DOI:
10.1039/D0RA03668D
(Paper)
RSC Adv., 2020,
10, 24073-24078
An indirect analytical approach based on ATR-FTIR spectroscopy for determining the FFA content in vegetable oils
Received
24th April 2020
, Accepted 31st May 2020
First published on 24th June 2020
Abstract
In this study, we developed a novel approach for determining a free fatty acid (FFA) in vegetable oils using attenuated total reflection Fourier transform infrared (ATR-FTIR) spectroscopy. FFA was converted to carboxylate species by a reaction with phthalimide potassium salt, and the linear relationship between FFA content and ATR-FTIR peak areas at 1541–1616 cm−1 (1595 cm−1 as baseline) was established. Results showed that the R2 values obtained during calibration and validation were more than 0.99. The calibration method concurred to within ±0.035% over the range of 0.4% to 4.0% (quantitative determination of the percentage of FFA in oils, expressed as the percentage of oleic acid). In the calibration model, the root mean square error of prediction was 0.0104, the relative error was less than 0.246% and the relative average deviation was 0.386%, respectively. These indexes demonstrated that the calibration model has great accuracy, high precision and good stability. The indirect method established using ATR-FTIR has the advantages of excellent reproducibility, high exactitude, independent of oil type, simple operation and easy cleaning of the instrument surface. The slope of the verification equation between FFA prediction values and American Oil Chemists' Society's (AOCS) titration method was close to 1, R2 value was more than 0.99. These indicators suggested that the proposed method and the AOCS method have a good correlation through AOCS titration and ATR–FTIR spectroscopy to determine validation samples parallel. In addition, for comparison, when the AOCS titration and ATR-FTIR spectroscopy methods were used for sample validation, the results indicated that the latter method is more reproducible, highly sensitive and has strong anti-disturbance. Therefore, the ATR-FTIR technique can be applied as a simple, highly sensitive, convenient and timely method for the analysis of FFAs in oils.
Introduction
The free fatty acid (FFA) content is regarded as a significant and remarkable index for assessing the quality of oils. The main reason for the presence of FFAs is the hydrolytic rancidity of oils. Active lipolytic enzymes promote the decomposition of triglycerides to produce FFAs in the presence of water during vegetable oil processing.1 When oil storage conditions are improper (high temperature and exposure to light and air) or the storage time is prolonged, oils undergo oxidation rancidity, which increases the FFA content.2 FFAs are usually subjected to strict supervision because they produce undesirable effects, such as reduced shelf life, decreased product nutritional values, and can harm the human intestinal tract.3,4 Hence, measuring the FFA content is important during vegetable oil processing. The most extensively used traditional method for FFA is the chemical titration method of the American Oil Chemists' Society (AOCS). Although the AOCS method is reliable and widely used, it is generally laborious and requires abundant environmentally toxic chemical reagents and solvents, and the end point is inaccurate for the detection of dark oils.5,6
A number of methods, such as pH-metric, chromatographic, and spectroscopic methods, have been used to detect the FFA content in vegetable oils. These techniques have been extensively used as significant tools in vegetable oils. In the absence of a chemical laboratory, the method for determining FFA in vegetable oils is a pH-metric technique that uses triethanolammonium salts, triethanolamine, or 1,2-dichloroethane.7–10 Based on chromatographic techniques, some robust methods for resolving FFA in rice bran oil, corn oil, sesame oil and soybean oil were successfully developed.6,11–14 Another high-speed colorimetry method was developed to determine FFA in oils with synthetic polyamidoamine dendrimers and citrate-capped gold nanoparticles.3 A number of spectroscopic methods, such as UV-vis, H-1-nuclear magnetic resonance, and molecular spectroscopy, are used in the detection of FFA in vegetable oils.15,16 These methods are precise and widely reported but are normally complicated requiring tedious pretreatments and are time-consuming. The Fourier transform infrared (FTIR) spectroscopy technique is a detection technology that has rapidly developed in recent years. FTIR spectroscopy can help obtain effective molecular structures and component information by the in situ noninvasive analysis of samples.17 It is a highly efficient, fast, nondestructive, and green method and can simultaneously determine several different components.1,18
Numerous FTIR methods are used for the analysis of FFAs in oils and these methods can be categorized into direct and indirect methods based on different principles. Direct methods directly measure infrared absorption at 1711 cm−1, which is related to the C
O vibration of the carboxylic group. Clear and significant FTIR methods based on direct methods have been used in analyzing FFAs in frying palm oil, rapeseed oil, coconut oil, and soybean oil.19–25 Although these methods are effective in determining FFAs, the peak at 1711 cm−1, which corresponds to the C
O vibration in carboxyl, is normally located at the shoulder of the very strong C
O peak at 1746 cm−1 of triacylglycerol ester. The peak at 1711 cm−1 only becomes a resolution peak at high contents of FFA. The extent of the overlap of the peaks depends on oils and influences the accurate quantification of the FFA content. Indirect methods involve measuring a new peak at 1570 cm−1, which is interrelated with the C
O group of the carboxylic acid radical formed by the addition of carboxylate to oils. The method successfully prevented the influence of the C
O group in the triacylglycerol ester. Sodium carbodiimide, potassium phthalimide, and KOH methanol are often used.23,26,27 These methods prevented various sample effects and demonstrated high precision. However, the complexity of the sample processing, inconvenience, and the high cost of liquid cell washing limited its further utilization. Even though these issues can be resolved using a specially designed flow-through transmission cell unit, the accessory has not been used commercially. As a rapid detection technology based on the principle of optical internal reflection, attenuated total reflection (ATR) has the characteristics of simple sample preparation and convenient handling.28 An ATR-FTIR method combined with an indirect method to measure the ν(COO−) band formed by the reaction of FFA with alkali salt overcomes the limitation imposed by the less-accurate direct methods.
The purpose of this study was to develop a simple and less sample consuming method for the FFA measurement in oils. The method is based on the ATR-FTIR technique combined with an indirect method. The key point of indirect methods is the choice of a reaction reagent. A reaction reagent too weak to completely react with FFA, as saponification of vegetable oils can occur when strong alkali salt such as KOH is used, which interferes with the accurate determination of the FFA content.24 Phthalimide potassium salt satisfies this criterion. When excessive amounts of phthalimide potassium salt were used, the reaction was accurate and complete. The effectiveness of the proposed method was evaluated using the AOCS method.
Materials and methods
Materials and reagents
A variety of vegetable oils were purchased from a local supermarket and stored in plastic bottles at 4 °C from 1 month to 1 year. Soybean oil was used as the basic oil for sample calibration and validation. Nine vegetable oil samples (soybean oil, flaxseed oil, coconut oil, cottonseed oil, peanut oil, rapeseed oil, corn oil, rice bran oil, and camellia oil) were used in the blind validation set. When FFA-free soybean oil samples were required, FFA was removed by using a column of microwave-activated silica gel (3 cm in diameter and 28 cm in length) and validated by the AOCS method. All reagents and chemicals used were of analytical grade. Isopropyl alcohol, diethyl ether, and hexanoic acid were supplied by Guangdong Guanghua Sci-Tech Co (Guangdong, Guanghua, China). Phthalimide potassium salt was purchased from Shanghai Macklin Biochemical Co (Shanghai, China). Potassium hydroxide (KOH) was purchased from Chengdu of the Industrial Development Zone, Xindu Mulan (Chengdu, China).
Preparation of calibration and validation samples
Ten calibration standards (0.4%, 0.5%, 0.6%, 1.0%, 1.5%, 2.0%, 2.5%, 3.0%, 3.5%, and 4.0%) covered the range of 0.0–4.0% FFA content in the samples (expressed as the percentage of oleic acid), and sixteen (0.3%, 0.5%, 0.65%, 0.85%, 1.0%, 1.2%, 1.5%, 2.0%, 2.3%, 2.5%, 2.7%, 3.0%, 3.3%, 3.5%, 3.7% and 4.0%) validation standards [relative proportion of FFA oil (Rp)] were prepared by adding hexanoic acid to FFA-free samples through gravimetric method. Subsequently, a series of FFA calibration standards were used to develop ATR-FTIR calibration (section Analytical protocol). Validation samples were prepared by blending samples and validation standards to produce two validation sets.
Analytical protocol
All standards and blind samples (3 mL) were placed in 15 mL tubes, then 6 mL of phthalimide potassium salt/isopropyl alcohol (40 g L−1) was added to these samples. Samples were rapidly mixed with a vortex mixer for 60 s and centrifuged at 2368 × g for 10 min. Supernatants were reserved for the ATR-FTIR analysis. The reaction mechanism (Scheme 1) involves a reaction of the nucleophilic phthalimide potassium salt and FFA. FFA was converted to carboxylate, which was determined by the ATR-FTIR technique. Sublayer oil samples did not show the FFA content by the AOCS method.
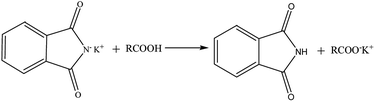 |
| Scheme 1 Schematic of the FFA being converted. | |
ATR-FTIR analysis
A Bruker Vertex 70 series FTIR spectrometer equipped with a diamond single-bounce ATR accessory was employed, and the spectra were obtained using the OPUS Program 7.2 from Bruker. The ATR-FTIR spectra of all samples were obtained by determining the average of 16 scans and recorded with a resolution of 4 cm−1 in the spectral range of 4000–600 cm−1. The air spectrum was collected as background at 25 °C and 20% relative humidity. Approximately 20 μL of the supernatant was directly added dropwise on the surface of ATR and washed with alcohol after each measurement. Samples from each concentration were prepared in triplicate, and the average spectra were used for calibration and validation analyses.
FFA determination
A calibration equation relating FFA to the peak areas at 1541–1616 cm−1 relative to the baseline at 1595 cm−1 in the spectra of the calibration standards (prepared as described in section Analytical protocol) was programmed in the Origin10.0 (Originlab, Northampton, MA) software. The calibration equation was used in processing the spectra of the validated samples, and the correlation was analyzed between the reported FFA results and the values obtained by the AOCS titration.
Statistical analysis
All analyses were performed in triplicate, and the results were expressed as mean values. Spectral data processing and statistical analysis were performed using OMNIC 7.3 (Thermo Electron Inc., Madison, WI) and Origin 10.0. R2 value, standard deviation (SD) revealed the accuracy of the fitting curves, and the root mean square error of prediction (RMSEP) was used to determine the fitting degree of the regression curve. The relative mean deviation (RD) and relative error (RE) were used to estimate the precision and accuracy of the calibration model, respectively. First-order coefficients and vertical intercepts were applied in the estimation of the accuracy between the present and AOCS methods.
Results and discussion
Spectral analysis
Fig. 1 shows the assignments of the absorption bands obtained using the ATR-FTIR technique in the region between 4000 cm−1 and 750 cm−1 in the calibration samples.
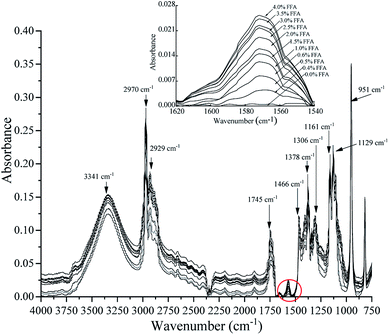 |
| Fig. 1 Spectral response at 4000–750 cm−1 was obtained after the addition of phthalimide potassium salt as the reactive reagent to the samples prepared using different hexanoic acid contents with FFA-free oil by the ATR-FTIR technique. | |
The band at 1571 cm−1 is associated with the ν(COO−) anti-symmetric stretching vibration. A test set of samples was prepared with different hexanoic acid contents with FFA-free oil and tested by the ATR-FTIR technique. The spectra of the samples showed an increase in the ν(COO−) band at 1571 cm−1 as FFA in the oil increased, indicating that FFA was converted to carboxylate by phthalimide potassium salt. Spectroscopic analysis was performed on the acquired ATR-FTIR data to further determine the bands obtained from the calibration samples found in the 4000–750 cm−1 region. In the FFA samples, the intensities of the rest spectral region had similar trends compared to the 1571 cm−1 band. The 951 cm−1 band was connected to the out-of-plane deformation vibration of carboxylic acid. The C–C and C–N vibrations were attributed to the bands at 1129 cm−1 and 1161 cm−1, respectively. The band at 1306 cm−1 can be attributed to the C–O telescopic vibration or the O–H deformation vibration of carboxylic acid. The band at 1378 cm−1 corresponded with the C–O symmetrical telescopic vibration of carboxylate. The bands observed at 1466 cm−1 and 2970 cm−1 can be attributed to –CH3. The band at 2929 cm−1 was related to –CH2, and that at 3341 cm−1 was attributed to the carboxylate group.
Calibration
The calibration standards consisted of FFA-free oil containing varying hexanoic acid contents, representing an FFA range of 0.0–4.0% content (% expressed as the percentage of oleic acid). Fig. 2 illustrates the standard curve of the FFA contents and the peak areas of the ν(COO−) band. Peak area was measured at 1541–1616 cm−1 relative to a baseline point at 1595 cm−1, which is parallel to the response of ν(COO−) band illustrated in Fig. 1. The following equation was used for the prediction of FFA content in the calibration set samples: |
FFA (%) = 13.338A(1541–1616/1595 cm−1) − 1.0073
| (1) |
where A represents the peak areas at 1541–1616 cm−1, and 1595 cm−1 is the baseline for the ATR-FTIR technique. R2 value and SD are statistical indicators pointing out the reliability of regression equation estimation. The FFA contents measured by the ATR-FTIR technique (R2 > 0.99, SD = 0.0359, RMSEP = 0.0104) were significantly correlated with peak areas of the ν(COO−) band. The detection limit was 0.4%, and the range of FFA was 0.4–4.0%. The linear regression equation indicated that FFA can be determined to within ±0.035% over the range of 0.4–4.0% in vegetable oils. The RE was less than 0.246%, and the RD value was 0.386%.
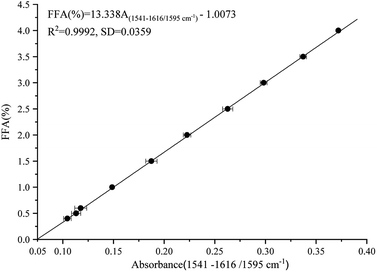 |
| Fig. 2 Calibration of FFA contents versus peak areas of FFA measured at 1616–1541 cm−1 and referenced to 1595 cm−1 as baseline, which was obtained from the spectra of soybean oil spiked with varying amounts of FFA. | |
Validation
Internal validation is an available and considerable method to estimate the prediction accuracy of the calibration by selecting a partial amount of the calibration samples to model the rejected samples and comparing the difference between the predicted values of the rejected samples and the actual values determined by internationally recognized approaches. Fig. 3 shows the relationship between FFA validation standard and FFA values determined by the ATR-FTIR technique [(predicted from eqn (1)] and the AOCS method, respectively. The corresponding regression equations are presented below: |
FFA(ATR-FTIR) (%) = 0.9977Rp + 0.0006
| (2) |
|
FFA(AOCS) (%) = 0.9202Rp + 0.0873
| (3) |
where Rp represents the relative proportion of FFA oil. Fig. 3 shows a similar trend of the two fitting curves, an increase in the measurement value of FFA as the FFA oil content in the validation standard increased. However, the ATR-FTIR equation (R2 = 0.9998, SD = 0.2543) had a higher R2 value and significantly lower SD value than the equation for the AOCS titration method (R2 = 0.9985, SD = 0.6958). The RMSEP of the ATR-FTIR method (0.0123) was less than that of the AOCS titration (0.0459) method. Therefore, the established calibration model (eqn (1)) has a better stability. As can be seen from eqn (3), the slope of eqn (3) was lower than that of eqn (2). When the content of FFA in oils was less than 1.0%, the volume measurement of the basic burette was not accurate in the AOCS method, which leads to large consumption of KOH in actual use.
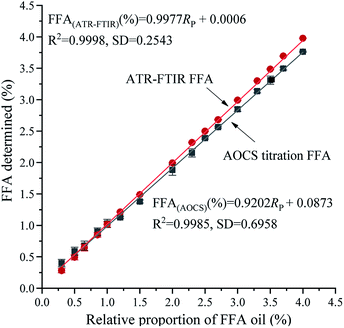 |
| Fig. 3 Graphical representation of the data obtained from duplicate analyses of a series of FFA validation standards by the ATR-FTIR technique and AOCS method. | |
The established method was estimated using the slope and vertical intercepts of the fitted regression line of the equation and R2 value of the equation. The equation was defined as y = x when the slope and vertical intercept were ascertained as 1 and 0, respectively, with 100% predictability. Consequently, the slope and vertical intercepts were significant parameters to support the rationality of the model.
The R2 value was greater than 0.99 and SD was equal to 0.2543 in eqn (2). The slope of the relationship was determined to be 0.9977 (approaches to 1), and the vertical intercept was 0.0006 (approximately equal to 0). The model equation established by the relationship between FFA validation standard and the FFA value measured by ATR-FTIR technology was extremely analogous to y = x, which verifies the rationality of the model.
When comparing the results obtained by the ATR-FTIR technique and AOCS method, the following linear regression equation was obtained:
|
FFA(ATR-FTIR) (%) = 1.0824 FFA(AOCS) (%) − 0.0906
| (4) |
The following equation was obtained from the forced regression equation of origin:
|
FFA(ATR-FTIR) (%) = 1.0475 FFA(AOCS) (%)
| (5) |
The R2 value was more than 0.99, and the SD value was determined to be 0.4097 in eqn (5). The slope of the equation was determined to be 1.0475, which indicates the relationship between the ATR-FTIR method and AOCS procedure was analogous to y = x. The validation methods concur to within ±0.41%, most of the variability in the relationship was due to the AOCS titration method (volume of the basic burette, the concentration of standard KOH, and analytical balance). Therefore, ATR-FTIR is an effective alternative method for the AOCS titration method.
Blind sample validation
Blind sample verification displays the noteworthy and prominent relationship between the calibrated model and the real situation. It is an analytical method to verify whether the established method is dependent on the oil variety. Soybean oil, flaxseed oil, coconut oil, cottonseed oil, peanut oil, rapeseed oil, corn oil, rice bran oil, and camellia oil were selected as blind samples. Fig. 4 presents a composite plot of all the FFA results obtained by the calibration model and the AOCS method. The following linear regression equation for the composite data was obtained from Fig. 4. |
FFA(ATR-FTIR) (%) = 1.0860 FFA(AOCS) (%) + 0.0070
| (6) |
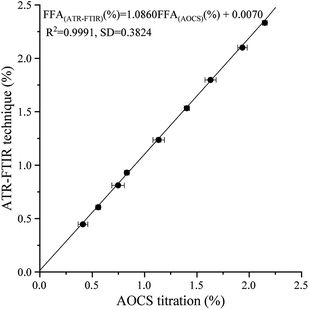 |
| Fig. 4 Plots of FFA results obtained from blind samples by the ATR-FTIR technique and AOCS method. | |
The expected result of the blind verification was analogous to the internal verification model (eqn (4)). In eqn (6), the R2 value, SD value, RMSEP and slope and vertical intercepts were 0.9991, 0.3824, 0.0127, 1.0860, and 0.0070, respectively. These results further proved the effectiveness and reasonability of the established model. Given the storage and usage situations of vegetable oils, the slope of the blind sample verification was reasonably slightly higher than the results of internal verification.
Precision analysis
FTIR spectroscopy has been extensively employed in vegetable oils. Extensive research has been applied to quantify FFA in vegetable oils. Al-Alawi has applied FTIR technique to measure FFA with contents of 0–4% in vegetable oils, and standard error was determined to be ≤ 0.02%.26 Dong et al., using a disposable polyethylene film in FTIR technique, found that the FFA content in vegetable oil was between 0% and 3%.19 Given the intrinsically short path length of ATR cells, the sensitivity of the results in this study (FFA content was between the range of 0.4–4.0%, with an S.D. of ±0.035%, the RE was less than 0.246%, and the RD was 0.386%) was slightly lower than that in the above-mentioned research. However, the conventional FTIR instrument was equipped with an ATR accessory to measure FFA content in oils. This instrument is relatively cheap, easy to operate, convenient to clean, and is suitable for commercialization. In the proposed method, sample preparation is simple, and no special requirements for sample size, water content, and shape are needed. It is a simple and reasonable method.
Conclusions
The primary objective of this study was to develop a simple, less sample consumption and highly sensitive method that does not require toxic and pungent reagents for predicting FFA in vegetable oils by ATR-FTIR spectroscopy. FFA can be converted to carboxylate by using a phthalimide potassium salt. A linear calibration between FFA contents and peak areas at 1541–1616 cm−1 (1595 cm−1 as baseline) was established by detecting ν(COO−) band antisymmetric stretching vibration. The R2 values during calibration and validation were more than 0.99. The RMSEP value was 0.0104 in the calibration model. Obtained by the developed method, the calibration methods concur to within ±0.035% over the range of 0.4–4.0% in vegetable oils. The RE was less than 0.246%, and the RD was 0.386%. These indexes prove that the calibration model has good accuracy, high precision, and good stability. The slope and intercept of the verification equation between the FFA prediction values and AOCS titration values were close to 1 and 0, respectively. The FFA content predicted by the ATR-FTIR technique was significantly correlated with the results determined by the AOCS method. Therefore, the proposed method is practically useful in FFA determination from the analytical viewpoint and can be used as a promising method for the analysis of FFA in different kinds of edible oils.
Abbreviations
FFA | Free fatty acid |
AOCS | American Oil Chemists' Society |
FTIR | Fourier transform infrared |
ATR | Attenuated total reflection |
SD | Standard deviation |
RD | Relative mean deviation |
RE | Relative error |
RMSEP | Root mean square error of prediction |
Conflicts of interest
Yaoyao Dong, Shaoxia Shi, Qi Li, Lingyan Zhang and Xiuzhu Yu declare that they have no conflict of interest.
Acknowledgements
The authors would like to thank the National Natural Science Foundation of China (NO.: 31671819) for financial support.
References
- I. Tarhan, A. A. Ismail and H. Kara, Int. J. Food Prop., 2017, 20, 1–8 CrossRef.
- X. Jiang, S. Li, G. Xiang, Q. Li, L. Fan, L. He and K. Gu, Food Chem., 2016, 212, 585–589 CrossRef CAS PubMed.
- Y. Guo, X. Liang, J. Bi, R. Ling, Y. Jiang, Z. Mou, F. Huang and W. Qin, Food Chem., 2019, 285, 450–457 CrossRef CAS PubMed.
- A. H. Chizari and M. Fehrestisani, Supply Chain Forum: An International Journal, 2018, 19, 132–141 CrossRef.
- S. G. Li, Z. Hui and W. T. Xue, Eur. J. Lipid Sci. Technol., 2010, 109, 1088–1094 CrossRef.
- P. Wan, M. K. Dowd, A. E. Thomas and B. H. Butler, J. Am. Oil Chem. Soc., 2007, 84, 701–708 CrossRef CAS.
- E. Kardash-Strochkova, Y. I. Tur'yan, A. Shenhar and I. Kuselman, Croat. Chem. Acta, 2003, 76, 329–334 CAS.
- O. Y. Berezin, Y. I. TurYan, L. Kogan, I. Kuselman and A. Shenhar, J. Am. Oil Chem. Soc., 1997, 74, 1339–1341 CrossRef CAS.
- Y. I. Turyan, O. Y. Berezin, I. Kuselman and A. Shenhar, J. Am. Oil Chem. Soc., 1996, 73, 295–301 CrossRef CAS.
- I. Kuselman, Y. I. Tur'yan, O. Y. Berezin, L. Kogan and A. Shenhar, J. AOAC Int., 1998, 81, 873–879 CrossRef CAS PubMed.
- M. Kadam and D. N. Bhowmick, J. Food Lipids, 2006, 13, 354–361 CrossRef CAS.
- B. W. Kail, D. D. Link and B. D. Morreale, J. Chromatogr. Sci., 2012, 50, 934–939 CAS.
- G. T. Zhu, F. Liu, P. Y. Li, S. He, S. K. Zhu, Q. Gao and Y. Q. Feng, Food Chem., 2019, 297, DOI:10.1016/j.foodchem.2019.124998.
- M. G. Williams and J. Macgee, J. Am. Oil Chem. Soc., 1983, 60, 1507–1509 CrossRef CAS.
- J. N. Conceição, B. S. Marangoni, F. S. Michels, I. P. Oliveira, W. E. Passos, M. A. G. Trindade, S. L. Oliveira and A. R. L. Caires, Fuel Process. Technol., 2019, 183, 1–7 CrossRef.
- R. Kumar, V. Bansal, A. K. Tiwari, M. Sharma, S. K. Puri, M. B. Patel and A. S. Sarpal, J. Am. Oil Chem. Soc., 2011, 88, 1675–1685 CrossRef CAS.
- S. Sivakesava and J. Irudayaraj, J. Food Sci., 2001, 66, 972–978 CrossRef CAS.
- Y. W. Zhuang, Z. Y. Ren, L. Jiang, J. X. Zhang, H. F. Wang and G. B. Zhang, Spectrochim. Acta, Part A, 2018, 199, 146–152 CrossRef CAS PubMed.
- X. Dong, Q. Li, D. Sun, X. Chen and X. Yu, Food Anal. Methods, 2015, 8, 857–863 CrossRef.
- Y. B. Che Man, M. H. Moh and F. R. van de Voort, J. Am. Oil Chem. Soc., 1999, 76, 485–490 CrossRef.
- M. Mahboubifar, S. Yousefinejad, M. Alizadeh and B. Hemmateenejad, J. Iran. Chem. Soc., 2016, 13, 1–9 CrossRef.
- A. C. Lanser, G. R. List, R. K. Holloway and T. L. Mounts, J. Am. Oil Chem. Soc., 1991, 68, 448–449 CrossRef CAS.
- A. Alalawi, F. R. van de voort, J. Sedman and A. Ghetler, J. Assoc. Lab. Autom., 2006, 11, 23–29 CrossRef CAS.
- S. T. H. Sherazi, S. A. Mahesar, M. I. Bhanger, F. R. van de Voort and J. Sedman, J. Agric. Food Chem., 2007, 55, 4928–4932 CrossRef CAS.
- X. Yu, F. R. van de Voort, J. Sedman and J. M. Gao, Anal. Bioanal. Chem., 2011, 401, 315–324 CrossRef CAS PubMed.
- A. Al-Alawi, F. R. van de Voort and J. Sedman, J. Am. Oil Chem. Soc., 2004, 81, 725 CrossRef CAS.
- A. A. Ismail, F. R. van de Voort, G. Emo and J. Sedman, J. Am. Oil Chem. Soc., 1993, 70, 335–341 CrossRef CAS.
- F. N. Arslan and F. Caglar, Food Anal. Methods, 2019, 12, 355–370 CrossRef.
|
This journal is © The Royal Society of Chemistry 2020 |
Click here to see how this site uses Cookies. View our privacy policy here.