DOI:
10.1039/D0RA02916E
(Paper)
RSC Adv., 2020,
10, 25073-25088
BiOCl/WS2 hybrid nanosheet (2D/2D) heterojunctions for visible-light-driven photocatalytic degradation of organic/inorganic water pollutants
Received
31st March 2020
, Accepted 15th June 2020
First published on 1st July 2020
Abstract
This report presents the superior visible-light-driven photocatalytic response of novel 2D/2D BiOCl/WS2 (BWX) hybrid nanosheet heterojunctions prepared by a simple solution based sonochemical technique. These BWX hybrid nanosheets are composed of 2D transition metal dichalcogenide material WS2 and BiOCl nanosheets. The comparative study of photocatalytic activity of BiOCl and BiOCl/WS2 hybrid nanosheets is carried out via photodegradation of Malachite Green (MG) and photoreduction of heavy metal ion Cr(VI) under visible light irradiation. The quantum efficiency of the samples is estimated in terms of the incident photon to electron conversion efficiency (IPCE) measurements. Nearly 98.4% of the MG degradation was achieved over BiOCl/WS2 (2%) photocatalyst in 45 min of irradiation. BiOCl/WS2 (2%) hybrid nanosheet catalyst showed the highest external quantum efficiency (EQE) in both the UV and visible regimes. This accomplishment demonstrated the promise of commercial application of the 2D/2D BiOCl/WS2 (2%) hybrid nanosheet photocatalyst.
1. Introduction
The crises of global energy and increasing pollution in the environment are the two major concerns that the whole world would be looking for immediate yet sustainable solutions.1,2 The utilization of non-conventional sources of energy such as wind and visible light can be the most suitable remediation for the energy crisis and pollution. Among the sources of different renewable energy, using solar energy for the degradation of pollutants is of high demand.3–5 Since the first report on TiO2 catalyzed water splitting under the UV irradiation by Fujishima and Honda,6 there has been a plethora of examples of hydrogen gas production via water splitting using solar energy and water disinfection by using photocatalytic degradation of water pollutants.7–14 The photocatalytic degradation of water pollutants is a very promising technology being an eco-friendly, low cost technique and having a lack of any consequential contamination.15–17 A large variety of semiconductor nano-heterostructures have been proposed and fabricated to date that contribute to the advancement of photocatalytic conversion technology.18–21 However, making a photocatalyst with high efficiency, low recombination rate and maximum visible light absorption in the full spectral range is still a challenge.
The most commonly used organic dyes in the textile, pharmaceutical, and cosmetic industries are Methyl orange; Malachite green; Rhodamine B; Methylene blue; Congo red, etc. When these organic dyes are discharged into water resources, they can be very harmful.22–25 Malachite Green (MG), a water-soluble cationic dye used as an antifungal, antimicrobial and a topical antiseptic in aquaculture since 1930,26,27 has a structure similar to carcinogenic triphenylmethane dyes. Besides, the effluent containing Cr(VI) ions, the inorganic heavy metal water pollutant, is very toxic and detrimental to the environment.28 Various common techniques are being used to take out Cr(VI) from water viz. ultra-filtration, adsorption, reverse osmosis, etc.29 However, these methods have high power consumption and high operating cost. In an alternate approach for the removal of organic/inorganic pollutants of water, the development of an efficient photocatalyst that can work in the entire solar spectrum (that reaches our earth's surface), is of utmost priority because of low operating cost.30 Many materials, like α-Ga2O3, activated carbon from rice husk, and Cocos nucifera has been investigated and used as photocatalysts by researchers.31 However, the degradation efficiency of these materials was found to be less than 65% even after using a comparatively higher dosage of catalyst. This lead the researchers to find a new class of materials that include, Fe doped TiO2, Ag3PO4 modified by MWCNT's, UiO-66-NH2(Zr/Hf) and z-scheme based photocatalysts which increased the degradation efficiency to about 85%, but with high photocatalyst dosage. Also, the synthesis of this class of materials is complicated in comparison to the photocatalyst synthesized in the present work.29,32
In the last few years, bismuth-based semiconductors viz. bismuth oxyhalides have attracted many researchers due to their large optical and chemical stability, non-toxicity, cost-effectiveness, and potential application in photocatalytic energy conversion and water disinfection.30,33 One of the oxyhalides of bismuth, BiOCl, a ternary semiconductor (V–VII), has a wide bandgap ranging from ∼3.17–3.54 eV (ref. 34) possessing a layer-type structure consisting of [Bi2O2]2+ and Cl− located in between the layers.35 This type of layered geometry facilitates the generation of an internal electric field useful for escalating the separation of the photo-induced electron–hole pairs leading to photoactivity.36 BiOCl generally gives higher photocatalytic response with UV-light irradiation in comparison to TiO2, due to its layer-type geometry.37 Besides, the wide bandgap of BiOCl degrades the photocatalytic activity in the visible-light regime. Hence, the extension of the photocatalytic properties of BiOCl nanostructures in the visible region has become a topic of interest for many researchers. Various methods, like dehalogenation, surface functionalization, photosensitization effect, metal/non-metal doping, and heterojunction constructions are being applied to modify BiOCl for the enhancement of absorption of light in the UV-visible regime.38–43 Among these, methods, making a heterojunction composed of BiOCl and another semiconductor with narrow bandgap lying in the visible regime having suitably positioned valence band maxima and conduction band minima, can be promising in the enhancement of visible-light harvesting, decreasing the electron–hole recombination rate and increasing the lifespan of the charge carriers. Numerous combinations of heterojunction systems, e.g. Bi2WO6/BiOCl, Bi2S3/BiOCl, g-C3N4/BiOCl, BiOCl/RGO, BiOI/BiOCl, CdS/BiOCl, WO3/BiOCl, BiVO4/BiOCl, NaBiO3/BiOCl, BiOCl/TiO2, etc.44–49 have been investigated by many researchers. It has been found that these heterojunctions show enhanced photocatalytic performance in comparison to their counterparts. However, there is only a single report present to date on the BiOCl/WS2 heterostructure system showing the enhanced photocatalytic response in degrading complex organic dyes.49
Tungsten disulfide (WS2) belongs to the family of 2D transition metal dichalcogenides (TMDs). It possesses a direct bandgap in the range ∼1.35–2 eV that leads to the absorption of light to 910 nm.50 So, the combination of BiOCl and WS2 nanosheets can significantly improve the spectrum absorption efficiency via bandgap modification and efficient charge transfer. Hence, a detailed investigation of the BiOCl/WS2 heterostructure system is important for the water remediation by photocatalytic degradation of the pollutants.
In the present study, the 2D/2D nanostructure composite of WS2 nanosheets and BiOCl nanosheets were prepared by using a simple solution based sonochemical technique. The study of photodegradation of Malachite Green (MG) and the photoreduction of a very toxic heavy metal ion Cr(VI)) was carried out under visible light irradiation using BiOCl/WS2 hybrid nanosheets. The photocatalytic response of BiOCl/WS2 was significantly affected by the amount of WS2 incorporated. The combination of BiOCl and WS2 nanosheets resulted in the enhancement of the charge carriers' transfer rate, resulting in better photocatalytic activity.
2. Experimental details
2.1 Reagents and materials
In the present work, all the chemicals used were of analytical grade unless otherwise mentioned and were used as they were bought from the supplier without any additional purification. Sodium tungstate dihydrate (Na2WO4·2H2O, 96%) and thiourea (CH4N2S) were bought from the Thermo Fischer Scientific India (P) Ltd., Mumbai, India. Hydroxylamine hydrochloride (NH2OH·HCl, 98%) and N-cetyl-N,N,N-trimethyl ammonium bromide (CTAB, 98%) were purchased from Central Drug House (P) Ltd., New Delhi, India. The pH value of the solution was maintained using buffer capsules (Merck, India). Potassium dichromate (K2Cr2O7, Merck, India, 99.99%) was used as a source of chromium. Bismuth nitrate pentahydrate (reagent grade, Bi (NO3)3·5H2O, 98%), HCl (37%), NaOH (≥97%, pellets), n-propanol (99.7%), and ethanol (99.9%, AR grade) were sourced from Sigma Aldrich, India.
2.2 Synthesis of WS2 nanosheets
The solution was prepared by mixing 0.005 M sodium tungstate, 0.02 M thiourea, and 0.01 M hydroxylamine hydrochloride in 30 ml DI water. In this solution, 0.24 g CTAB was added and stirred constantly for 60 min. The measured pH value of the white-colored precipitate thus formed was 6.15. The solution was then poured into a teflon lined autoclave. For 24 h the autoclave was kept in a furnace at a temperature of 180 °C. After 24 h the autoclave was taken out from the furnace and kept at room temperature for natural cooling. After natural cooling, the solution was filtered. The solid content thus recovered was washed with DI water and ethanol. Then the content was dried at a temperature of 30 °C inside the furnace for 4 h.51 The chemical reactions involved in the synthesis of WS2 nanosheets are as follows: |
4CH4N2S + Na2WO4 + 4H2O → Na2WS4 + 8NH3 + 4CO2
| (1) |
|
Na2WS4 + 2NH2OH·HCl → WS2 + 2H2S + 2NaCl + N2+ 2H2O
| (2) |
2.3 Synthesis of WS2 nanosheets and BiOCl nanosheets hybrid
A solution of n-propanol (10 ml) and hydrochloric acid (2 M, 25 ml) was prepared in 20 ml DI water. In this solution, 1.86 g Bi(NO3)3·5H2O and a measured amount of WS2 nanosheets were added. The whole solution thus formed was then ultrasonicated for 30 min to get a uniform suspension. The suspension thus formed was turned white steadily after adding ∼10 ml of 4 M NaOH aqueous solution, drop by drop. The pH value of the mixture was found to be 8.0. The suspension was mixed with stirrer for 30 min and then kept as it is for 6 h. The formed resultant precipitates formed were centrifuged and washed using ethanol and DI water thoroughly. At last, the precipitates were dried at 100 °C for 12 h to achieve BiOCl/WS2 hybrid nanosheets (Fig. 1).
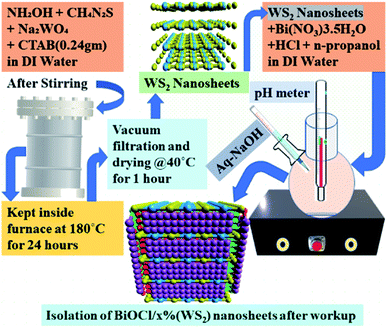 |
| Fig. 1 Schematic diagram of the synthesis of BWX (X varies from 0% to 5%) hybrid nanosheets. | |
A series of BiOCl/WS2 hybrid nanosheets by varying the wt% of WS2 from 0% to 5% were synthesized and denoted as BWX hybrid nanosheets, where X denotes the weight percentage of WS2 nanosheets to BiOCl. Pure BiOCl nanosheets (BW0) were synthesized under similar conditions without the addition of WS2 nanosheets.
2.4 Characterization
FESEM (Field Emission Scanning Electron Microscopy) study was carried out using Zeiss, Sigma Field Emission Scanning Electron Microscope, to investigate the morphology of the samples. EDX (Energy Dispersive X-ray) spectra taken from FESEM were used for composition analysis. Crystal structure and phase identification studies were carried out through X-ray diffractogram (XRD Smart Lab Guidance, Rigaku) were recorded by using Cu Kα source with wavelength, λ = 1.5418 Å. The Raman scattering measurements were conducted using Alpha 300 RAS TS-150 Witec Confocal Raman Microprobe equipped with 365 nm and 532 nm argon-ion lasers. The absorption of light in 300–800 nm range was investigated by UV-vis diffuse reflectance spectroscopy (UV-vis Cary Series, Agilent Technologies). Photocatalytic activity of the BWX hybrid nanosheets for MG degradation and highly toxic Cr(VI) ion reduction was analyzed using the UV-vis spectrometer (UV-vis Cary Series, Agilent Technologies). Fourier transform infrared spectroscopy (FTIR) (Vertex 70V, Bruker) was used to study the functional groups and bond structure. The lifetime of charge carriers was evaluated by using the Time-Correlated Single-Photon Counting (TCSPC) (Horiba DeltaFlex-01-DD) measurement spectrometer at an excitonic emission wavelength of 280 nm with typical short optical pulses from <100 ps optical FWHM, spectral FWHM < 15 nm in pulsed mode, 100 MHz maximum repetition rate, and with an instrument response function of ∼200 ps. The emission decays were deconvolved with IRF using EZ time software. The double-exponential kinetics model was used to fit the decay curves of the samples and the fitting was done in the best fitness limits, with χ2 in the range 1–1.2. The relationship between the photoactivity improvement and the wavelength of the incident light, and the external quantum efficiency of the samples were investigated by using Incident Photon to Converted Electron (IPCE) studies. For IPCE measurements, 100 mg of photocatalyst was compressed under a pelletizer using a 10 mm stainless steel die and the applied force was 50 kN. The thin pellet with 10 mm diameter was annealed for 2 hours at a temperature of 50 °C. For electrode preparation, silver paste was applied to one of the faces of the pellet and a thin copper wire was placed on the silver pasted face and again the silver paste was applied to the copper wire at the contact point as well to make the ohmic contact. The silver paste was allowed to dry for at least 1 hour to make sure that the proper contact is established. The other end of the wire was inserted through thin hollow glass pipe with 2 mm diameter and 3-inch height. One end of the pipe was kept close to the edge of the pellet and at this end, resin was applied to the copper wire, silver pasted face, edge of pellet and thin pipe in such a way that only the bare side of pellet could come in contact with electrolyte when dipped in it. In this case, 1 molar sodium sulphate (Na2SO4) was used as an electrolyte. After the whole setup was kept in place, a linear sweep voltammetry was performed with the help of Autolab PGSTAT302N potentiostat and simultaneously the bare face of the sample was illuminated with the help of monochromator which was controlled by lab view programme to automatically select a particular wavelength (in the range of 200–700 nm) for measurement of electrode current as a function of wavelength.
2.5 Photocatalytic experiment
As-synthesized samples were investigated for their photocatalytic properties by Malachite Green (MG) degradation under visible light. A green-colored solution was prepared by mixing 1 mg of MG in 100 ml of DI water. The solution was then kept in the dark for half an hour. After half an hour, 50 mg BWX (X = 0%, 2%, and 4%) was added in the solution. Again, the solution was kept in the dark for half an hour. Then, the solution was irradiated in visible light by using xenon arc lamp with a power of 100 mW cm−2 with AM 1.5 filter. Immediately 1 ml solution was transferred into an eppendorf as the first sample and the subsequent samples were collected each after 15 min for 1.5 h. The collected samples were centrifuged to study the absorption spectra of supernatants. The photocatalytic performance of BWX hybrid nanosheets was further tested for the Cr(VI) ions photoreduction. A solution of 1 mg of Cr(VI) ions was prepared in 100 ml of DI water. Then 50 mg of the photocatalyst was added in this solution. Taking the first sample at 0 min, 1 ml sample was taken out of the solution after every 15 min for 3 h. After this, the collected samples were kept for centrifugation and studied for the absorption spectroscopy. The photodegradation efficiency was calculated by using the formula: |
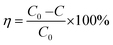 | (3) |
where C0 is the initial concentrations of MG/Cr(VI) solutions and C is the concentration at time t, after irradiation.
3. Results and discussion
3.1 Morphology and crystal structure
All the samples' microstructure was studied by FESEM. Morphology in Fig. 2(a) of BW0 revealed a quite smooth surface with a nanosheet-like structure having round edges and different diameters. The hierarchical plate-like structure for BW0 was in agreement with the previous report.52 Fig. 2(b and f) revealed that the WS2 incorporation significantly altered the morphology of BiOCl nanosheets in BWX nanostructures. After the addition of WS2, the morphology of the heterojunctions changed to a sheet-like structure with sharp edges. However, the further increase in WS2 content (>3%) in BiOCl nanosheets resulted in the agglomeration of the nanosheets. This agglomeration leads to a decrease in active sites for light-harvesting and molecule adsorption.
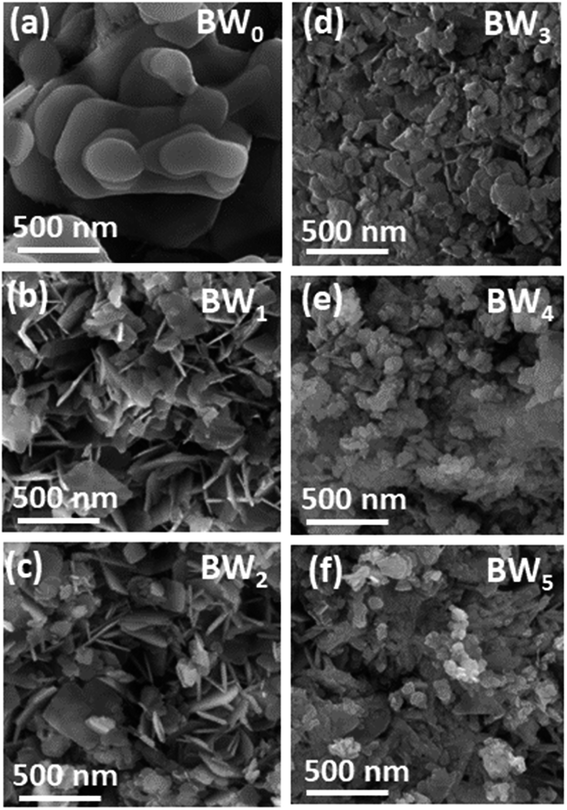 |
| Fig. 2 FESEM images of BWX hybrid nanosheets where X varies from 0% to 5% prepared by sonochemical method. | |
Energy dispersive X-ray (EDX) was used to study the elemental mapping of BW2 to confirm the existence of WS2 in the sample. Fig. 3 depicted that the W and S atoms are evenly spread on the surface of BW2 hybrid nanosheets, that confirmed the existence of WS2. The compositional mapping of BW2 is given in Table 1, quantitatively.
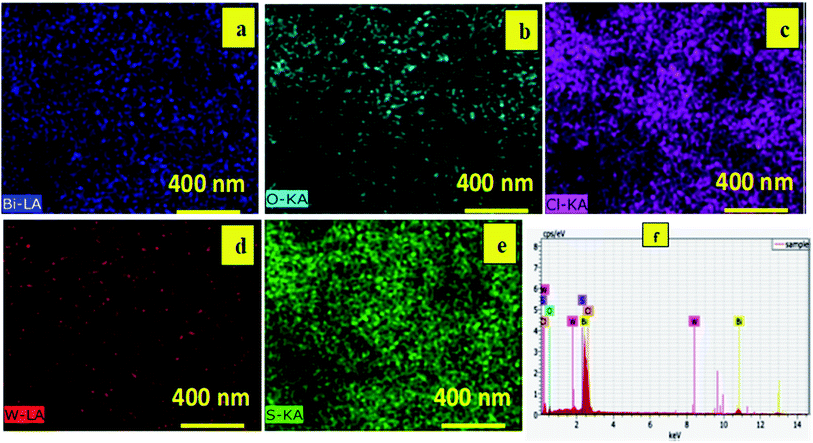 |
| Fig. 3 Elemental mapping of Bi, O, Cl, W and S on the BW2 nanosheets showing (a) blue, (b) aqua, (c) magenta (d) red (e) green, corresponding to bismuth, oxygen, chlorine, tungsten, and sulphur, respectively and (f) EDX spectra of BW2 nanosheets. | |
Table 1 Composition analysis of BW2 nanosheets
Element |
Atomic concentration (%) |
Weight concentration (%) |
Bi |
34 |
96 |
O |
40 |
9 |
Cl |
25 |
12 |
W |
0.6 |
1.6 |
S |
1.3 |
0.6 |
XRD patterns (Fig. 4) of BWX (X varies from 0% to 5%) hybrid nanosheets were analyzed to study the crystallographic structure. The 2θ values corresponding to the observed peaks in all the samples were matched with the 2θ values of standard data (JCPDS # 73-2060) of the XRD peaks of pure BiOCl. It was found that the as-synthesized samples were pure in-phase and crystallized in the expected tetragonal matlockite structure. In all the samples, the XRD peaks displayed the obvious broadening. This broadening of the peaks was attributed to the nanoscale structure of the samples.
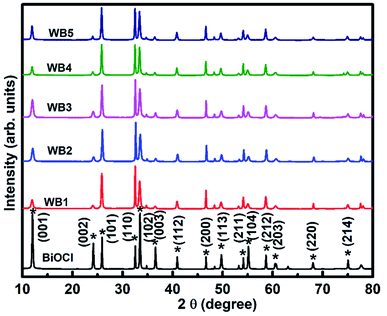 |
| Fig. 4 X-ray diffractograms of BWX hybrid nanosheets where X varies from 0% to 5% prepared by the sonochemical method. | |
The BW0 nanosheets displayed a pronounced (001) texture. With the addition of WS2 in BiOCl nanosheets, crystal structure got reoriented from (001) texture to (110) texture followed by (101) texture. The intensity ratios of (110) and (001) peaks for all BWX hybrid nanosheets are given in Table 2. This reorientation depicts the inhibition of crystal growth along the basal ‘ab’ plane in the case of BWX hybrid nanosheets. As per literature, this texture/faceted helps in setting up internal electric fields, that support the electron–hole separation in this type of material.53 It was thus clearly indicated that increasing WS2 content was accountable for the crystal reorientation in these hybrid nanosheets. This kind of crystallographic reorientation was also being reported by Jia et al. in Bi2S3/BiOCl composites.54 The observed change in crystallographic orientation can be attributed to the transition from reduced surface termination to oxidized surface termination and the surface energy stability associated.
Table 2 Variation of lattice parameters, cell volume (V), and peak intensity ratio (I110/I001) of BWX hybrid nanosheets where X varies from 0% to 5% with the change in WS2 content
Samples |
a (Å) |
c (Å) |
V (Å3) |
Dhkl (nm) |
Intensity ratio (I110/I001) |
BW0 |
3.888 |
7.358 |
111.228 |
118.49 |
0.41 |
BW1 |
3.888 |
7.413 |
112.059 |
105.41 |
5.03 |
BW2 |
3.881 |
7.336 |
110.496 |
102.65 |
3.05 |
BW3 |
3.887 |
7.374 |
111.412 |
98.1 |
2.13 |
BW4 |
3.882 |
7.412 |
111.698 |
81.49 |
3.72 |
BW5 |
3.881 |
7.416 |
111.701 |
79.71 |
2.32 |
The crystallite size of the hybrid nanosheets was evaluated from the Scherrer's formula41 given as follows:
|
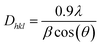 | (4) |
where
Dhkl (nm) represents the average crystallite size,
λ = 0.1540 nm, is the Cu K
α radiation's wavelength,
β (radians) represents the full-width at half-maximum (FWHM) of the peak, and
θ is the Bragg's diffraction angle.
It was discovered that the crystallite size decreases from 118.5 to 79.7 nm with the increase in WS2 content in the BiOCl matrix from 0% to 5% (Table 2). This indicates that the BWX hybrid nanosheets possessed smaller crystallite sizes with a large surface area. In a tetragonal unit cell, the relationship between interplanar spacing ‘d’ of (hkl) planes and the lattice constants ‘a’ and ‘c’ is given as:
|
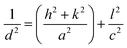 | (5) |
(001) and (200) planes were used to calculate the lattice constants all the samples. The calculated lattice parameters were found to agree with the standard values a = 3.887 Å, c = 7.354 Å, and V0 = 111.11 Å3 corresponding to BiOCl. The non-presence of XRD peaks corresponding to WS2 in the X-ray diffractogram of BWX is attributed to the presence of WS2 in a very low amount in the BiOCl precursor. Similar results were reported in the case of CQDs/BiOI and Co3O4/BiOCl hybrids.55,56
Information regarding vibration along with the crystal structure and stress state was obtained from the Raman spectra of BWX hybrid nanosheets (Fig. 5(a)).
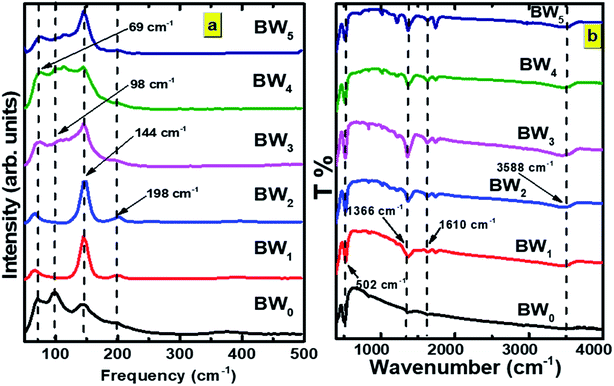 |
| Fig. 5 (a) Raman spectra and (b) FTIR spectra of BWX hybrid nanosheets where X varies from 0% to 5% prepared by the sonochemical method. | |
Five Raman vibration bands were observed for BW0 at 69, 98, 144, 198, and 365 cm−1. Peaks at 144 and 198 cm−1 were dedicated to the A1g and Eg internal mode of Bi–Cl stretching.37 Peaks at the positions 69 and 98 cm−1 were dedicated to the first-order scattering corresponding to Eg and A1g modes of Bi.57 This depicts the presence of the unreacted Bi in the BiOCl matrix. It was observed that the BW1 and BW2 had a sharp peak at 142 cm−1 along with smaller intensity peaks at 67 and 196 cm−1. The high-intensity blue-shifted peak at 142 cm−1 followed by the intensity reduction of the other peaks indicated that the addition of WS2 nanosheets significantly affected the Bi–Cl internal A1g stretching mode. The reduced degrees of freedom along ‘a’ and ‘b’ axes resulted in restricted stretching in the tetragonal crystal lattice of BiOCl.58 The blue shift of the Raman vibrational peaks in the BW1 and BW2 samples evidenced a strong coupling between the BiOCl and WS2 nanosheets. However, the nature of the Raman spectra for BW3, BW4, and BW5 quite similar to that of BW0.
FTIR measurements were carried out to further study the phase transformation of BiOCl and interfacial relations between BiOCl and WS2. FTIR spectra of BWX nanostructures are presented in Fig. 5(b). Bi–O stretching vibration at around 502 cm−1 was observed in BW0 which is common in a tetragonal phase BiOCl crystal. O–H stretching frequency was found at 1366 cm−1 and 1610 cm−1 in BW0. A wide hump around 3588 cm−1 was dedicated to the presence of H2O in BiOCl.32 In the case of the BWX hybrid nanosheets, the intensity of O–H stretching vibrations at 1366 cm−1 and 1610 cm−1 was increased significantly. This implies the presence of a large number of O–H groups at the surface of BWX hybrid nanosheets. The stretching vibration observed around 671 cm−1 and 820–980 cm−1 could be allocated to W–S and S–S for the BWX hybrid nanosheets.42 A steady intensification and a shift of the band slightly at 820–980 cm−1 in BWX was due to the strong coupling of BiOCl and WS2 nanosheets. This strong coupling is vital to escalate the separation and transfer of the charge carriers and consequently increase the photocatalytic performance of the hybrid nanosheets. The prominent absorption band of W–S was not observed for the hybrid nanosheets, indicating that 2D WS2 nanosheets are well distributed over the BiOCl matrix.
3.2 Optical properties
From the photocatalysis point of view, one more significant property of BWX is the absorption of light. The optical properties of BWX, (X = 0%, 2%, and 4%), hybrid nanosheets were evaluated by using the UV-vis diffuse reflectance spectroscopy (DRS). The DRS of the pure BiOCl nanosheets is different from the DRS of BWX hybrid nanosheets (Fig. 6(a)). The BW0 nanosheets showed a sharp fundamental absorption edge at ∼372 nm due to intrinsic bandgap absorption. However, after the incorporation of WS2 nanosheets into BiOCl nanosheets, the absorption edge of the BWX hybrid nanosheets was shifted to the lower wavelength. This difference leads to the change in the bandgap of these samples.
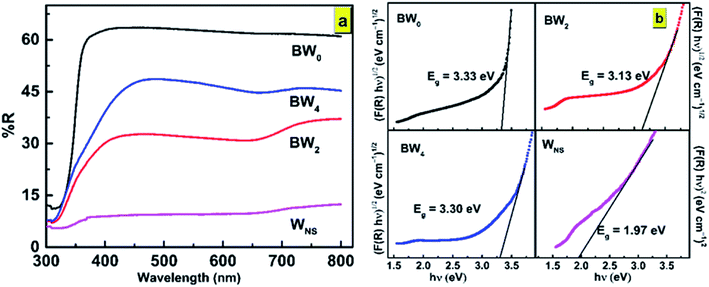 |
| Fig. 6 (a) UV-vis DRS spectra and (b) the plot of (F(R)hν)1/2 vs. hν for determination of bandgap energies of BW0, BW2, BW4, and WNS hybrid nanosheets. | |
3.2.1 Band gap calculations. The bandgap calculation for BW0, BW2, and BW4 hybrid nanosheets and WNS was done by using Kubelka–Munk (K–M) theory from the respective diffuse reflectance spectra (DRS).59,60 The relation between the diffuse reflectance (R) and the absorbance of the material is given by Kubelka–Munk (K–M) function, F(R), as follows: |
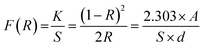 | (6) |
where A is the absorbance of the sample, K is the absorption coefficient, S is the scattering coefficient, and ‘d’ is the thickness of the sample. The scattering coefficient, ‘S’ accounts for the internal scattering. It depends upon the properties of the material like refractive index and particle size. If we take ‘S’ as a constant with respect to wavelength, then F(R) ∝ α, where ‘α’ represents the coefficient of absorption, written as: |
 | (7) |
The bandgap energy (Eg) is given by:
|
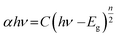 | (8) |
where ‘
h’ represents the Plank's constant, ‘
ν’ represents the frequency of light, ‘
C’ represents the constant of proportionality, and ‘
Eg’ represents the bandgap energy. ‘
n’ is an integer and its value is based on nature of the transition occur in a semiconductor material.
n is taken as 4 for an indirect bandgap material and
n is taken to be 1 for a direct bandgap material. BiOCl has an indirect bandgap. The value of
n was taken to be 4 for BiOCl. However,
n = 1 for WS
2 as it possesses a direct bandgap transition.
The determination of the bandgap of BWX (X varies from 0% to 5%) was done by using the graph: (F(R)hν)1/2 vs. hν (Fig. 6(b)). The bandgap energy of the sample is obtained by extrapolating the linear portion of the corresponding (F(R)hν)1/2 vs. hν plots at (F(R)hν)1/2 = 0. The bandgap energy of the BWX samples is decreased from 3.33 eV to 3.13 eV when the amount of WS2 is increased from 0% to 4%. The strong coupling between BiOCl and WS2 nanosheets resulted in a significant bandgap narrowing near the interface. Hence, the sensitivity of the heterostructures towards the visible light is increased which significantly contributes to the visible-light-driven photocatalysis.
The position of EVB, the valence band edge potential and ECB, the conduction band edge potential for BWX hybrid nanosheets, was calculated by using the Butler and Ginley equation.59
‘X’ represents the absolute electronegativity of the semiconductor calculated from the electronegativity of the atoms, it is composed of, ‘Ee’ (∼4.5 eV) represents the free electrons' energy on the hydrogen scale, and ‘Eg’ represents the bandgap energy. The calculated EVB and ECB values corresponding to BW0, BW2, BW4, and WS2 nanosheets are presented in Table 3.
Table 3 Calculated values of the energy band structure parameters of BW0, BW2, BW4 hybrid nanosheets, and WS2 nanosheets
Sample |
X (eV) |
Eg (eV) |
EVB (eV) vs. NHE |
ECB (eV) vs. NHE |
BW0 |
6.36 |
3.33 |
3.525 |
0.195 |
BW2 |
6.36 |
3.13 |
3.425 |
0.295 |
BW4 |
6.36 |
3.30 |
3.01 |
−0.29 |
WNS |
5.66 |
1.99 |
2.155 |
0.165 |
The schematic arrangement of the estimated band edge positions of these samples is presented in Fig. 7.
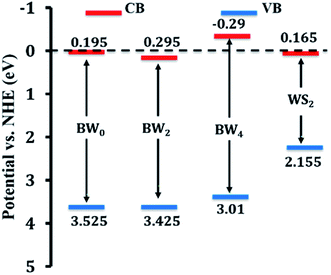 |
| Fig. 7 Schematic illustration of band edge positions of BW0, BW2, BW4 hybrid nanosheets and WS2 nanosheets. | |
3.3 Time-correlated single photon counting
The average lifetime of the excitons and dynamics of the photo-induced charge carriers was evaluated by Time-Correlated Single Photon Counting (TCSPC) study (Fig. 8).
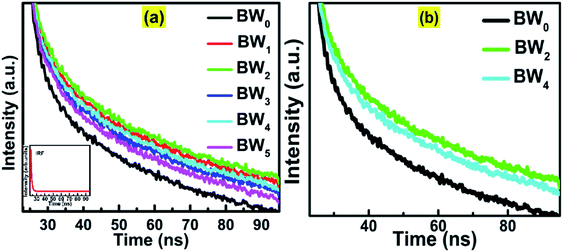 |
| Fig. 8 (a) Time-correlated single-photon counting (TCSPC) spectra of BWX hybrid nanosheets where X varies from 0% to 5%. Inset shows the IRF data. (b) Magnified TCSPC spectra for BW0, BW2 and BW4 nanosheets. | |
The decay curves of the hybrids, BWX (X varies from 0% to 5%), were simulated by using the double-exponential model.61
|
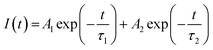 | (11) |
where
I(
t) represents the intensity of photoluminescence,
τ1 and
τ2 represent the decay times corresponding to slower and faster decay processes, respectively, and
A1 and
A2 represent the amplitudes. The inter-band exciton recombination results in a fast PL decay process, however, the slow PL decay process is based on the electron–hole pairs recombine in an indirect mode.
62
For pure BiOCl nanosheets, the contribution of the fast decay process with a 6.5 ns lifetime (τ2), is 91.79% and slow decay process of 277.38 ns (τ1), contributes only 8.21%. This implies that almost 92% of the excited states are getting deactivated via non-radiative path. The incorporation of WS2 in BiOCl has a significant effect on the dynamics of the charge carriers. 1 wt% addition of WS2 slows down the decay process by increasing the contribution of τ1 from 8.2% to 22.36% along with the increase in both τ1 and τ2 at the cost of the contribution of τ2. As per Table 4, when the WS2 content was increased from 1 wt% to 2 wt%, there was a further increase in the contribution of τ1 from 22% to 26% and the fast, and the slow decay components were lengthened from 8.21 ns to 9.64 ns and 325.49 ns to 340.11 ns. This increment suggests the evolution of new radiative pathways which promote the transfer of a greater number of photoexcited electrons to BiOCl. Similar observations were reported in case of CdS–CdSnO3 composite.20 Further addition of WS2 (3 wt%) however shortened the time constant of both the fast and the slow decay components of BiOCl (6.95 ns and 285.41 ns, respectively). This depicted the trapping of charge carrier from WS2 with the addition of WS2 content beyond 3 wt% in BiOCl nanosheets. The excess of the charge carrier trapping boosts the electron–hole pair recombination in the nanostructures.53 At relatively higher WS2 content of 4 wt% and 5 wt%, electrons trapped at BiOCl were such abundant that it helped in the enhancement of recombination rate of the charge carriers across the BiOCl/WS2 interface. This outcome depicted that the content of WS2 beyond a certain optimum value might act as a booster for interfacial electron–hole pairing and the prolonged decay lifetime observed for BW2 demonstrated the improved charge transfer from WS2 to BiOCl.
Table 4 Simulated parameters of eqn (11) and the calculated average lifetime of the BWX hybrid nanosheets (X varies from 0% to 5%)
Sample |
Lifetime (ns) |
Relative intensities (%) |
Chi-squared |
τ1 |
τ2 |
f1 |
f2 |
χ2 |
BW0 |
277.3 |
6.5 |
8.2% |
91.8% |
1.167 |
BW1 |
325.5 |
8.9 |
22.4% |
78.6% |
1.152 |
BW2 |
340.1 |
9.6 |
26.1% |
73.9% |
1.21 |
BW3 |
285.4 |
7.0 |
11.6% |
88.4% |
1.144 |
BW4 |
326.2 |
9.3 |
24.2% |
75.8% |
1.130 |
BW5 |
328.4 |
9.5 |
23.9% |
76.1% |
1.236 |
3.4 Photocatalytic response
The as-synthesized BW0, BW2, and BW4 hybrid nanosheets were analyzed for their photocatalytic response by Malachite Green (MG) degradation and Cr(VI) ion photoreduction under visible light irradiation. Fig. 9(a) shows the photodegradation of Malachite Green (MG) over BW0, BW2, and BW4 hybrid nanosheets. As can be seen in Fig. 9(b), after 30 min irradiation in visible light, ∼94.6% of MG was degraded over BW2 catalysts, whereas only 48.6% of MG was decomposed over BW0 and BW4 at the same time. Among all the BWX (X varies from 0% to 5%) samples, BW2 showed the highest photocatalytic response towards MG degradation. This was attributed to the incorporated WS2 nanosheets that helped in structural reorientation and bandgap narrowing. The structure reorientation facilitated the development of internal electric fields that helped in the separation of charge carriers and were beneficial for the charge transfer (revealed by X-ray diffractograms and TCSPC spectra). And bandgap narrowing contributed towards the visible-light-harvesting (confirmed by DRS).
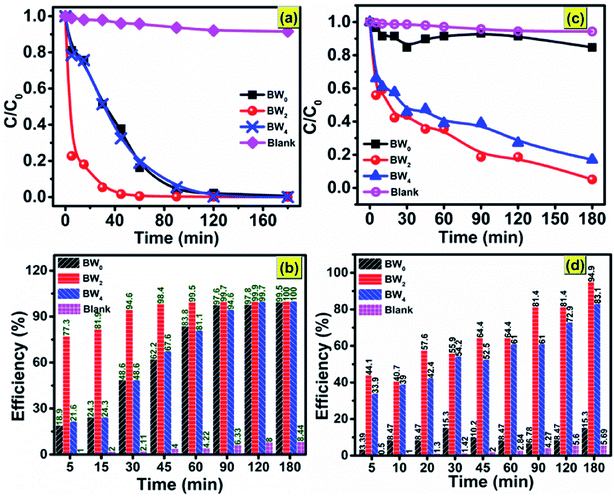 |
| Fig. 9 Photocatalytic degradation of (a) MG dye; (c) Cr(VI) without catalyst and in the presence of BW0, BW2, and BW4 under visible light irradiation; photocatalytic efficiency vs. time plot for (b) MG dye and (d) Cr(VI), over BW0, BW2, and BW4 hybrid nanosheets. | |
However, too much WS2 incorporation would result in decreasing the light absorption due to a lowering of the active sites as a result of agglomeration confirmed via FESEM images. Hence photocatalytic response got degraded upon increase the WS2 content beyond 2%. The degradation efficiency for MG reached ∼99.5% in 60 min, indicating the high photocatalytic response of BW2. The photocatalytic reduction of CrO3 solution using BW0, BW2, and BW4 hybrid nanosheets is shown in Fig. 9(c). It is demonstrated that the BW2 enabled the photoreduction of Cr(VI) up to ∼95% within 3 h of irradiation (Fig. 9(d)).
To date, only a few reports are present on the photocatalytic degradation of MG and Cr(VI) ions photocatalytic reduction using bismuth oxychloride-based catalysts under visible light irradiation. Some of these previous reports are mentioned in Table 5. Table 5 shows that the BiOCl/WS2 hybrid nanosheets prepared in the present work are better in the photocatalytic response than the existing bismuth oxychloride-based catalysts. The papers reporting more than 90% degradation efficiency have used a higher photocatalyst dose for a comparatively lower amount of dye to be degraded. The irradiation time taken for the photodegradation is also much higher than the irradiation time taken in the present work. Degradation efficiency ∼98.5% in only 45 min and more than 80% in 90 min was achieved in case of 10 mg l−1 of MG and Cr(VI) ions respectively, over only 0.5 g l−1 of BWX hybrid nanosheets catalysts dosage. This result indicates that the 2D/2D BWX hybrid nanosheet can be applied as an efficient photocatalyst for the degradation of complex and toxic organic dyes and heavy metal ion pollutants that are extremely hard to degrade.
Table 5 Previous reports on photocatalytic degradation of MG and Cr(VI) using BiOCl based materials
Catalyst |
Dye conc. (mg l−1) |
Dose (g l−1) |
Irradiation time (min) |
Dyes |
Efficiency |
Reference |
BiOCl |
25 |
0.7 |
120 |
MG |
65% |
63 |
Sn:BiOCl |
10 |
— |
240 |
MG |
98% |
64 |
Mn:BiOCl |
25 |
0.7 |
480 |
MG |
97% |
40 |
Bi (Bi2S3)/BiOCl |
40 |
20 |
40 |
MG |
97.4% |
65 |
BiOCl0.5Br0.5 |
10 |
— |
100 |
MG |
95% |
66 |
BiOCl/Fe2O3 |
10 |
10 |
300 |
Cr(VI) |
50% |
67 |
BiOCl |
30 |
— |
12 |
Cr(VI) |
100% |
68 |
g-BN/BiOCl |
10 |
0.8 |
150 |
Cr(VI) |
90% |
69 |
Ag/BiOCl |
— |
— |
180 |
Cr(VI) |
— |
70 |
BiOCl/WS2 |
10 |
0.5 |
45 |
MG |
98.4% |
Present work |
10 |
0.5 |
120 |
Cr(VI) |
94.9% |
In general, the experimental data of photocatalytic degradation is simulated by using the pseudo-first and second-order kinetic equations stated as follows:71
Pseudo-first-order kinetic equation,
|
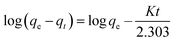 | (12) |
Pseudo-second-order kinetic equation,
|
 | (13) |
where
qe (mg g
−1) represents the amounts of BW
X adsorbed at equilibrium and
qt (mg g
−1) represents the amounts of BW
X adsorbed at time
t.
K (min
−1) and
k′ (g (mg
−1 min
−1)) represent the rate constants corresponding to pseudo-first and second-order adsorption, respectively.
Fig. 10(a,c) and (b,d) and show the plots of experimental data for MG and Cr(VI), based on eqn (12) and (13), respectively. Table 6 (represents the absorption parameters as per eqn (12) and (13)) shows that the regression coefficient values (R2) of eqn (12) and (13) are greater than 0.90. Hence, both the models are being followed in case of adsorption of MG over BWX hybrid nanosheets. Besides, in the case of Cr(VI) ions photoreduction, the R2 values (>0.90) of eqn (13) are greater than the R2 values (<0.90) of eqn (12). Hence, we can infer that the adsorption of Cr(VI) ions over BWX hybrid nanosheets follows only the second-order equation.
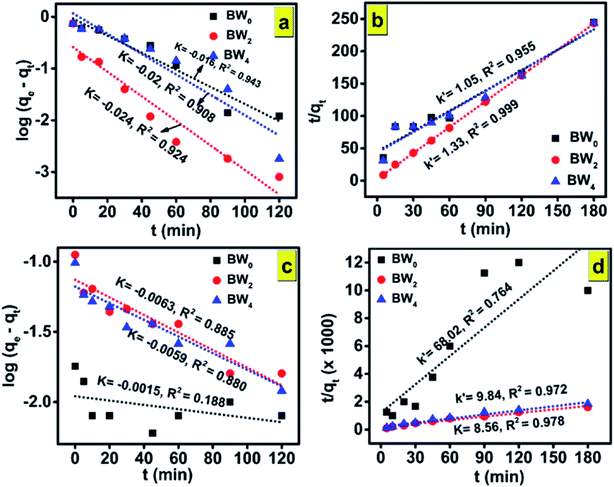 |
| Fig. 10 The adsorption mechanism model of (a and b) organic dye MG and (c and d) heavy metal ion Cr(VI) over BW0, BW2, and BW4 hybrid nanosheets. (a and c) Corresponds to the pseudo-first-order kinetics model; (b and d) corresponds to the pseudo-second-order kinetics model. | |
Table 6 Parameters of eqn (12) and (13) determined by the adsorption of MG and reduction of Cr(VI) onto the photocatalysts BW0, BW2, and BW4 hybrid nanosheets under visible light irradiation
Sample |
Malachite Green (MG) |
Chromium ion (Cr(VI)) |
First order |
Second order |
First order |
Second order |
K (min−1) |
R2 |
k′ (g (mg−1 min−1)) |
R2 |
K (min−1) |
R2 |
k′ (g (mg−1 min−1)) |
R2 |
BW0 |
−0.016 |
0.943 |
1.05 |
0.955 |
−0.002 |
0.188 |
68.02 |
0.764 |
BW2 |
−0.024 |
0.924 |
1.33 |
0.999 |
−0.006 |
0.885 |
8.56 |
0.978 |
BW4 |
−0.02 |
0.908 |
1.05 |
0.955 |
−0.006 |
0.880 |
9.84 |
0.972 |
Four consecutive tests of photodegradation of MG on BW2 were run to check the stability of the BW2 sample. Fig. 11(a) revealed only 10–20% decrease in photocatalytic performance over the consecutive runs. Hence the BW2 exhibit satisfying chemical stability.
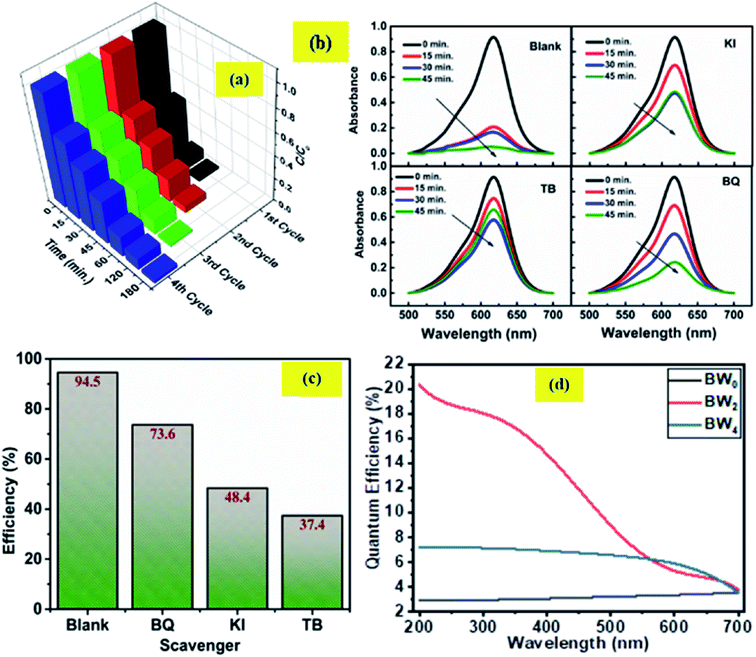 |
| Fig. 11 (a) Cyclic tests of photodegradation of MG on BW2 hybrid nanosheets. (b) Absorption spectra of MG dye with BW2 photocatalyst in the presence of various scavengers (BQ, TB, and KI). (c) Effect of various scavengers in the photodegradation process of MG over BW2 nanosheet hybrid and (d) IPCE spectra of BW0, BW2, and BW4 hybrid nanosheets recorded at 0 V vs. Ag/AgCl in the wavelength range 200–700 nm. | |
3.5 Active species trapping experiment
Generally, the photo-induced charge carriers i.e. electrons and holes are involved in the photocatalytic process. They get involved in the reaction with H2O/HO− and O2 and form the superoxide radicals ·OH and ·O2− as a product. These active species are fundamentally important in the photocatalytic reactions. In the intention of investigating the active species involved in the photodegradation process of MG using BWX hybrid nanosheets, a series of experiments were conducted for trapping the active species in the case of BW2 nanosheet hybrid. The different scavengers for different active species viz. tetra-butanol (TB) for ·OH, 1,4-benzoquinone (BQ) for ·O2−, and potassium iodide (KI) for both ·OH and h+ were used in the trapping experiments.72 Fig. 11(b and c) shows the effect of TB, BQ, and KI on the percentage of photodegradation of MG. The addition of the BQ in MG solution had a little effect on the C/C0, indicating that ·O2− is the secondary species and not the primary active species during the photocatalytic reaction. On the contrary, the addition of the KI and TB used as ·OH and h+ scavenger made a considerable impact on the conversion efficiency. The C/C0 for degradation of MG noticeably decreased after the addition of TB and KI. Therefore, it was established that h+ and ·OH have a substantial role in MG degradation. These observations propose that photogenerated ·OH is a dominating player in the MG photocatalytic degradation.
3.6 Incident photon to converted electron (IPCE) study
The relationship between the photoactivity improvement and the wavelength of the incident light, and the external quantum efficiency of the samples BW0, BW2, and BW4 were investigated by using Incident Photon to Converted Electron (IPCE) study. In this study, the photocurrent density at each incident wavelength was measured. The IPCE value for a given wavelength was calculated by using the equation |
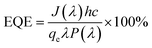 | (14) |
where J(λ) is the current density as a function of wavelength in A cm−2, h (Js) represents the Planck's constant, c (m s−1) is the velocity of light. qe represents the charge of the electron in C, λ (nm) represents the wavelength of incident light and P(λ) (W cm−2) represents the intensity of the power that depends on the wavelength of the incident light. Fig. 11(d) displays the IPCE spectra for BW0, BW2, and BW4 hybrid nanosheets. The IPCE values of follows the order: BW0 < BW4 < BW2, in the complete UV-visible spectrum. In particular, the IPCE values of the BW2 sample at the shorter wavelength region (<400 nm) were 10 times higher (18–20%) than the IPCE values of BW0 nanosheets. This established that the BW2 hybrid nanosheet converted the high energy incident photons to electrons well. It is well known that light-harvesting efficiency, charge separation, and collection yields73 play an important role in determining the IPCE value of a sample. Thus, we can further infer that the BW2 hybrid nanosheets had a larger light-harvesting efficiency and improved charge separation, which was in good agreement with its lower bandgap and slow decay time and better photocatalytic degradation results.
3.7 Photocatalytic mechanism
After being modified with WS2 nanosheets, the BiOCl/WS2 nanocomposite showed the higher optical absorption strength in the UV-visible range and larger dye absorption as compared to pure BiOCl nanosheets. The bandgap of BWX hybrid nanosheets was also found to be less than the bandgap of pure BiOCl nanosheets. The smaller bandgap of BWX hybrid nanosheets is responsible for higher visible light absorption. Due to the higher optical absorption of the BWX hybrid nanosheets, the surface adsorption of the dye was also improved. EVB and ECB corresponding to pure WS2 nanosheets are about 2.155 and 0.165 eV, respectively. And for pure BiOCl nanosheets, 3.525 and 0.195 eV, respectively. ECB and EVB corresponding to WS2 are at a higher position than the ECB and EVB corresponding to pure BiOCl, respectively. So, the electrons that are reaching in the conduction band of WS2 can easily transfer to the conduction band of BiOCl and the holes can easily move from BiOCl to the WS2 nanosheets (Fig. 12). As depicted by the scavenger test, the photogenerated ·OH radicals play the lead role in the MG photodegradation. The photocatalytic reactions that take place on the surface of BWX hybrid nanosheets can be described as follows:
BWX + hν → BW(X) (hvb+ + ecb−) |
O2 + BWX (ecb−) → ·O2− + BWX |
MG + hvb+ + ·OH → H2O + CO2 + other products. |
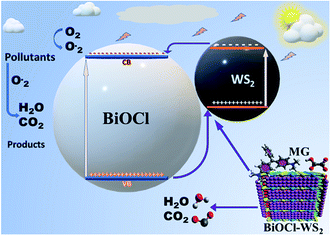 |
| Fig. 12 Schematic diagram of photocatalysis mechanism. | |
Photocatalytic activity is boosted with the increase in the charge carrier separation time, resulting in the hindrance to the charge carrier recombination. The time of the separation of charge carriers decides the number of formation of radicals that take part in the photodegradation of the pollutants.
4. Conclusion
In conclusion, a low-cost sonochemical route has been applied to synthesize a series of 2D/2D hybrid nanosheets of BiOCl/WS2 having high visible-light absorption. The preparation process was simplified efficiently along with reducing the preparation time. The successful fabrication of 2D/2D hybrid nanosheets of BiOCl/WS2 is confirmed through the various characterization techniques like XRD, Raman spectroscopy, FTIR spectroscopy, FESEM and EDX. The optical studies including band gap measurement, TCSPC and IPCE confirmed the higher visible light absorption and reduced decay rate of the charge carrier that causes the higher charge transfer rate in the hybrid nanosheets. The as synthesized BWX hybrid nanosheets exhibited an enhanced photocatalytic response than pure BiOCl nanosheets for MG photodegradation and Cr(VI) ion photoreduction. The BW2 sample achieved the highest photodegradation rate of more than 99% for MG photodegradation in 60 min under visible light irradiation. The major contributing factors in the enhancement of the photocatalytic performance of BWX are higher absorption of the visible range, higher rate of charge transfer, larger separation time of photogenerated charge carriers, and high IPCE values in the whole UV-visible spectrum. The photogenerated holes on WS2 nanosheets that form ·OH radicals, are found to be the primary active species in the photocatalytic process. The combination of high recyclability, high photocatalytic responsivity in visible light, and non-toxicity of the as-synthesized 2D/2D BWX hybrid nanosheet material pave the way for its usage in photocatalysis, and energy renovation applications. This study provides the scope of incorporation of 2D transition metal dichalcogenides in 2D BiOCl nanosheets to assist bandgap engineering and improve the rate of charge transfer for better photocatalytic activity.
Conflicts of interest
There are no conflicts to declare.
Acknowledgements
This work was supported by Science and Engineering Research Board vide [SERB (No. ECR/2017/001222)] and University Grants Commission vide [UGC (No. F.4–5(201 FRP)/2015(BSR))] to one of the authors (Dr Manika Khanuja). The support is highly acknowledged.
References
- P. R. Gogate and A. B. Pandit, A review of imperative technologies for wastewater treatment I: oxidation technologies at ambient conditions, Adv. Environ. Res., 2004, 8(3–4), 501–551 CrossRef CAS.
- J. Low, J. Yu and W. Ho, Graphene-Based Photocatalysts for CO2 Reduction to Solar Fuel, J. Phys. Chem. Lett., 2015, 6(21), 4244–4251 CrossRef CAS PubMed.
- Y. Zhang, G. Zhu, M. Hojamberdiev, J. Gao, J. Hao, J. Zhou and P. Liu, Synergistic effect of oxygen vacancy and nitrogen doping on enhancing the photocatalytic activity of Bi2O2CO3 nanosheets with exposed {001} facets for the degradation of organic pollutants, Appl. Surf. Sci., 2016, 371, 231–241 CrossRef CAS.
- X. Li, J. Yu and M. Jaroniec, Hierarchical photocatalysts, Chem. Soc. Rev., 2016, 45(9), 2603–2636 RSC.
- S. Wang, X. Yang, X. Zhang, X. Ding, Z. Yang, K. Dai and H. Chen, A plate-on-plate sandwiched Z-scheme heterojunction photocatalyst: BiOBr-Bi2MoO6 with enhanced photocatalytic performance, Appl. Surf. Sci., 2017, 391, 194–201 CrossRef CAS.
- L. Finegold and J. L. Cude, Biological sciences: one and two-dimensional structure of alpha-helix and beta-sheet forms of poly(L-alanine) shown by specific heat measurements at low temperatures (1.5–20 K), Nature, 1972, 238(5358), 38–40 CrossRef CAS PubMed.
- M. Wang, Y. S. Chang, C. W. Tsao, M. J. Fang, Y. J. Hsu and K. L. Choy, Enhanced photoelectrochemical hydrogen generation in neutral electrolyte using non-vacuum processed CIGS photocathodes with an earth-abundant cobalt sulfide catalyst, Chem. Commun., 2019, 55(17), 2465–2468 RSC.
- P. Y. Hsieh, Y. H. Chiu, T. H. Lai, M. J. Fang, Y. T. Wang and Y. J. Hsu, TiO2 nanowire-supported sulfide hybrid photocatalysts for durable solar hydrogen production, ACS Appl. Mater. Interfaces, 2019, 11(3), 3006–3015 CrossRef CAS PubMed.
- Y. S. Chang, M. Choi, M. Baek, P. Y. Hsieh, K. Yong and Y. J. Hsu, CdS/CdSe co-sensitized brookite H:TiO2 nanostructures: charge carrier dynamics and photoelectrochemical hydrogen generation, Appl. Catal., B, 2018, 225, 379–385 CrossRef CAS.
- H. Mittal and M. Khanuja, Nanosheets- and nanourchins-like nanostructures of MoSe2 for photocatalytic water purification: kinetics and reusability study, Environ. Sci. Pollut. Res., 2019 DOI:10.1007/s11356-019-06275-8.
- Y. H. Chiu and Y. J. Hsu, Au@Cu7S4 yolk@shell nanocrystal-decorated TiO2 nanowires as an all-day-active photocatalyst for environmental purification, Nano Energy, 2017, 31, 286–295 CrossRef CAS.
- I. Siddiqui, H. Mittal, V. K. Kohli, P. Gautam, M. Ali and M. Khanuja, Hydrothermally synthesized micron-sized, broom-shaped MoSe2 nanostructures for superior photocatalytic water purification, Mater. Res. Express, 2018, 5(12), 11–14 Search PubMed.
- C. N. Van, T. H. Do, J. W. Chen, W. Y. Tzeng, K. A. Tsai, H. Song, H. J. Liu, Y. C. Lin, Y. C. Chen, C. L. Wu, C. W. Luo, W. C. Chou, R. Huang, Y. J. Hsu and Y. H. Chu, WO3 mesocrystal-assisted photoelectrochemical activity of BiVO4, NPG Asia Mater., 2017, 9, 357 CrossRef.
- T. H. Do, C. N. Van, K. A. Tsai, L. T. Quynh, J. W. Chen, Y. C. Lin, Y. C. Chen, W. C. Chou, C. L. Wu, Y. J. Hsu and Y. H. Chu, Superior photoelectrochemical activity of self-assembled NiWO4–WO3 heteroepitaxy, Nano Energy, 2016, 23, 153–160 CrossRef CAS.
- Y. H. Chiu, T. F. M. Chang, C. Y. Chen, M. Sone and Y. J. Hsu, Mechanistic insights into photodegradation of organic dyes using heterostructure photocatalysts, Catalysts, 2019, 9(5), 430 CrossRef CAS.
- Y. H. Chiu, T. H. Lai, M. Y. Kuo, P. Y. Hsieh and Y. J. Hsu, Photoelectrochemical cells for solar hydrogen production: challenges and opportunities, APL Mater., 2019, 7, 080901 CrossRef.
- M. J. Fang, C. W. Tsao and Y. J. Hsu, Semiconductor nanoheterostructures for photoconversion applications, J. Phys. D: Appl. Phys., 2020, 53(14), 143001 CrossRef CAS.
- K. A. Tsai and Y. J. Hsu, Graphene quantum dots mediated charge transfer of CdSe nanocrystals for enhancing photoelectrochemical hydrogen production, Appl. Catal., B, 2015, 164, 271–278 CrossRef CAS.
- M. Y. Chena and Y. J. Hsu, Type-II nanorod heterostructure formation through one-step cation exchange, Nanoscale, 2013, 5, 363–368 RSC.
- Y. F. Lin and Y. J. Hsu, Interfacial charge carrier dynamics of type-II semiconductor nanoheterostructures, Appl. Catal., B, 2013, 130–131, 93–98 CrossRef CAS.
- Y. C. Chen, Y. C. Pu and Y. J. Hsu, Interfacial Charge Carrier Dynamics of the three-component In2O3–TiO2–Pt heterojunction system, J. Phys. Chem. C, 2012, 116, 2967–2975 CrossRef CAS.
- S. Saha, N. Chaudhary, H. Mittal, G. Gupta and M. Khanuja, Inorganic–organic nanohybrid of MoS2-PANI for advanced photocatalytic application, Int. Nano Lett., 2019, 9(2), 127–139 CrossRef CAS.
- R. Sharma, M. Khanuja, S. S. Islam, U. Singhal and A. Varma, Aspect-ratio-dependent photoinduced antimicrobial and photocatalytic organic pollutant degradation efficiency of ZnO nanorods, Res. Chem. Intermed., 2017, 43(10), 5345–5364 CrossRef CAS.
- T. K. Sen and S. Dawood, Review on Dye Removal from Its Aqueous Solution into Alternative Cost-Effective and Non-Conventional Adsorbents, J. Chem. Process Eng. Res., 2013, 1(104), 1–11 Search PubMed.
- H. Mittal, A. Kumar and M. Khanuja, In-situ oxidative polymerization of aniline on hydrothermally synthesized MoSe2 for enhanced photocatalytic degradation of organic dyes, J. Saudi Chem. Soc., 2019, 23(7), 836–845 CrossRef CAS.
- N. P. Raval, P. U. Shah and N. K. Shah, Malachite green ‘a cationic dye’ and its removal from aqueous solution by adsorption, Appl. Water Sci., 2017, 7(7), 3407–3445 CrossRef CAS.
- D. R. Doerge, M. I. Churchwell, T. A. Gehring, Y. M. Pu and S. M. Plakas, Analysis of malachite green and metabolites in fish using liquid chromatography atmospheric pressure chemical ionization mass spectrometry, Rapid Commun. Mass Spectrom., 1998, 12(21), 1625–1634 CrossRef CAS.
- H. Wang, X. Yuan, Y. Wu, G. Zeng, X. Chen, L. Leng, Z. Wu, L. Jiang and H. Li, Facile synthesis of amino-functionalized titanium metal-organic frameworks and their superior visible-light photocatalytic activity for Cr(VI) reduction, J. Hazard. Mater., 2015, 286, 187–194 CrossRef CAS PubMed.
- M. A. Barakat, New trends in removing heavy metals from industrial wastewater, Arabian J. Chem., 2011, 4(4), 361–377 CrossRef CAS.
- A. C. Sophia, D. Catherine and V. M. Bhalambaal, Utilization of rice-husk and coconut shell carbons for water disinfection, J. Environ. Sci. Eng., 2013, 55(1), 9–16 CAS.
- S. Singh, R. Sharma and M. Khanuja, A review and recent developments on strategies to improve the photocatalytic elimination of organic dye pollutants by BiOX (X = Cl, Br, I, F) nanostructures, Korean J. Chem. Eng., 2018, 35(10), 1955–1968 CrossRef CAS.
- X. Li, J. Xie, C. Jiang, J. Yu and P. Zhang, Review on design and evaluation of environmental photocatalysts, Front. Environ. Sci. Eng., 2018, 12(5), 1–32 CrossRef CAS.
- Y. Yang, C. Zhang, C. Lai, G. Zeng, D. Huang, M. Cheng, J. Wang, F. Chen, C. Zhou and W. Xiong, BiOX (X = Cl, Br, I) photocatalytic nanomaterials: applications for fuels and environmental management, Adv. Colloid Interface Sci., 2018, 254, 76–93 CrossRef CAS PubMed.
- M. Sun, Q. Zhao, C. Du and Z. Liu, Enhanced visible-light photocatalytic activity in BiOCl/SnO2: heterojunction of two wide band-gap semiconductors, RSC Adv., 2015, 5(29), 22740–22752 RSC.
- M. A. Gondal, C. Xiaofeng, and M. A. Dastageer, Novel Bismuth-Oxyhalide-Based Materials and their Applications, Springer India, Dhahran, Saudi Arabia, 1st edn, 2017, vol. 76 Search PubMed.
- J. Jiang, K. Zhao, X. Xiao and L. Zhang, Synthesis and facet-dependent photoreactivity of BiOCl single-crystalline nanosheets, J. Am. Chem. Soc., 2012, 134(10), 4473–4476 CrossRef CAS PubMed.
- H. Gnayem and Y. Sasson, Hierarchical nanostructured 3D flowerlike BiOClxBr1−x semiconductors with exceptional visible light photocatalytic activity, ACS Catal., 2013, 3(2), 186–191 CrossRef CAS.
- G. Li, B. Jiang, S. Xiao, Z. Lian, D. Zhang, J. C. Yu and H. Li, An efficient dye-sensitized BiOCl photocatalyst for air and water purification under visible light irradiation, Environ. Sci.: Processes Impacts, 2014, 16(8), 1975–1980 RSC.
- S. Weng, B. Chen, L. Xie, Z. Zheng and P. Liu, Facile in situ synthesis of a Bi/BiOCl nanocomposite with high photocatalytic activity, J. Mater. Chem. A, 2013, 1(9), 3068–3075 RSC.
- B. Pare, B. Sarwan and S. B. Jonnalagadda, Photocatalytic mineralization study of malachite green on the surface of Mn-doped BiOCl activated by visible light under ambient condition, Appl. Surf. Sci., 2011, 258(1), 247–253 CrossRef CAS.
- N. Tahmasebi, Z. Maleki and P. Farahnak, Enhanced photocatalytic activities of Bi2WO6/BiOCl composite synthesized by one-step hydrothermal method with the assistance of HCl, Mater. Sci. Semicond. Process., 2019, 89, 32–40 CrossRef CAS.
- F. Li, Y. Li, M. Chai, B. Li, Y. Hao, X. Wang and R. Liu, One-step construction of {001} facets-exposed BiOCl hybridized with Al2O3 for enhanced molecular oxygen activation, Catal. Sci. Technol., 2016, 6(22), 7985–7995 RSC.
- Y. Shi, X. Xiong, S. Ding, X. Liu, Q. Jiang and J. Hu, In situ topotactic synthesis and photocatalytic activity of plate-like BiOCl/2D networks Bi2S3 heterostructures, Appl. Catal., B, 2018, 220, 570–580 CrossRef CAS.
- K. Wang, C. Shao, X. Li, X. Zhang, N. Lu, F. Miao and Y. Liu, Hierarchical heterostructures of p-type BiOCl nanosheets on electrospun n-type TiO2 nanofibers with enhanced photocatalytic activity, Catal. Commun., 2015, 67, 6–10 CrossRef CAS.
- Q. Li, X. Zhao, J. Yang, C. J. Jia, Z. Jin and W. Fan, Exploring the effects of nanocrystal facet orientations in g-C3N4/BiOCl heterostructures on photocatalytic performance, Nanoscale, 2015, 7(45), 18971–18983 RSC.
- F. Dong, Y. Sun, M. Fu, Z. Wu and S. C. Lee, Room temperature synthesis and highly enhanced visible-light photocatalytic activity of porous BiOI/BiOCl composites nanoplates micro flowers, J. Hazard. Mater., 2012, 219–220, 26–34 CrossRef CAS PubMed.
- B. Liu, W. Xu, T. Sun, M. Chen, L. Tian and J. Wang, Efficient visible-light photocatalytic activity of CdS on (001) facets exposed to BiOCl, New J. Chem., 2014, 38(6), 2273–2277 RSC.
- S. Shamaila, A. K. L. Sajjad, F. Chen and J. Zhang, WO3/BiOCl, a novel heterojunction as a visible light photocatalyst, J. Colloid Interface Sci., 2011, 356(2), 465–472 CrossRef CAS PubMed.
- P. Xiao, J. Lou, H. Zhang, W. Song, X. L. Wu, H. Lin, J. Chen, S. Liu and X. Wang, Enhanced visible-light-driven photocatalysis from WS2 quantum dots coupled to BiOCl nanosheets: Synergistic effect and mechanism insight, Catal. Sci. Technol., 2018, 8(1), 201–209 RSC.
- N. Chaudhary and M. Khanuja, Architectural design of photodetector based on 2D(MoS2 nanosheets)/1D(WS2 nanorods) heterostructure synthesized by facile hydrothermal method, J. Electrochem. Soc., 2019, 166(14), 1–11 CrossRef.
- W. Ashraf, T. Fatima, K. Srivastava and M. Khanuja, Superior photocatalytic activity of tungsten disulfide nanostructures: role of morphology and defects, Appl. Nanosci., 2019, 9(7), 1515–1529 CrossRef CAS.
- Y. Lei, G. Wang, S. Song and H. Zhang, Synthesis, characterization, and assembly of BiOCl nanostructure and their photocatalytic properties, CrystEngComm, 2009, 11(9), 1857–1862 RSC.
- J. Chen, M. Guan, W. Cai, J. Guo, C. Xiao and G. Zhang, The dominant (001) facet-dependent enhanced visible-light photoactivity of ultrathin BiOBr nanosheets, Phys. Chem. Chem. Phys., 2014, 16(38), 20909–20914 RSC.
- Z. Jia, W. Chen, T. Liu, T. Huang and X. Liu, Biomolecule-assisted solvothermal synthesis and enhanced visible-light photocatalytic performance of Bi2S3/BiOCl composites, J. Wuhan Univ. Technol., Mater. Sci. Ed., 2016, 31(4), 765–772 CrossRef CAS.
- J. Di, J. Xia, M. Ji, L. Xu, S. Yin, Q. Zhang, Z. Chen and H. Li, Carbon quantum dots in situ coupling to bismuth oxyiodide via reactable ionic liquid with enhanced photocatalytic molecular oxygen activation performance, Carbon, 2016, 98, 613–623 CrossRef CAS.
- C. Tan, G. Zhu, M. Hojamberdiev, K. Okada, J. Liang, X. Luo, P. Liu and Y. Liu, Co3O4 nanoparticles-loaded BiOCl nanoplates with the dominant {001} facets: efficient photodegradation of organic dyes under visible light, Appl. Catal., B, 2014, 152–153(1), 425–436 CrossRef CAS.
- L. Shan, J. Bi and Y. Liu, Roles of BiOCl (001) in face-to-faced BiOI(010)/BiOCl(001) heterojunction, J. Nanopart. Res., 2018, 20, 170 CrossRef.
- J. Xia, L. Xu, J. Zhang, S. Yin, H. Li, H. Xu and J. Dia, Improved visible-light photocatalytic properties of Fe/BiOCl microspheres synthesized via self-doped reactable ionic liquids, CrystEngComm, 2013, 15(46), 10132–10141 RSC.
- S. Singh, A. Ruhela, S. Rani, M. Khanuja and R. Sharma, Concentration specific and tunable photoresponse of bismuth vanadate functionalized hexagonal ZnO nanocrystals based photoanodes for photoelectrochemical application, Solid State Sci., 2018, 76, 48–56 CrossRef CAS.
- S. Ebraheem and A. El-Saied, Bandgap determination from diffuse reflectance measurements of irradiated lead borate glass system doped with TiO2 by using diffuse reflectance technique, Mater. Sci. Appl., 2013, 4(5), 324–329 Search PubMed.
- F. Li, Y. Li, M. Chai, B. Li, Y. Hao, X. Wang and R. Liu, One-step construction of {001} facet-exposed BiOCl hybridized with Al2O3 for enhanced molecular oxygen activation, Catal. Sci. Technol., 2016, 6(22), 7985–7995 RSC.
- H. Zhang, R. Zong and Y. Zhu, Photocorrosion inhibition and photoactivity enhancement for zinc oxide via hybridization with monolayer polyaniline, J. Phys. Chem. C, 2009, 113(11), 4605–4611 CrossRef CAS.
- B. Sarwan, A. D. Acharya and B. Pare, Visible light-driven photocatalytic degradation and mineralization of the malachite green dye in a slurry photoreactor, Part. Sci. Technol., 2017, 35(4), 472–478 CrossRef CAS.
- X. Han, S. Dong, C. Yu, Y. Wang, K. Yang and J. Sun, Controllable synthesis of Sn-doped BiOCl for efficient photocatalytic degradation of mixed-dye wastewater under natural sunlight irradiation, J. Alloys Compd., 2016, 685, 997–1007 CrossRef CAS.
- Z. Shen, Q. Han, W. Liu, X. Wang and J. Zhu, One-step synthesis of Bi(Bi2S3)/BiOCl heterojunctions by a simple solid-state milling method and their visible light photoreactivity, J. Mater. Sci., 2019, 54(1), 613–624 CAS.
- X. Jia, Q. Han, X. Wang and J. Zhu, Milling-induced synthesis of BiOCl1−xBrx solid solution and their adsorptive and photocatalytic performance, Photochem. Photobiol., 2018, 94(5), 942–954 CrossRef CAS PubMed.
- K. Li, Y. Liang, J. Yang, H. Zhang, G. Yang and W. Lei, BiOCl/Fe2O3 heterojunction nanoplates with the enhanced visible-light-driven photocatalytic performance for degrading organic pollutants and reducing Cr(VI), J. Photochem. Photobiol., A, 2018, 364, 240–249 CrossRef CAS.
- Y. Peng, Y. G. Mao, P. F. Kan, J. Y. Liu and Z. Fang, Controllable synthesis and photoreduction performance towards Cr(VI) of BiOCl micro rods with exposed (110) crystal facets, New J. Chem., 2018, 42(20), 16911–16918 RSC.
- H. Xu, Z. Wu, M. Ding and X. Gao, Microwave-assisted synthesis of flower-like BN/BiOCl composites for photocatalytic Cr(VI) reduction upon visible-light irradiation, Mater. Des., 2017, 114, 129–138 CrossRef CAS.
- H. Li and L. Zhang, Oxygen vacancy induced selective silver deposition on the {001} facets of BiOCl single-crystalline nanosheets for enhanced Cr(VI) and sodium pentachlorophenol removal under visible light, Nanoscale, 2014, 6(14), 7805–7810 RSC.
- R. Arasteh, M. Masoumi, A. M. Rashidi, L. Moradi, V. Samimi and S. T. Mostafavi, Adsorption of 2-nitrophenol by multi-wall carbon nanotubes from aqueous solutions, Appl. Surf. Sci., 2010, 256(14), 4447–4455 CrossRef CAS.
- X. V. Doorslaer, P. M. Heynderickx, K. Demeestere, K. Debevere, H. V. Langenhove and J. Dewulf, TiO2 mediated heterogeneous photocatalytic degradation of moxifloxacin: operational variables and scavenger study, Appl. Catal., B, 2012, 111–112, 150–156 CrossRef.
- J. T. Kirner and R. G. Finke, Water-oxidation photoanodes using organic light-harvesting materials: a review, J. Mater. Chem. A, 2017, 5, 19560–19592 RSC.
|
This journal is © The Royal Society of Chemistry 2020 |
Click here to see how this site uses Cookies. View our privacy policy here.