organic nanocomposite (NC) ion gels were formed when the inorganic aggregates formed after the organic network formation. The mechanical properties of these ion gels differed vastly from each other, even though both gels indicated superior mechanical strength compared with a PDMAAm single-network (SN) ion gel. The inorganic/organic DN ion gels showed clear mechanical hysteresis, while the inorganic/organic NC ion gels showed an elastic behavior. Despite the unique mechanical behaviors, such as excellent mechanical strength and elastic behavior, the network structure and toughening mechanism of the inorganic/organic NC ion gel remained unknown.
The inorganic/organic NC ion gels showed specific stress–strain curves of which stress significantly increased when the gel was highly elongated.21 These specific mechanical behaviors are similar to those of slide ring (SR) hydrogels reported by Ito et al.22–27 The SR gels are composed of a polyrotaxane network, which is composed of poly(ethylene oxide) as an axis polymer and α-cyclodextrin as ring molecules. In the SR gels, the ring molecules can serve as a movable cross-linker for the axis polymer when the ring molecules are chemically cross-linked. Owing to the movable cross-link function by ring molecules, the SR gels showed specific J-shaped stress–strain curves and a nonhysteresis loop.27 We speculated that the characteristic mechanical properties and the toughening mechanism of the inorganic/organic NC ion gels were attributed to the movable cross-linker because the stress–strain behavior of the inorganic/organic NC ion gels was similar to that of the SR gels. If the movable cross-link phenomenon occurred in the inorganic/organic NC ion gels, silica particles in the ion gels should behave as the ring by incorporating PDMAAm chains in the particles to serve as a movable cross-linker for the PDMAAm network.
To demonstrate the hypothesis above, in this study, the network structure and mechanical properties of the inorganic/organic NC gel were investigated. The mechanical properties of the inorganic/organic NC ion gels with various silica particle contents were measured using a uniaxial stretch test and compared with that of SR hydrogel. The structure of the silica particles and their dispersion state in the inorganic/organic NC ion gels were observed using transmission electron microscopy (TEM) to confirm the structure of the silica nanoparticles formed in the NC ion gels. Based on the results of mechanical properties and TEM observation, the hypothesis on the toughening mechanism of the inorganic/organic NC ion gel is discussed.
Experimental section
Materials
As an IL, 1-butyl-3-methylimidazolium bis(trifluoro-methylsulfonyl)imide ([C4mim][Tf2N]) (Sigma-Aldrich Co., St. Louis, MO, U.S.A.) was used after eliminating the dissolved water by bubbling dry nitrogen through the IL for more than 15 min. N,N-dimethylacrylamide (DMAAm, Tokyo Chemical Industry Co., Tokyo, Japan) as a monomer of PDMAAm, 2-oxoglutaric acid (OA, Tokyo Chemical Industry Co.) as a photo radical initiator, and N,N′-methylenebis(acrylamide) (MBAA, FUJIFILM Wako Pure Chemicals Corporation, Osaka, Japan) as a chemical cross-linker of PDMAAm were used to form an organic PDMAAm network in the IL. DMAAm and OA were used as received. MBAA was used after purification by recrystallization in ethanol. Tetraethyl orthosilicate (TEOS, Sigma-Aldrich Co.) was used to form the silica particle without further purification. Formic acid (FUJIFILM Wako Pure Chemicals Corporation) was used as a solvolytic agent for the sol–gel reaction of TEOS without further purification.
Preparation of inorganic/organic NC ion gels and PDMAAm SN ion gel
Inorganic/organic NC ion gels were prepared via a one-pot/two-step process, inducing a photic-initiated free-radical polymerization of DMAAm first, followed by a thermally initiated sol–gel reaction of TEOS in the IL.21 For example, a precursor solution was prepared by mixing 7.92 g of [C4mim][Tf2N], 1.10 g of TEOS, 1.57 g of DMAAm (molar ratio of TEOS/DMAAm = 1/3 mol mol−1), 9.8 mg of MBAA (0.4% of DMAAm in mole), and 2.3 mg of OA (0.1% of DMAAm in mole) until the solution became completely transparent. Subsequently, 1.90 g of formic acid was gently added to the precursor solution and stirred until it was completely dissolved. The solution was injected into a mold comprising two glass plates with a fluorinated ethylene propylene copolymer film and a poly(tetrafluoroethylene) spacer (1.0 mm thickness) and then irradiated by a 365 nm ultraviolet light in an incubator at 293 K for 9 h to form a PDMAAm network. Subsequently, the ion gel was placed in a thermostat oven at 323 K for 48 h for silica particle formation. The obtained ion gel was maintained at 373 K for 12 h under vacuum to remove the formic acid, unreacted monomer, and generated ethanol through the sol–gel reaction of TEOS. To evaluate the effect of silica particle content on the mechanical property of the inorganic/organic NC ion gels, the silica particle content was controlled from 1.1 wt% to 5.5 wt%. The content of PDMAAm in the inorganic/organic NC ion gel was fixed at 17 wt% for every sample by controlling the [C4mim][Tf2N] content.
For comparison, the inorganic/organic DN ion gels were prepared by swapping the formation order of the PDMAAm network and silica particle from the same precursor solution of the inorganic/organic NC ion gels, i.e. the thermally initiated sol–gel reaction of TEOS was performed first, followed by the photic-initiated free-radical polymerization of DMAAm. Additionally, a PDMAAm SN ion gel was prepared in the same method without using the chemicals for the sol–gel reaction of TEOS. In the DN and SN gels, the content of PDMAAm was fixed at 17 wt%, which was the same as that of NC gel, by controlling the content of [C4mim][Tf2N].
Mechanical property measurement
A uniaxial stretch test of inorganic/organic NC ion gels was performed using an automatic recording universal testing instrument (EZ-LX, Shimadzu Co., Kyoto, Japan) at 298 K. A dumbbell-shaped specimen (length, width, thickness: 75.0, 4.0, 1.0 mm) was used for the uniaxial stretching test. Because the inorganic/organic NC ion gels contained no volatile components, the mechanical properties could be measured in an open environment without considering any composition change during the measurement. For all measurements, the sample was attached to the instrument at a distance of 35 mm between the jigs. A uniaxial stretching test was conducted by stretching the sample at a constant strain rate of 100 mm min−1. In the cyclic loading–unloading test, stretching and return operations were performed to increase the maximum strain in steps of 0.5 until the sample broke.
TEM observation
The silica particle in the inorganic/organic NC ion gels was observed using field-emission transmission electron microscopy (FE-TEM) (JEM-2100F, JEOL Ltd., Tokyo, Japan). The IL in the inorganic/organic NC ion gels was replaced with epoxy resin to prepare ultrathin sections. A 1 mm cubic sample of the ion gel was immersed in a sufficient volume of ethanol for 12 h to swap the IL in the ion gels for ethanol. The sample was immersed in a solution of epoxy resin (Plain Resin Kit, Nisshin EM Co., Ltd., Tokyo, Japan)/ethanol mixture (weight ratio of 1
:
1 g/g) for 6 h and subsequently immersed in a solution of epoxy resin for 12 h to completely swap the ethanol in the sample for the epoxy resin solution. The epoxy-resin-solution-impregnated sample was embedded in a silicon mold; subsequently, the epoxy resin solution was poured into the mold and cured at 343 K for 5 d. The resin block embedding the gel sample was subsequently thin-sectioned using an ultramicrotome (UC7, Leica Microsystems GmbH, Wetzlar, Germany), and sections of thickness 100 nm were collected on a copper mesh TEM grid with a microgrid mesh and observed by FE-TEM. The acceleration voltage of the electron gun used for observation was 200 kV.
SiO2/PDMAAm ratio measurement
Thermogravimetric (TG) measurement of the inorganic/organic composite skeleton was performed to calculate the SiO2/PDMAAm ratio. The inorganic/organic composite skeleton was obtained from the inorganic/organic NC ion gels via IL extraction using a sufficient amount of ethanol followed by drying in vacuum at an elevated temperature. The TG measurement was conducted using open Pt pans on a thermogravimetry-differential thermal analysis device (TG-DTA Thermo plus EVO II, Rigaku Co., Tokyo, Japan) from 373 to 1273 K at a heating rate of 10 K min−1 under pure air (TAIYO NIPPON SANSO CORPORATION, Tokyo, Japan) atmosphere. Before the measurement, the sample atmosphere was maintained at 373 K for 2 h to remove the absorbed water. The PDMAAm weight was obtained as the weight loss from 373 to 1273 K. The SiO2 weight was obtained as the residual weight at 1273 K.
Results and discussion
Mechanical behavior of the inorganic/organic NC ion gels
Fig. 1 shows the cyclic loading–unloading curves of the inorganic/organic DN and NC ion gels. As shown in Fig. 1(a), the inorganic/organic DN ion gel showed a clear mechanical hysteresis in the cyclic loading–unloading. The mechanical hysteresis observed in the inorganic/organic DN ion gel was resulted from the energy dissipation via the internal fracture of the silica particle network.28 However, in the case of the inorganic/organic NC ion gel, as shown in Fig. 1(b), the stress–strain curve did not show a clear mechanical hysteresis. In other words, the mechanical property of the NC ion gel was almost elastic. It was reported that SR hydrogels did not indicate any mechanical hysteresis.27 Therefore, this almost elastic mechanical property of the NC ion gel was consistent with that of SR hydrogel. The very small mechanical hysteresis observed in the cyclic loading–unloading curves in the NC ion gel would be attributed to the fracture of aggregated silica nanoparticles because silica particles were generally aggregated in the IL medium by interparticle attraction forces such as hydrogen bonding and van der Waals interaction.29–33 However, comparing the stress–strain curves of the DN and NC ion gels, the hysteresis of the NC ion gel was much smaller than that of DN ion gel. Therefore, it can be considered that the extent of the silica nanoparticle aggregates ruptured in the NC ion gels would be much smaller than that in the DN ion gels.
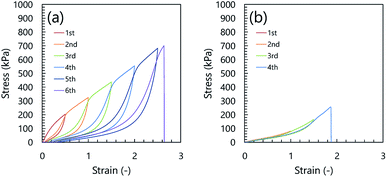 |
| Fig. 1 Cyclic loading–unloading curves of inorganic/organic DN ion gel (a) and inorganic/organic NC ion gel (b). The SiO2/PDMAAm weight ratio of the inorganic/organic DN and NC ion gels were 0.20 and 0.22, respectively. | |
On the other hand, we evaluated the relationship between toughness and dissipated energy of three kinds of inorganic/organic ion gels, i.e. NC, DN, and μ-DN ion gels. Here, μ-DN ion gel is a special type of DN ion gel with partially developed silica particle network.34 The relationship between the dissipated energy and toughness of the NC, DN, and μ-DN ion gels with the same inorganic network composition is shown in Fig. 2. In this figure, it is clearly shown that the relationship between the dissipated energy and toughness of the DN and μ-DN ion gels showed the same trend. On the other hand, the NC ion gels showed a different tendency, i.e. the toughness was much higher than that of DN and μ-DN ion gels. Thus, it could be considered that the toughening mechanism of the NC ion gel would be completely different from the DN and μ-DN ion gels.
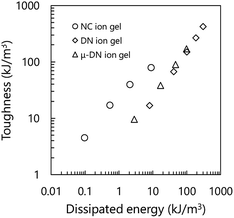 |
| Fig. 2 Relationship between toughness and dissipated energy of inorganic/organic NC, DN, and μ-DN ion gels. The NC, DN, and μ-DN ion gels are prepared from the same precursor solution with 0.17 of TEOS/DMAAm molar ratio. | |
Effect of silica particle content on the mechanical properties of inorganic/organic NC ion gels
In the SR hydrogels, the stress–strain behavior is affected by the cross-linking density of the polyrotaxane network. For example, the fracture stress of the SR gels increased with increasing cross-linking density while the fracture strain was almost constant.27 Therefore, if the toughening mechanism of the NC ion gel is the same as that of the SR gels, the fracture stress of the NC ion gels would be increased by increasing the cross-linking density of the silica particle-based aggregates. The cross-linking density could be controlled by the silica particle content. Thus, to evaluate the effect of cross-linking density of the silica particle-based aggregates on the mechanical properties of the NC ion gels, we prepared the NC ion gels with various silica particle contents with an almost constant PDMAAm content. The experimentally determined composition of the prepared inorganic/organic NC ion gels are summarized in Table 1.
Table 1 Compositions of inorganic/organic NC ion gels prepared from precursor solutions with various TEOS/DMAAm molar ratios. The IL contents are obtained via IL extraction using sufficient ethanol followed by drying in a vacuum at an elevated temperature. The SiO2 and PDMAAm contents in the inorganic/organic skeleton were calculated from the weight ratio of a silica particle and PDMAAm, which were obtained by TG measurement
TEOS/DMAAm molar ratio |
IL content (wt%) |
Silica particle content (wt%) |
PDMAAm content (wt%) |
SiO2/PDMAAm weight ratio |
0.5 |
77.3 |
5.5 |
17.2 |
0.32 |
0.33 |
79.4 |
3.6 |
17.0 |
0.22 |
0.25 |
81.1 |
2.6 |
16.3 |
0.16 |
0.20 |
82.1 |
1.9 |
15.9 |
0.12 |
0.17 |
82.3 |
1.8 |
16.0 |
0.11 |
0.10 |
82.4 |
1.1 |
15.5 |
0.07 |
Fig. 3 shows the stress–strain curves of the inorganic/organic NC ion gels with various SiO2/PDMAAm weight ratios. In Fig. 3, the line (a) (SiO2/PDMAAm = 0) corresponds to the result of PDMAAm SN ion gel. It can be clearly found that the shapes of the stress–strain curves of the inorganic/organic NC ion gels were different from that of the PDMAAm SN ion gel. For the PDMAAm SN ion gel, the stress–strain curve showed no significant stress increase at high strain region. Meanwhile, the stress–strain curves of the inorganic/organic NC ion gels showed a significant stress increment at the high strain region, which was similar to the specific mechanical behavior of SR gels.27 In addition, the inorganic/organic NC ion gels indicated a higher fracture stress than the PDMAAm SN ion gel. From these results, it was confirmed that the mechanical property of the inorganic/organic NC ion gels were significantly affected by the silica particle content. The effect of silica particle content on the fracture stress and fracture strain of the inorganic/organic NC ion gels are shown in Fig. 4(A) and (B), respectively. As shown in Fig. 4(A) and (B), the fracture stress of the inorganic/organic NC ion gels increased with the increase of the SiO2/PDMAAm weight ratio although the fracture strain was almost constant in the range of 1.5–1.9. The properties shown in Fig. 3, 4(A) and (B) were consistent with those of SR gels.27
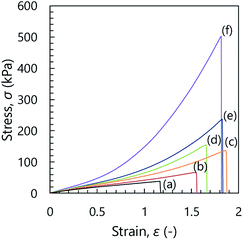 |
| Fig. 3 Stress–strain curves of the inorganic/organic NC ion gels. SiO2/PDMAAm (weight ratio) = 0 (a), 0.07 (b), 0.11 (c), 0.16 (d), 0.22 (e), and 0.32 (f). Line (a) corresponds to PDMAAm SN ion gel. | |
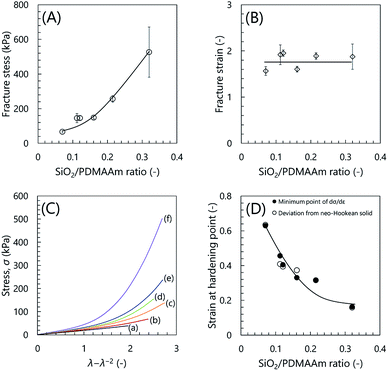 |
| Fig. 4 (A), (B) Fracture stress and fracture strain as a function of SiO2/PDMAAm weight ratio, respectively. (C) Relationship between σ and λ − λ−2 of the inorganic/organic NC ion gels. SiO2/PDMAAm (weight ratio) = 0 (a), 0.07 (b), 0.11 (c), 0.16 (d), 0.22 (e), and 0.32 (f). Line (a) corresponds to PDMAAm SN ion gel. (D) Strain at hardening point as a function of SiO2/PDMAAm weight ratio. | |
Furthermore, we evaluated the strain at the hardening point. We plotted stress σ against λ − λ−2, where λ is strain +1. As shown in Fig. 4(C), the PDMAAm SN ion gel showed linear relationship between σ and λ − λ−2. This indicated that the SN ion gel was neo-Hookean solid. On the other hand, the inorganic/organic NC ion gels showed non-linear relationship. The relationship deviated from σ = G(λ − λ−2), where G is elastic modulus. This indicated the strain hardening occurred in the NC ion gels. We defined that the strain at the hardening point is that at which the difference between the applied σ and the calculated σ from G(λ − λ−2) was 1 kPa. The strains at the hardening points determined from the above analysis were shown in Fig. 4(D). Additionally, to increase the reliability of the hardening point, we determined the strain at the hardening point as the strain at the local minimum of the differential stress–strain curve (dσ/dε), where ε is strain. The relationship between dσ/dε and strain was shown in Fig. S1.† The determined strains at the hardening points were plotted in Fig. 4(D). As shown in Fig. 4(D), the determined strain at the hardening point monotonically decreased with increasing silica particle content.
At the hardening point, the load applied to the gel network suddenly increases. This would mean that a large parts of gel networks became elongated state to sustain the applied load. If the gel network was randomly developed and had no special structure, the number of the gel network sustaining the applied force should gradually increase, as shown in the result of SN ion gel (Fig. 3(a)). In other words, the sudden increase of the applied stress to the gel suggested that the NC ion gel would have characteristic network structure, which was different from the SN and DN ion gels. In addition, it was considered that the ratio of the characteristic structure in whole of the developed network would be increased by the increase of the silica nanoparticles in the NC ion gel.
In order to confirm the silica nanoparticle originated characteristic network structure in the NC ion gel, we then observed the structure of the silica nanoparticles formed in the NC ion gels using TEM.
Network structure of inorganic/organic NC ion gels
The structure of silica nanoparticles in the inorganic/organic NC ion gels was observed by TEM. The TEM images of the inorganic/organic NC ion gel are shown in Fig. 5. The dark part in the TEM images indicates the inorganic silica particles. As shown in Fig. 5(a) and (b), the silica particles formed very small aggregates, which were secondary silica nanoparticles, and the secondary silica nanoparticles were well dispersed in the inorganic/organic NC ion gel. The structure of the well-dispersed secondary silica nanoparticles in the inorganic/organic NC ion gel completely differed with the case of the inorganic/organic DN ion gel, which was composed of network-like silica nanoparticle aggregates with approximately several hundred nanometers shown in Fig. S2.†34 Owing to the difference in size of the silica particle aggregates, the inorganic/organic NC ion gel was transparent although the inorganic/organic DN ion gel was translucent (Fig. S3†). In addition, because of the very small size of the secondary silica particles formed in the NC ion gel, it could be considered that the number of the secondary silica particles interpenetrating the polymer network was small. Therefore, the ruptured secondary silica particles during stretching test would be very small. As the result, as shown in Fig. 1, energy dissipated by the rupture of the secondary silica nanoparticles would be much smaller than that of the DN ion gel.
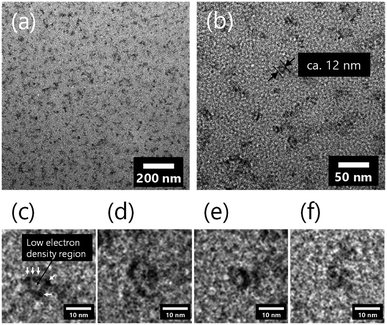 |
| Fig. 5 Electron microscopy images of silica nanoparticles in inorganic/organic NC ion gel with SiO2/PDMAAm weight ratio = 0.32. (a) Over view, (b) magnified view, and (c–f) ring-like silica nanoparticle aggregates. The white arrows in the (c) mean primary particles with ca. 3 nm diameter. | |
Subsequently, we discuss the detailed structure of the silica particles in the inorganic/organic NC ion gel. As shown in Fig. 5(c)–(f), secondary silica particles with approximately 12 nm diameter were composed of primary silica nanoparticles with approximately 3 nm diameter as indicated in Fig. 4(c) with white arrows. In addition, a low electron density region, which is shown as a lighter part than the primary silica particle in the TEM image shown in Fig. 5(c)–(f), appeared around the center of the secondary silica particles. It could be assumed that the low electron density region contained not only amorphous SiO2, but also low electron density materials such as organic polymers. That is, the PDMAAm chain would be bored through the secondary silica particle. As the result, it was confirmed that a characteristic gel network composed of ring-like silica nanoparticle aggregates and PDMAAm chains were formed in the NC ion gel.
Possible toughening mechanism of the inorganic/organic NC ion gels
Considering the experimental results and comparing the mechanical properties of the NC ion gel and SR hydrogel, we considered several possibilities on the role of the silica nanoparticles on the increase of the mechanical strength of the NC ion gel.
Recently, Watanabe et al. reported that the inorganic/organic ion gel prepared using surface modified silica particles having negligibly low interaction between the silica nanoparticles had very low mechanical strength.35 From this report, it is considered that the well-dispersed silica nanoparticles themselves could not increase the strength of the NC ion gel if the silica nanoparticles did not interact with polymer network. In other words, it could be said that the silica nanoparticles should have some interaction with each other or with PDMAAm to toughen the NC ion gel.
On the other hand, if the silica nanoparticles had strong interaction with the polymer network, the adsorption of PDMAAm to silica particles will be occurred. Such adsorption was confirmed in silica particle/PDMAAm composite hydrogel system.36 If the adsorption of PDMAAm on the silica nanoparticles occurred in our developed NC ion gels, the ion gels should show not only high mechanical strength but also large mechanical hysteresis in the cyclic stress loading–unloading test. However, the NC ion gels did not show large hysteresis. In addition, in our previous study, we demonstrated that PDMAAm hardly adsorbed on the silica particles in the ionic liquid medium.28 Therefore, the adsorption of PDMAAm on silica particle cannot be the toughening mechanism of the inorganic/organic NC ion gel.
In addition, as shown in the TEM images of the NC ion gels (Fig. 5(a) and (b)), the silica nanoparticles formed no network-like structure in the NC ion gels. Therefore, the energy dissipation mechanism seen in the DN ion gel system was not the toughening mechanism of the NC ion gel.
Here, considering the experimental results in this work, from the TEM observation, it was suggested that the PDMAAm network and silica nanoparticles formed a characteristic ring-like structure of which PDMAAm bored through the very small silica nanoparticle-based aggregates consisted of a few silica nanoparticles. If silica nanoparticles formed such ring-like structure in the NC ion gel, it can be considered that the characteristic network could increase the mechanical strength of the NC ion gel according to the following two possible mechanisms. The first conceivable mechanism is the energy dissipation via the internal fracture of the small silica particle aggregates. However, in the cyclic stress loading–unloading test, the NC ion gel did not show large mechanical hysteresis (Fig. 1(b)). Thus, it is hard to consider that the energy dissipation along with the internal rupture of the silica nanoparticle aggregates was the main mechanism to toughen the NC ion gel. The other conceivable mechanism was the energy dispersion mechanism owing to the silica nanoparticle aggregates-based movable cross-linker. As mentioned before, the characteristic mechanical properties of the NC ion gels, such as the drastic stress increase at the high strain state (Fig. 3) and very low mechanical hysteresis (Fig. 1(b)), were also very similar to the mechanical property of SR gels. Therefore, because of the estimated network structure and the characteristic mechanical properties, we consider that the energy dispersion owing to the silica nanoparticle aggregate-based movable cross-linker would be the toughening mechanism of the NC ion gels.
The expected formation mechanism of the secondary silica particles incorporating PDMAAm chains in the inorganic/organic NC ion gel is shown in Fig. 6. The silica particle in inorganic/organic NC ion gel grew within the highly developed PDMAAm network. Therefore, it was reasonable to consider that the nuclear growth of the silica particle started on the PDMAAm chain and subsequently, secondary silica particles grew around the PDMAAm chains. This implies that PDMAAm chains were incorporated in a secondary silica particle via the nuclear growth of the silica particle. As the result, the low electron density region in the secondary silica particles were observed in the TEM images of the NC ion gels. According to this hypothesis, it could be considered that the secondary silica particles incorporating a PDMAAm chain acted as a movable cross-linker for the PDMAAm network in the inorganic/organic NC ion gels.
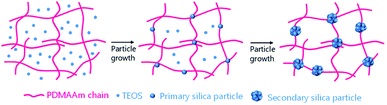 |
| Fig. 6 Schematics of formation mechanism of secondary silica particle incorporating PDMAAm chains in the inorganic/organic NC ion gels. | |
Based on these results, the schematics of the estimated network structure of the inorganic/organic NC ion gel are as shown in Fig. S4.† The gel network of the inorganic/organic NC ion gel was composed of secondary silica particles and a PDMAAm network. The secondary silica particles dispersed well in the gel and incorporated PDMAAm chains via particle growth. The secondary silica particles incorporating more than two PDMAAm chains served as a movable cross-linker in the gel, while the secondary silica particle incorporating only one PDMAAm chain was not a cross-linker.
Conclusion
In this study, the reasonable network structure and toughening mechanism of the inorganic/organic NC ion gel were estimated. Structural observation results indicated that the secondary silica particles were well dispersed in the inorganic/organic NC ion gel. In addition, the secondary silica particles would incorporate PDMAAm chains. Based on the network formation process, the silica particle grew in the highly developed PDMAAm network. In this case, the nuclear growth of silica particle would start on the PDMAAm chain. Therefore, it could be considered that the secondary silica particles formed ring-like structure, incorporated PDMAAm chains, and acted as a movable cross-linker of the PDMAAm chains. Because of the sliding motion by secondary silica particles, the inorganic/organic NC ion gels could exhibit characteristic stress increase when it was highly elongated.
Conflicts of interest
There are no conflicts to declare.
Acknowledgements
The authors thank the Research Facility Center for Science and Technology of Kobe University for providing FE-TEM for this study. Parts of this work were supported by KAKENHI (18K04812 and 19J11528) of the Japan Society for the Promotion of Science (JSPS).
References
- A. Noda and M. Watanabe, Electrochim. Acta, 2000, 45, 1265–1270 CrossRef CAS.
- J. Ding, D. Zhou, G. Spinks, G. Wallace, S. Forsyth, M. Forsyth and D. MacFarlane, Chem. Mater., 2003, 15, 2392–2398 CrossRef CAS.
- T. Ueki and M. Watanabe, Macromolecules, 2008, 41, 3739–3749 CrossRef CAS.
- J. E. Bara, E. S. Hatakeyama, D. L. Gin and R. D. Noble, Polym. Adv. Technol., 2008, 19, 1415–1420 CrossRef CAS.
- T. P. Lodge, Science, 2008, 321, 50–51 CrossRef CAS PubMed.
- M. Armand, F. Endres, D. R. MacFarlane, H. Ohno and B. Scrosati, Nat. Mater., 2009, 8, 621–629 CrossRef CAS PubMed.
- J. Lu, F. Yan and J. Texter, Prog. Polym. Sci., 2009, 34, 431–448 CrossRef CAS.
- J. Le Bideau, J. B. Ducros, P. Soudan and D. Guyomard, Adv. Funct. Mater., 2011, 21, 4073–4078 CrossRef CAS.
- D. L. Gin and R. D. Noble, Science, 2011, 332, 674–676 CrossRef CAS PubMed.
- S. Imaizumi, H. Kokubo and M. Watanabe, Macromolecules, 2012, 45, 401–409 CrossRef CAS.
- S. Kasahara, E. Kamio, R. Minami and H. Matsuyama, J. Membr. Sci., 2013, 431, 121–130 CrossRef CAS.
- S. Kasahara, E. Kamio, A. Yoshizumi and H. Matsuyama, Chem. Commun., 2014, 50, 2996–2999 RSC.
- F. Moghadam, E. Kamio, A. Yoshizumi and H. Matsuyama, Chem. Commun., 2015, 51, 13658–13661 RSC.
- F. Ranjbaran, E. Kamio and H. Matsuyama, J. Membr. Sci., 2017, 544, 252–260 CrossRef CAS.
- K. Fujii, A. Hanako, T. Ueki, T. Sakai, S. Imaizumi, U. Chung, M. Watanabe and M. Shibayama, Soft Matter, 2012, 8, 1756–1759 RSC.
- Y. Gu, S. Zhang, L. Martinetti, K. H. Lee, L. D. McIntosh, C. D. Frisbie and T. P. Lodge, J. Am. Chem. Soc., 2013, 135, 9652–9655 CrossRef CAS PubMed.
- H. Arafune, S. Honma, T. Morinaga, T. Kamijo, M. Miura, H. Furukawa and T. Sato, Adv. Mater. Interfaces, 2017, 4, 1700074 CrossRef.
- Y. Ding, J. Zhang, L. Chang, X. Zhang, H. Liu and L. Jiang, Adv. Mater., 2017, 29, 1704253 CrossRef PubMed.
- F. Moghadam, E. Kamio and H. Matsuyama, J. Membr. Sci., 2017, 525, 290–297 CrossRef CAS.
- F. Moghadam, E. Kamio, T. Yoshioka and H. Matsuyama, J. Membr. Sci., 2017, 530, 166–175 CrossRef CAS.
- E. Kamio, T. Yasui, Y. Iida, J. P. Gong and H. Matsuyama, Adv. Mater., 2017, 29, 1704118 CrossRef PubMed.
- Y. Okumura and K. Ito, Adv. Mater., 2001, 13, 485–487 CrossRef CAS.
- K. Ohmori, I. Abu Bin, T. Seki, C. Liu, K. Mayumi, K. Ito and Y. Takeoka, Chem. Commun., 2016, 52, 13757–13759 RSC.
- K. Kato, Y. Okabe, Y. Okazumi and K. Ito, Chem. Commun., 2015, 51, 16180–16183 RSC.
- A. B. Imran, K. Esaki, H. Gotoh, T. Seki, K. Ito, Y. Sakai and Y. Takeoka, Nat. Commun., 2014, 5, 5124 CrossRef PubMed.
- K. Kato, Y. Ikeda and K. Ito, ACS Macro Lett., 2019, 8, 700–704 CrossRef.
- K. Ito, Polym. J., 2007, 39, 489–499 CrossRef CAS.
- T. Yasui, S. Fujinami, T. Hoshino, E. Kamio and H. Matsuyama, Soft Matter, 2020, 16, 2363–2370 RSC.
- K. Ueno, K. Hata, T. Katakabe, M. Kondoh and M. Watanabe, J. Phys. Chem. B, 2008, 112, 9013–9019 CrossRef CAS PubMed.
- K. Ueno, A. Inaba, M. Kondoh and M. Watanabe, Langmuir, 2008, 24, 5253–5259 CrossRef CAS PubMed.
- J. Nordström, L. Aguilera and A. Matic, Langmuir, 2012, 28, 4080–4085 CrossRef PubMed.
- K. Ueno, S. Imaizumi, K. Hata and M. Watanabe, Langmuir, 2009, 25, 825–831 CrossRef CAS PubMed.
- H. C. Chang, T. C. Hung, S. C. Chang, J. C. Jiang and S. H. Lin, J. Phys. Chem. C, 2011, 115, 11962–11967 CrossRef CAS.
- T. Yasui, E. Kamio and H. Matsuyama, Langmuir, 2018, 34, 10622–10633 CrossRef CAS PubMed.
- T. Watanabe, R. Takahashi and T. Ono, Soft Matter, 2020, 16, 1572–1581 RSC.
- W. C. Lin, W. Fan, A. Marcellan, D. Hourdet and C. Creton, Macromolecules, 2010, 43, 2554–2563 CrossRef CAS.
Footnote |
† Electronic supplementary information (ESI) available: Differential stress–strain curve of inorganic/organic NC ion gel and PDMAAm SN ion gel, TEM image of inorganic/organic DN ion gel, photographs of inorganic/organic NC, DN, and PDMAAm SN ion gel, and toughening mechanism of inorganic/organic NC ion gel. See DOI: 10.1039/d0ra02478c |
|
This journal is © The Royal Society of Chemistry 2020 |
Click here to see how this site uses Cookies. View our privacy policy here.