DOI:
10.1039/D0RA04162A
(Paper)
RSC Adv., 2020,
10, 27249-27258
Barrier-promoting efficiency of two bioactive flavonols quercetin and myricetin on rat intestinal epithelial (IEC-6) cells via suppressing Rho activation
Received
9th May 2020
, Accepted 15th July 2020
First published on 21st July 2020
Abstract
Polyphenols are beneficial to human health because of their bio-activities. In this study, two flavonols quercetin and myricetin with or without heat treatment at 100 °C for 30 min were assessed for their barrier-promoting efficiency in rat intestinal epithelial (IEC-6) cells. The results indicated that the heated and unheated flavonols at dose levels of 2.5–20 μmol L−1 had a nontoxic effect on the cells treated for 24 and 48 h but enhanced the values of cell viability larger than 100% (especially at a dose level of 5 μmol L−1). Moreover, the cells exposed to these flavonols of 5 μmol L−1 for 24 and 48 h had improved barrier integrity compared to the control cells without any flavonol treatment, reflected by enhanced transepithelial electrical resistance and anti-bacterial effect but decreased paracellular permeability and bacterial translocation. Moreover, the results from both mRNA and protein expression verified 1.1–3.4 fold up-regulation of zonula occludens-1, occludin, and claudin-1 that are critical to tight junctions and barrier function of cells. Furthermore, the expression of other two proteins RhoA and ROCK in the treated cells was also down-regulated, demonstrating suppressed Rho activation and consequently barrier promotion via the RhoA/ROCK signaling pathway. Overall quercetin, due to its lower molecular polarity, mostly gave higher barrier-promoting efficiency than myricetin, while the heated flavonols were always less efficient than the unheated counterparts to promote barrier integrity of IEC-6 cells. It is thus highlighted that flavonols can provide barrier-promoting effects on intestinal epithelial cells with a promoting efficiency dependent on flavonol polarity; however, heat treatment especially excessive heat treatment of plant foods might lead to damaged flavonol activity.
Introduction
The small intestine in the body is not only the final organ to digest and absorb dietary nutrients, but also the key organ to prevent exogenous pathogens from entering systemic circulation.1 Thus, intestinal integrity is essential for the survival, growth, and health of animals and humans. It has been found that the occurrence of a variety of diseases is related to intestinal mucosal barrier dysfunction.2 Reasonably, it is assumed that the enhancement of the intestinal barrier can reduce the risk of diseases, because an impaired intestinal barrier may result in an easy barrier crossing of lipopolysaccharides, peptidoglycans, bacteria, and other harmful factors into the body. As we know, the entrance of these harmful factors into the body is responsible for the pathogenesis of many diseases such as inflammatory bowel diseases and leaky gut syndrome.3–5 However, various dietary fractions can contact intestinal mucosa and thereby affect barrier and other functions of the small intestine. Dietary fibers, for example, can be fermented in the intestinal lumen, resulting in the production of the so-called short-chain fatty acids (SCFAs). The three past results thus found that SCFAs could support the proliferation of intestinal epithelial cells and more important, protect the integrity of the intestinal barrier.6–8 Epidemiological evidence also indicated that increased protein intake might lead to an increase in intestinal permeability, due to the production of toxic substances by the proteins and microbial interactions.9,10 Using animal models, high-fat and high-sugar Western diets were evidenced to impaire intestinal barrier function.11,12 Moreover, food additives like emulsifiers, surfactants, and organic solvents, which are often used in food processing, were also proved to cause the opening of tight junctions (TJ) between cells and thus damage barrier function in animal experiments.13–16 Overall, dietary components might have both positive and negative effects on intestinal barrier function. Because of the particularity of intestinal structure and function, the progress in this field will be of fundamental importance based on the importance of human health.
TJ of intestinal epithelium undertakes several complex tasks, including the paracellular transport of water, various ions and nutrients as well as blocking bacterial translocation, which points out the importance of exploring TJ permeability and barrier function.17,18 However, this balance can be disrupted under disease condition.18 TJ is comprised by a series of fusion points between the outer leaves of adjacent cells, which can fill the gap between cells. In the present time, three complete proteins occludin, claudins, and junction-associated molecule (JAM) have been found in TJ. The first two constitute the backbone of TJ chain, while JAM seems to be important to immune surveillance and inflammatory response.19–22 At the same time, zonula occludens-1 (ZO-1) is connected to occludin and claudins via different domains, while its carboxyl terminal can bind to actin cytoskeleton. Thus, the proteins at TJ can communicate with the proteins in the cells through ZO-1.21–25 In the family of claudins, claudin-1 exists in high resistance epithelial cells but not in leaky epithelial cells; hence, claudin-1 is very important for the mammalian epidermal barrier.26 Occludin, claudin-1, and ZO-1 are therefore widely used to assess the TJ-associated barrier function of intestinal epithelial cells.
A variety of vegetables and fruits are indispensable in daily diet because of their nutritional values and health benefits. In recent years, it has been found that polyphenols in these foods have desired biological functions. Flavonoids as the most important polyphenols have attracted wide attention because of their good anti-oxidant, anti-inflammatory, anti-cancer, anti-cardiovascular disease, and anti-aging effects.27–30 In view of the importance of intestinal barrier function and the ubiquity of flavonoids with excellent biological activities, we also try to explore whether there is a direct relationship between them. Quercetin and myricetin are flavonols in the flavonoid family.31 Intake of quercetin and myricetin will lead to direct interaction between the two flavonols and intestinal epithelial cells. However, there are few studies to assess their barrier-promoting efficiency in rat intestinal epithelial cells (e.g. IEC-6 cells). What more noteworthy is that molecular structures of quercetin and myricetin are same in both A-ring and C-ring but different in B-ring (two –OH versus three –OH). This structural character arouses our enthusiasm for further research to reveal whether number change of –OH in B-ring might affect their barrier-promoting efficiency. Moreover, flavonols are sensitive to oxygen and thus easy to be oxidized.32,33 Hence, heat treatment will accelerate oxidative rates of flavonols. Heat treatment is widely used in both family diets and industrially produced foods. In the past, flavonols were found with degradation after heating.34 It is also hoped that another question will be answered in this study; that is, whether heat treatment of the two flavonols will bring about changed barrier-promoting efficiency in IEC-6 cells.
In this study, quercetin and myricetin and their heated counterparts (100 °C, 30 min) were assessed for their barrier-promoting effects in IEC-6 cells, using those commonly used indicators likes transepithelial electrical resistance (TEER), paracellular permeability, anti-bacterial activity, and bacterial translocation. At the same time, the effects of these samples on barrier integrity of IEC-6 cells were also investigated from a molecular point of view, via assaying both gene and protein expression of occludin, claudin-1, ZO-1, RhoA, and ROCK. These obtained scientific data were used to determine whether the two flavonols had different barrier-promoting effects on IEC-6 cells, and whether the used heat treatment could damage or promote their barrier-promoting efficiency.
Materials and methods
Materials
Quercetin and myricetin (purity > 98%) were obtained from Shanghai Yousi Biotechnology Co., Ltd. (Shanghai, China). Fetal bovine serum (FBS) was brought from Wisent Inc. (Montreal, Quebec, Canada). Trypsin–EDTA was brought from Beyotime Institute of Biotechnology (Shanghai, China). Dulbecco's modified Eagle's medium (DMEM), dimethyl sulfoxide (DMSO), and 4 kDa fluorescein isothiocyanate (FITC)-dextran (FD-4), were all provided by Sigma-Aldrich Co. (St Louis, MO, USA). Phosphate-buffered saline (PBS) and Luria-Bertani (LB) agar were both purchased from Solarbio Science and Technology Co., Ltd. (Beijing, China). The water used in this study was ultrapure water prepared with a Milli-Q Plus system (Millipore Corp., New York, NY, USA). Other chemicals were analytical reagents.
Cell Counting Kit-8 (CCK-8) was purchased from Dojindo Molecular Technologies, Inc. (Kyushu, Japan). The primary antibodies such as GAPDH (ab181602), occludin (ab216327) and claudin-1 (ab15098) were all brought from Abcam plc. (Cambridge, UK). The primary antibodies RhoA (AF6352) and ZO-1 (AF5145) were produced by Affinity Biosciences (Cincinnati, OH, USA) while primary antibody ROCK (A11158) was brought from ABclonal, Inc., Company (Wuhan, Hubei, China). The goat anti-rabbit secondary antibody was obtained from Bioss Biotechnology Co., Ltd. (Beijing, China). The bacterial strain Escherichia coli (ATCC 25922) was kindly provided by the Food Microbiology Laboratory in Northeast Agricultural University (Harbin, Heilongjiang, China), and stored at −80 °C until used.
Culture conditions of the cells
IEC-6 cells, having the characteristics of stable passage of crypt epithelial cells,35 were obtained from the American Type Culture Collection (Rockville, MD, USA), cultured in an incubator (Type HF 90, Heal Force, Hongkong, China) with a medium composing of 10% FBS, 90% DMEM, 100 U L−1 bovine insulin, and 100 μg mL−1 penicillin and streptomycin. Incubator temperature was set at 37 °C while CO2 concentration was adjusted to 5%.
Heat treatment of polyphenols and assay of their cytotoxic effects
Both quercetin and myricetin were dissolved in DMSO to reach a concentration of 40 mmol L−1, and then diluted with serum-free medium to dose levels of 2.5–20 μmol L−1. The solution of each dose level was divided into two groups. One group was directly used to treat the cells, while the other group was heated at 100 °C for 30 min and used to treat the cells after cooling.
The cells were inoculated in 96-well plates (100 μL per well) and adjusted cell density to 2 × 103 cells per well. After the cells attached, they were starved in serum-free medium for 12 h. Fresh medium containing the unheated or heated flavonols was used to treat cells for 24 and 48 h respectively. The cells treated with normal medium (negative control) were regarded as 100% cell viability by CCK-8 assay. According to the kit instruction, the cells were washed twice with PBS (10 mmol L−1, pH 7.2) after medium removal, and added with fresh medium containing 10% CCK-8 solution. After an inoculation of 90 min, the absorbance of each well at 450 nm was measured at a microplate reader (Bio-Rad Laboratories, Hercules, CA, USA). Cell viability was calculated as previously described.36
Assay of transepithelial electrical resistance
Transwell inserts with growth area of 1.12 cm2 and membrane pore size of 0.4 μm (Corning, Kennebunk, ME, USA) were placed in 12-well plates. Cell suspension (5 × 105 cells per mL, 0.5 mL) was inoculated into the apical side, while normal medium (1.5 mL) was added to the basolateral side. TEER value was measured every 24 h using the Millicell-ERS2 Volt-Ohm Meter (Millipore Corp., Bedford, MA, USA). Fresh medium was changed every other day until TEER value reached to 50 Ω cm2. The cells in the inserts were treated with a serum-free medium for 12 h. The medium containing the unheated or heated flavonols was used to culture the cells for 24 and 48 h. The cells treated with normal medium were also used as negative control with TEER value of 100%. The specific calculation refers to the previous method.36
Assay of paracellular permeability
This assay was done using the Transwell inserts. When TEER value reached to 50 Ω cm2, the cells were treated with the medium containing the unheated or heated flavonols for 24 and 48 h, while the cells treated with normal medium were served as negative control. After that, medium containing FD-4 of 0.5 mg mL−1 was added to the apical side for 24 h. Finally, 100 μL medium was out of the basolateral side, placed in 96-well plates, and detected using fluorescence microplate reader (Infinite M200 pro, TECAN, Männedorf, Switzerland). The excitation and emission wavelengths were set at 490 and 520 nm, respectively. The result was expressed as percentage value of the negative control as a previous study did.36
Assay of anti-bacterial activity
Resistance ability to E. coli ATCC-25922 of the supernatant of the cells treated with the unheated and heated flavonols for 24 and 48 h was evaluated. The rejuvenated bacteria in the Luria-Bertani (LB) medium cultured to logarithmic phase were washed with the PBS for 3 times, and bacterial concentration was adjusted to 1 × 106 CFU mL−1 using the PBS. Bacterial suspension of 10 μL was mixed with 500 μL cell supernatant and cultured at 37 °C for 2 h. The cells treated with fresh medium were served as a control. Anti-bacterial activity was determined as previously described.36
Assay of bacterial translocation
The cells were inoculated into transwell inserts (12 mm diameter, 3.0 μm pore size, polyester membrane, Corning, Kenabond, ME, USA) until TEER value reached to 50 Ω cm2. The cells were then exposed to the medium containing the unheated or heated flavonols for 24 and 48 h. The cells treated with normal medium were used as negative control. E. coli was added to the apical side of inserts with adjusted level of 105 CFU per well. The medium out of the basolateral side (100 μL) was placed on the LB Agar to determine the colony number after E. coli and the cells were co-cultured for different times (1–4 h).
Quantitative real-time PCR assay
IEC-6 cells were plated at 5 × 105 cells per dish, cultured with serum-free medium for 12 h after cell adherence, and then treated with the samples (5 μmol L−1) for 24 h. The cells treated with normal medium were served as a control. The total RNA was extracted from the cells using the RNAprep Pure Cell Kit (Tiangen Biochemical Technology Co., Ltd., Beijing, China), while reverse transcription of cDNA was done using the PrimeScript TMRT Reagent Kit (Takara Bio Ltd., Kusatsu, Japan). Go Tag®qPCR Master Mix kit (Promega (Beijing) Biotechnology Co., Ltd., Beijing, China) and Applied Biosystems StepOnePlus Real-time PCR System (Life Technologies Corp., Carlsbad, CA, USA) were used in PCR amplification. Primer sequences of ZO-1, occludin, claudin-1, RhoA, and ROCK-1 (Table 1) were designed by Thermo Fisher Scientific (China) Co., Ltd (Shanghai, China). Glyceraldehyde-3-phosphate dehydrogenase (GAPDH) was used as internal standard. Expression levels of these genes were analyzed with the 2−ΔΔCt method, where ΔΔCt = ΔCttest − ΔCtcontrol.37
Table 1 The primers used in this study
Gene |
Species |
|
Primer (5′–3′) |
ZO-1 |
Rat |
FORWARD |
CCACCTCGCACGTATCACAAGC |
REVERSE |
GGCAATGACACTCCTTCGTCTCTG |
Occludin |
Rat |
FORWARD |
CCTCCTTACAGGCCGGATGA |
REVERSE |
AGCATTGGTCGAACGTGCAT |
Claudin-1 |
Rat |
FORWARD |
GTTTCATCCTGGCTTCGCTG |
REVERSE |
AGCAGTCACGATGTTGTCCC |
RhoA |
Rat |
FORWARD |
AGGCGGGAGTTAGCCAAAAT |
REVERSE |
GTACCCAAAAGCGCCAATCC |
Rock |
Rat |
FORWARD |
GGTGATGGAGTACATGCCAGGTG |
REVERSE |
ATCCAGTGCAAGCACGACTTCAG |
GAPDH |
Rat |
FORWARD |
CCCTCTGGAAAGCTGTGG |
REVERSE |
GCTTCACCACCTTCTTGATGT |
Western-blot assay
IEC-6 cells were plated at 2 × 106 cells per dish, treated for 24 h with the samples of 5 μmol L−1, washed by ice-cold PBS, and lysed on ice with a radio-immuno-precipitation assay (RIPA) lysis buffer (China Nanjing KGI Biotechnology Development Co., Ltd., Nanjing, Jiangsu, China) and 5 μL phenylmethansulfonyl fluoride (PMSF, 0.1 mol L−1) for 30 min. After centrifugation (14
000g) at 4 °C for 5 min, cell supernatants were collected and measured for protein concentrations using a BCA protein concentration detection kit (Beyotime Institute of Biotechnology, Shanghai, China). SDS-PAGE gels (30 μg per well) of 7.5% (ZO-1 and ROCK), 10% (occludin and claudin-1), and 13% (RhoA) were used to separate these proteins. The gels were transferred to the polyvinylidene fluoride (PVDF) membranes after electrophoresis. Skimmed milk in PBS of 5% including 0.1% Tween-20 (PBST) was used to block the PVDF at 37 °C for 2 h. After then, primary antibodies (1
:
1000 dilution) were used to connect with these proteins at 4 °C overnight. PBST was used to wash the membranes for three times. Secondary horseradish peroxidase-conjugated antibody (1
:
1500 dilution) was used to incubate with the membrane at 20 °C for 1 h. The enhanced chemiluminescence was used to detect protein bands at a Dolphin Series Gel Imaging System (Weiteke Corp., Ltd., Reno, NY, USA). The Gel-Pro Analyzer 4 software (Media Cybernetics, Rockville, MD, USA) was used in quantification. GAPDH was used as endogenous standard to normalize band density.
Statistical analysis
The data were reported as the averages ± standard deviations of three independent assays. Univariate analysis of variance (ANOVA) was used for significance analysis between the groups. The data were analyzed by Duncan multiple comparison using the SPSS software package version 16.0 (SPSS Inc., Chicago, IL, USA). The results of bacterial translocation were analyzed by chi-square test.
Results
Cytotoxic effect of these flavonols on the cells
It was necessary to determine whether the cells might receive obvious toxicity when they were exposed to quercetin and myricetin. The results from CCK-8 assay showed that the cells treated with the unheated and heated flavonols of 2.5–20 μmol L−1 had viability values of 80.9–119.4 (24 h) or 82.4–122.9% (48 h) (Fig. 1), indicating both growth inhibition and proliferation. When the two flavonols were used at 5 μmol L−1, the cells totally had the highest viability values. The data also reflected that using the heated flavonols in cells always led to lower viability values than using the unheated flavonols, suggesting the conducted heat treatment induced increased cytotoxic effect. It was also found that viability values of the treated cells were enhanced accompanying the increase of treating time, because the viability values of 48 h were always higher than those of 24 h. Moreover, quercetin showed higher activity than myricetin in the cells. In the following assays of this study, dose level 5 μmol L−1 was thus regarded as a better selection for cell exposure.
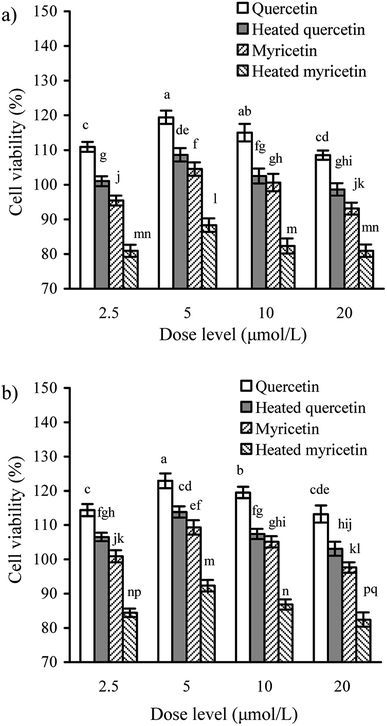 |
| Fig. 1 Viability values (%) of IEC-6 cells treated with the unheated and heated quercetin and myricetin at four dose levels for 24 (a) and 48 h (b). Different letters above the columns indicate that one-way ANOVA of the means differs significantly (p < 0.05). | |
Effect of these flavonols on transepithelial electrical resistance
TEER assay is a classic method to detect the integrity of cell monolayer. Thus, barrier integrity of these treated cells was also judged by the measured TEER values. The results showed that the treated cells during incubation had TEER increases gradually (Fig. 2), evidencing their enhanced barrier integrity. The cells treated with quercetin showed higher TEER values than these treated with myricetin (e.g. 133.4% versus 124.6% at 48 h), indicating quercetin had higher barrier-promoting efficiency than myricetin. Also found, the unheated flavonols were always more efficient than the heated ones to increase TEER values, highlighting barrier-promoting efficiency of the two flavonols was reduced by the used heat treatment.
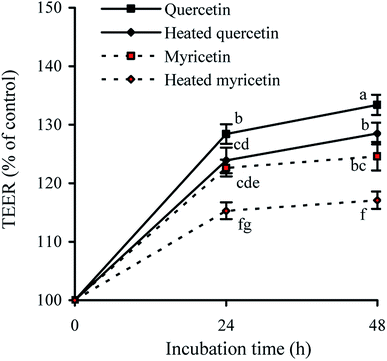 |
| Fig. 2 Time-responses of TEER in IEC-6 cells treated with the unheated and heated quercetin and myricetin at 5 μmol L−1 for 24 and 48 h. Different letters above the columns indicate that one-way ANOVA of the means differs significantly (p < 0.05). | |
Effect of these flavonols on paracellular permeability
The permeability assay also can reflect intestinal mucosal barrier function, because an increased permeability means worse TJ and weaker intestinal barrier. When the cells were treated with the unheated and heated flavonols for 24 and 48 h, cumulative throughput of FD-4 transferred from the apical to basolateral sides was measured to show paracellular permeability of the cells. The results (Fig. 3) demonstrated that all treated cells had FD-4 flux less than 100%, suggesting an improved barrier function. Moreover, longer treating time led to much reduction in paracellular permeability or greater barrier promotion. Overall, the quercetin-treated cells received better barrier than the myricetin-treated ones (FD-4 flux 74.2% versus 83.3% at 48 h), while the heated flavonols were consistently less efficient than the unheated counterparts (FD-4 flux 81.5% versus 74.2% for quercetin at 48 h, or 91.7% versus 83.3% for myricetin at 48 h). These results thus also verified barrier promotion of two flavonols on IEC-6 cells and how heat treatment damaged this activity.
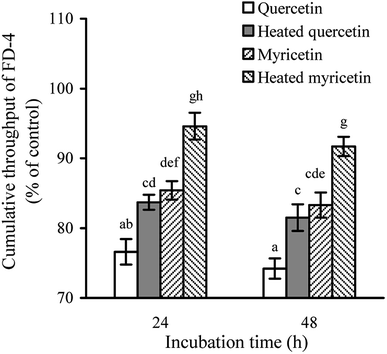 |
| Fig. 3 FD-4 diffusion in IEC-6 cells treated with the unheated and heated quercetin and myricetin at 5 μmol L−1 for 24 and 48 h. Different letters above the columns indicate that one-way ANOVA of the means differs significantly (p < 0.05). | |
Anti-bacterial activity and bacterial translocation of the treated cells
The assaying results mentioned above confirmed that the two flavonols were able to enhance barrier function of IEC-6 cells, and reasonably possessed helpful capacity in the intestine to prevent harmful bacteria from entering blood circulation. Two other assays were hence used to verify this capacity: anti-bacterial activity and bacterial translocation.
First, all treated cells showed increased anti-bacterial activity, because lower bacterial numbers were detected (Fig. 4a). Using different culture times (24 and 48 h) totally led to unclear change in anti-bacterial activity for the treated cells. Consistently, heat treatment of the two flavonols clearly caused lower anti-bacterial activity because larger bacterial numbers were detected. More interesting, the myricetin-treated cells displayed higher anti-bacterial activity than the quercetin-treated cells. Second, all treated cells showed reduced bacterial translocation, because fewer positive inserts were detected (Fig. 4b and c), suggesting the two flavonols possessed capacity to strength biological barrier function of IEC-6 cells. In detail, cell treatment of 24 or 48 h by these samples had no influence on the assessed bacterial translocation, while quercetin performed an efficiency same to myricetin to block bacterial translocation. It was also seen that longer co-culture time of the cells and bacteria led to more positive inserts or greater bacterial translocation. In consistence with other assaying results of this study, the cells treated with the unheated flavonols gave decreased positive inserts or reduced bacterial translocation (i.e. enhanced barrier integrity), verifying that the heat treatment weakened barrier-promoting efficiency of the two flavonols.
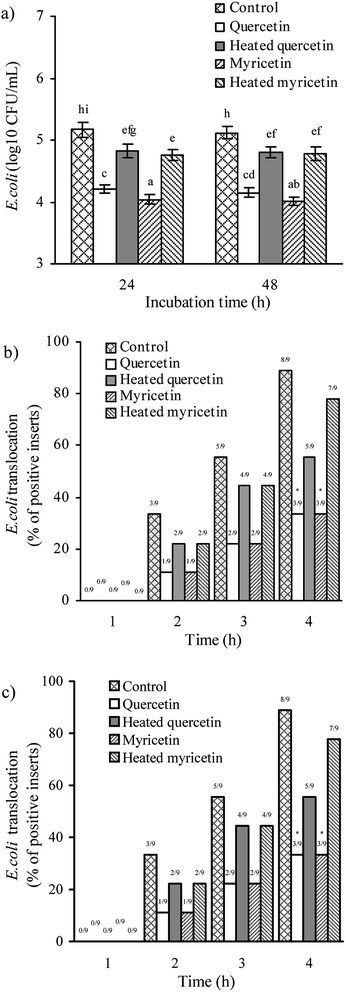 |
| Fig. 4 Anti-bacterial activities (a) of the culture supernatants of IEC-6 cells as well as E. coli translocation across the cell monolayer with flavanol treatment of 24 (b) or 48 h (c). The unheated and heated quercetin and myricetin were used at 5 μmol L−1. Different letters above the columns indicate that one-way ANOVA of the means differs significantly (p < 0.05). The asterisks indicate the significance from the control cells determined by the chi-squared tests. | |
Expression changes of the TJ-related mRNA and proteins in the treated cells
Barrier function of cells is mediated by TJ proteins; thus, the treated cells were assayed for the expression of ZO-1, occludin, and claudin-1 in both mRNA and protein levels. The results (Fig. 5a) showed that mRNA expression levels of ZO-1, occludin, and claudin-1 were all up-regulated. Following this up-regulated mRNA expression, the three TJ proteins were also up-regulated (Fig. 5b). Overall, their mRNA and protein expression levels were increased by 1.2–1.7 and 1.1–3.4 folds, respectively. Up-regulated ZO-1, occludin, and claudin-1 might lead to firm TJ, declaring barrier promotion of these samples applied to IEC-6 cells. It was also found that quercetin showed better barrier-promoting efficiency than myricetin, because it induced higher up-regulation on the three TJ proteins. Furthermore, heat treatment of the two flavonols damaged their activities to promote barrier integrity; subsequently, the treated cells received reduced expression of ZO-1, occludin, and claudin-1. From a molecular point of view, heat treatment of flavonols was adverse to the barrier-promoting function of flavonols.
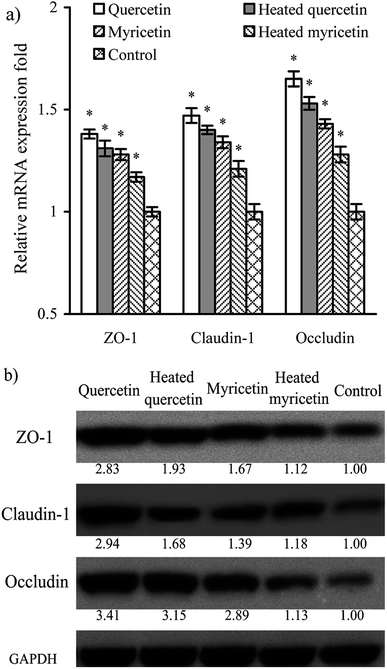 |
| Fig. 5 Relative mRNA (a) and protein (b) expression of ZO-1, occludin, and claudin-1 in IEC-6 cells treated with the unheated and heated quercetin and myricetin at 5 μmol L−1 for 24 h. *p < 0.05, compared with the control group. | |
A signaling pathway mediating barrier function of the treated cells
The results above proved that both quercetin and myricetin could up-regulate the three TJ-related proteins. Thus, it was necessary to verify the possible signaling pathway that mediates the TJ-related proteins; for example, the RhoA/ROCK pathway. The treated IEC-6 cells were detected for the mRNA and protein expression of RhoA and ROCK. The results showed that mRNA expression levels of RhoA and ROCK in the treated cells were reduced clearly, compared with the control cells without flavonol treatment (Fig. 6a). At the same time, protein expression levels of RhoA and ROCK in the cells were also decreased by flavonol treatment (Fig. 6b). Down-regulated RhoA and ROCK expression in the treated cells indicates a suppressed Rho GTPase activation. A signaling pathway mediating barrier function of IEC-6 cells is thus deciphered (Fig. 7). Compared with quercetin and myricetin, the heated counterparts all showed lower potentials to perform their action. This fact means heat treatment especially excessive heat treatment of the two flavonols was an unbeneficial event, considering barrier-promoting effects of flavonols on IEC-6 cells.
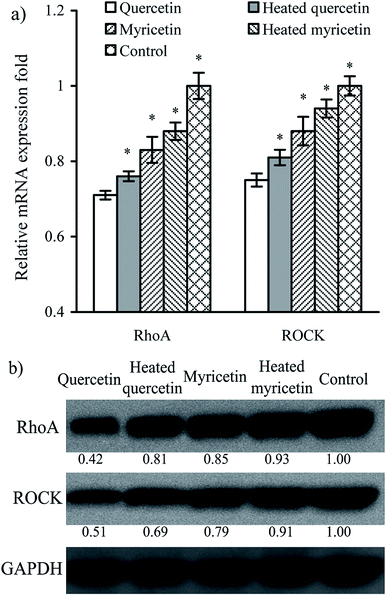 |
| Fig. 6 Relative mRNA (a) and protein (b) expression of RhoA and ROCK in IEC-6 cells treated with the unheated and heated quercetin and myricetin at 5 μmol L−1 for 24 h. *p < 0.05, compared with the control group. | |
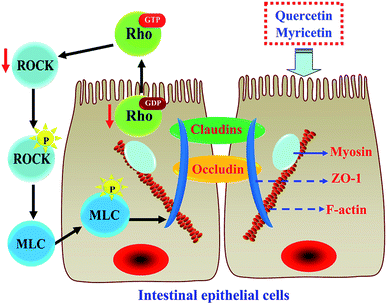 |
| Fig. 7 A signaling pathway responsible for barrier-promoting effect of quercetin and myricetin in cells. Two flavonols act on IEC-6 cells to cause the suppression of RhoA/ROCK. This suppression prevents the separation of GDP from the Rho GTPase, reduces enzyme activity, and causes the suppression of ROCK. Afterwards, phosphorylation of myosin light chain phosphatase (MLCP) is reduced while phosphorylation of myosin light chain (MLC) is suppressed, which leads to contractility inhibition of the actinomyosin. Subsequently, ZO-1 protein will highly interact with occludin and claudins proteins to form tight junctions, causing enhanced barrier function. | |
Discussion
As is known to us, paracellular barrier of the intestine is very important for biological survival, and TJ is an important part of the small intestinal barrier. TJ of intestinal epithelial cells could be regulated by a variety of stimuli; for example, glycated casein digest and butyrate that were reported to improve barrier integrity of IEC-6 cells by reducing paracellular permeability.36,38 It is estimated that dietary intake of flavonoids ranges from 3 to 1000 mg d−1.39 Thus, biogenic flavonoids are possible to interact with intestinal epithelial cells and consequently lead to changed barrier integrity. Today, many plant foods rich in flavonoids are consumed in the form of commercial processed products, for which heat treatment is a necessary processing step aiming to inactivate harmful microorganisms or toxins. Possible impact of food processing or storage on bioactive properties of polyphenols is an important issue to food scientists. A study comparing the barrier-promoting efficiency of various flavonoid compounds or verifying whether heat treatment has positive or negative effect on their barrier promotion thus deserves our consideration. Dietary flavonoids mainly exist in the form of glycoside conjugates (except of flavan-3-ols and proanthocyanidins). It is generally believed that the absorption of these glycosides is related to glycoside hydrolysis. If these glycosides can't be hydrolyzed in the small intestine, they will be transported to the colon where they will be hydrolyzed by microbes to release aglycone.40,41 It was found that even if dietary flavonoids were absorbed in the small intestine, a large amount of them was still transferred to the large intestine.42,43 A study on the biotransformation of myricetin in mice also showed that the main metabolic site of myricetin was the colon.44 Dietary flavonoids are thereby difficult to be absorbed in the small intestine, and reasonably exert various effects on intestinal epithelial cells. It is reasonable for this study using quercetin and myricetin (aglycone forms) as target substances.
Using these evaluated indices, both quercetin and myricetin were verified to provide barrier promotion for IEC-6 cells, causing enhanced TEER and anti-bacterial effect but decreased paracellular permeability and bacterial translocation. Moreover, quercetin mostly was more effective than myricetin to perform this barrier promotion, which might associate with their different chemical structures. Based on their chemical formulae, quercetin and myricetin have 5 and 6 hydroxyl groups in their molecules, respectively. Quercetin thus has lower polarity than myricetin. It has been proposed that flavonoids with less non-modification in the B-ring have the greatest interactivity with cell membrane.45 It is thus a reasonable conclusion for this study that quercetin had higher efficiency than myricetin in IEC-6 cells, due to its lower polarity and easy enrichment in the cells to perform biological function.46 Why myricetin led to better antibacterial effect than quercetin in the cells might be also arisen from their different structures in B-ring. It was found that if B-ring was replaced, anti-bacterial activity was enhanced; however, A-ring substitution reduced anti-bacterial activity.47 Anti-bacterial activity of flavonoids is regarded due to their inhibition of DNA gyrase, while this inhibitory activity is limited to B-ring hydroxylated compounds.48,49 For example, quercetin gives anti-bacterial action on E. coli through its inhibition on DNA gyrase.50 In B-ring, myricetin has three hydroxyl groups while quercetin has two hydroxyl groups. Myricetin was able to give stronger inhibition on E. coli in cell culture. Afterwards, the myricetin-treated cells were measured with higher anti-bacterial activity than those quercetin-treated cells.
In general, sensitive food components might carry out various chemical changes during food processing, which is important to the food industry when considering the potential beneficial biofunctions of these components. Polyphenols from a chemical point of review are unstable, and their degradation are easily accelerated by these environmental factors like temperature, oxygen/oxidants, and alkaline, because of high chemical susceptivity of polyphenols to these factors. It is known that when quercetin is undergone heat treatment in water, the main degraded products have chemical structures different from quercetin itself; for example, the formed 2,4,6-trihydroxymandelate and 2,4,6-trihydroxyphenylgloxylate that do not belong to the flavonol family.51 Moreover, polyphenols like anthocyanins and procyanidins were degraded in the processed berry fruits that were stored at ambient temperature, resulting in polyphenol losses accompanied by the formation of various polymeric pigments of higher molecular weights via condensation reaction.52 It is an accepted fact that structural variation may lead to different biological characteristics.53,54 Consequently, baking treatment essential to cocoa processing was proved to reduce total phenolic content of cocoa beans,55 while baking, pasteurization, and other processing of California almonds led to reduced total phenols and iron-reducing capacity.56 Based on these mentioned facts, it is possible that heat treatment of polyphenols will lead to structural modification and then bioactivity changes. So, anti-cancer effects of four flavonols in human colorectal cancer HCT-116 cells was negatively impacted by both thermal and oxidative treatments,33 while the blueberry juice packed in glass bottles in response to the increase of storage time had decreased anthocyanin content and reduced inhibitory activity against human colon cancer HT-29 cells.57 A previous study also indicated that the polyphenol fractions obtained from thermally treated blueberry products had lower anti-proliferation on murine liver cancer Hepa-1c1c7 cells than those obtained from non-thermally treated ones,58 proving a negative impact of thermal treatment on anti-cancer effects of polyphenols. Furthermore, structural change of other food components has been verified to alter their barrier promotion on cells. The results in two recent studies using IEC-6 cells as cell model showed that caseinate glycation of the Maillard-type led to decreased barrier promotion of the resultant caseinate digest, whereas that of the transglutaminase-type endowed the resultant caseinate digest with enhanced barrier promotion, compared with unglycated caseinate digest.36,59 The two glycated caseinate digests were different in their glycation sites and chemical structures of the conjugated saccharides, thus were detected with decreased and increased barrier-promoting efficiency. The two studies thus supported that the heated flavonols had changed barrier-promoting efficiency in the cells. Overall, heat treatment especially excessive heat treatment of plant foods should be paid a critical attention to examine its positive or negative effect on bioactivity of flavonoids, based on the present and other relevant results.
Possible biological mechanisms associated with barrier promotion of various substances in cells have been well-studied in both medicine and food fields. A pharmaceutical analogue FTY720 could enhance endothelial barrier function by a pathway involving c-Abl signaling.60 High density lipoprotein-associated sphingosine 1-phosphate was proved to promote endothelial barrier via the S1P1/Akt signaling pathway.61 Sinomenine could improve permeability of glomerular endothelial cells by inhibiting the activation of RhoA/ROCK signaling pathway.62 A propolis extract was able to increase the expression of occludin and ZO-1 via the activation of AMPK and ERK in human intestinal epithelial Caco-2 cells.63 Moreover, rutin (a derivative of quercetin) was declared to prevent the hyperglycemia-induced barrier dysfunction of renal endothelial cells through suppressing RhoA/ROCK signaling pathway.64 This study also observed the down-expression of RhoA and ROCK in IEC-6 cells in the presence of flavonol stimulus. TJ proteins in IEC-6 cells thus might be regulated through the RhoA/ROCK signaling pathway. In theory, when the RhoA/ROCK signaling pathway is activated, it induces the dynamic reorganization and contraction of the F-actin cytoskeleton around the cells; subsequently, this event damages cellular barrier integrity mediated by TJ proteins.65–67 Thus, the two flavonols were regarded to exert barrier-promoting effect on IEC-6 cells via attenuating Rho activation. The results from other studies also supported that RhoA/ROCK signaling pathway was indeed involved in barrier promotion via up-regulating ZO-1, occludin, and claudin-1 expression.68,69 However, whether other signaling pathways are also involved in barrier integrity of IEC-6 cells with flavonol stimulus should be verified in future study.
Conclusion
Both quercetin and myricetin at lower dose level were nontoxic to IEC-6 cells but could provide barrier-promoting effect on the cells via increasing transepithelial electrical resistance and anti-bacterial effect but decreasing paracellular permeability and bacterial translocation. From a biological point of review, three TJ proteins ZO-1, occludin, and claudin-1were up-regulated by the two flavonols through their suppression on the RhoA/ROCK signaling pathway that are critical to the TJ-mediated barrier function of cells. Overall, quercetin due to its lower molecular polarity had higher barrier-promoting efficiency in the cells than myricetin, while the heated flavonols at 100 °C for 30 min received reduced barrier promotion than the unheated counterparts. It is thus suggested that bioactive flavonols could provide a helpful action on intestinal epithelial cells through promoting cellular barrier integrity; at the same time, heat treatment especially excessive heat treatment of plant foods should be examined for its effect on flavonol activity, regarding its negative impact on barrier-promoting efficiency of bioactive flavonols.
Conflicts of interest
The authors declare no conflict of interest.
Acknowledgements
This study was funded by the Key Research Project in Science and Technology of The Education Department of Heilongjiang Province (Project No. 11551z018). The authors also thank the anonymous referees for their valuable advice.
References
- W. W. Souba, R. J. Smith and D. W. Wilmore, JPEN, J. Parenter. Enteral Nutr., 1985, 9, 608–617 CrossRef CAS PubMed
. - J. R. Turner, Nat. Rev. Immunol., 2009, 9, 799–809 CrossRef CAS PubMed
. - C. Nunes, R. Figueiredo, J. Laranjinha and G. J. da Silva, Chem.-Biol. Interact., 2019, 310, e108711 CrossRef PubMed
. - A. Michielan and R. D'Inca, Mediators Inflammation, 2015, 2015, 1–10 CrossRef PubMed
. - S. Citi, Science, 2018, 359, 1097–1098 CrossRef CAS PubMed
. - E. D. Sonnenburg and J. L. Sonnenburg, Cell Metab., 2014, 20, 779–786 CrossRef CAS PubMed
. - K. Makki, E. C. Deehan, J. Walter and F. Bäckhed, Cell Host Microbe, 2018, 23, 705–715 CrossRef CAS PubMed
. - H. Ichikawa and T. Sakata, J. Nutr., 1998, 128, 843–847 CrossRef CAS PubMed
. - S. Kakodkar and E. A. Mutlu, Clin. Gastroenterol., 2017, 46, 745–767 CrossRef PubMed
. - S. R. Llewellyn, G. J. Britton, E. J. Contijoch, O. H. Vennaro, A. Mortha, J. F. Colombel, A. Grinspan, J. C. Clemente, M. Merad and J. J. Faith, Gastroenterology, 2018, 154, 1037–1046 CrossRef PubMed
. - V. Volynets, S. Louis, D. Pretz, L. Lang, M. J. Ostaff, J. Wehkamp and S. C. Bischoff, J. Nutr., 2017, 147, 770–780 CrossRef CAS PubMed
. - M. Martinez-Medina, J. Denizot, N. Dreux, F. Robin, E. Billard, R. Bonnet, A. Darfeuille-Michaud and N. Barnich, Gut, 2014, 63, 116–124 CrossRef PubMed
. - B. Chassaing, O. Koren, J. K. Goodrich, A. C. Poole, S. Srinivasan, R. E. Ley and A. T. Gewirtz, Nature, 2015, 519, 92–96 CrossRef CAS PubMed
. - I. Kralova and J. Sjöblom, J. Dispersion Sci. Technol., 2009, 30, 1363–1383 CrossRef CAS
. - K. A. Hamid, H. Katsumi, T. Sakane and A. Yamamoto, Int. J. Pharm., 2009, 379, 100–108 CrossRef CAS PubMed
. - A. Lerner and T. Matthias, Autoimmun. Rev., 2015, 14, 479–489 CrossRef CAS PubMed
. - K. Dokladny, M. N. Zuhl and P. L. Moseley, J. Appl. Physiol., 2016, 120, 692–701 CrossRef CAS PubMed
. - B. Lee, K. M. Moon and C. Y. Kim, J. Immunol. Res., 2018, 2018, 1–11 Search PubMed
. - L. Langbein, Eur. J. Cell Biol., 2003, 82, 385–400 CrossRef CAS PubMed
. - M. Furuse, Cold Spring Harbor Perspect. Biol., 2010, 2, a002907 Search PubMed
. - B. Gumbiner, Am. J. Physiol., 1987, 253, C749–C758 CrossRef CAS PubMed
. - J. R. Turner, M. M. Buschmann, I. Romero-Calvo, A. Sailer and L. Shen, Semin. Cell Dev. Biol., 2014, 36, 204–212 CrossRef CAS PubMed
. - K. Umeda, J. Ikenouchi, S. Katahira-Tayama, K. Furuse, H. Sasaki, M. Nakayama, T. Matsui, S. Tsukita, M. Furuse and S. Tsukita, Cell, 2006, 126, 741–754 CrossRef CAS PubMed
. - L. Gonzalezmariscal, Prog. Biophys. Mol. Biol., 2003, 81, 1–44 CrossRef CAS
. - M. Itoh, J. Cell Biol., 1993, 121, 491–502 CrossRef CAS PubMed
. - D. Günzel and A. S. L. Yu, Physiol. Rev., 2013, 93, 525–569 CrossRef PubMed
. - S. Burda and W. Oleszek, J. Agric. Food Chem., 2001, 49, 2774–2779 CrossRef CAS PubMed
. - D. D. Orhan, B. Özçelik, S. Özgen and F. Ergun, Microbiol. Res., 2010, 165, 496–504 CrossRef CAS PubMed
. - T. Sergent, N. Piront, J. Meurice, O. Toussaint and Y. J. Schneider, Chem.-Biol. Interact., 2010, 188, 659–667 CrossRef CAS PubMed
. - R. Domitrović, K. Rashed, O. Cvijanović, S. Vladimir-Knežević, M. Škoda and A. Višnić, Chem.-Biol. Interact., 2015, 230, 21–29 CrossRef PubMed
. - W. Wang, C. Sun, L. Mao, P. Ma, F. Liu, J. Yang and Y. Gao, Trends Food Sci. Technol., 2016, 56, 21–38 CrossRef CAS
. - I. G. Zenkevich, A. Y. Eshchenko, S. V. Makarova, A. G. Vitenberg, Y. G. Dobryakov and V. A. Utsal, Molecules, 2007, 12, 654–672 CrossRef CAS PubMed
. - B. Wang, J. Wang and X. H. Zhao, J. Food Biochem., 2017, 41, e12310 CrossRef
. - N. Buchner, A. Krumbein, S. Rohn and L. W. Kroh, Mass Spectrom., 2006, 20, 3229–3235 CAS
. - A. Quaroni, J. Cell Biol., 1979, 80, 248–265 CrossRef CAS PubMed
. - J. Shi and X. H. Zhao, Food Funct., 2019, 10, 652–664 RSC
. - K. J. Livak and T. D. Schmittgen, Methods, 2001, 25, 402–408 CrossRef CAS PubMed
. - H. B. Wang, P. Y. Wang, X. Wang, Y. L. Wan and Y. C. Liu, Dig. Dis. Sci., 2012, 57, 3126–3135 CrossRef CAS PubMed
. - P. C. H. Hollman, M. G. L. Hertog and M. B. Katan, Food Chem., 1996, 57, 43–46 CrossRef CAS
. - I. B. Jaganath, W. Mullen, C. A. Edwards and A. Crozier, Free Radical Res., 2006, 40, 1035–1046 CrossRef CAS PubMed
. - S. Roowi, W. Mullen, C. A. Edwards and A. Crozier, Mol. Nutr. Food Res., 2009, 53, S68–S75 CrossRef PubMed
. - S. C. Marks, W. Mullen, G. Borges and A. Crozier, J. Agric. Food Chem., 2009, 57, 2009–2015 CrossRef CAS PubMed
. - A. F. Almeida, G. I. A. Borge, M. Piskula, A. Tudose, L. Tudoreanu, K. Valentová, G. Williamson and C. N. Santos, Compr. Rev. Food Sci. Food Saf., 2018, 17, 714–731 CrossRef CAS
. - S. Zhang, R. Wang, Y. Zhao, F. S. Tareq and S. Sang, Mol. Nutr. Food Res., 2019, 1900203 CrossRef PubMed
. - H. Tsuchiya, Food Chem., 2010, 120, 1089–1096 CrossRef CAS
. - P. I. Oteiza, A. G. Erlejman, S. V. Verstraeten, C. L. Keen and C. G. Fraga, Clin. Dev. Immunol., 2005, 12, 19–25 CrossRef CAS PubMed
. - F. E. Ward, D. L. Garling, R. T. Buckler, D. M. Lawler and D. P. Cummings, J. Med. Chem., 1981, 24, 1073–1077 CrossRef CAS PubMed
. - K. A. Ohemeng, C. F. Schwender and K. P. Fu, Bioorg. Med. Chem. Lett., 1993, 3, 225–230 CrossRef CAS
. - J. J. Hilliard, H. M. Krause, J. I. Bernstein, J. A. Fernandez, V. Nguyen, K. A. Ohemeng and J. F. Barrett, Adv. Exp. Med. Biol., 1995, 390, 59–69 CAS
. - A. Plaper, M. Golob, I. Hafner, M. Oblak, T. Solmajer and R. Jerala, Biochem. Biophys. Res. Commun., 2003, 306, 530–536 CrossRef CAS PubMed
. - J. S. Barnes, F. W. Foss and K. A. Schug, J. Am. Soc. Mass Spectrom., 2013, 24, 1513–1522 CrossRef CAS PubMed
. - L. R. Howard, R. L. Prior, R. Liyanage and J. O. Lay, J. Agric. Food Chem., 2012, 60, 6678–6693 CrossRef CAS PubMed
. - E. Pawlowska, J. Szczepanska, A. Koskela, K. Kaarniranta and J. Blasiak, Oxid. Med. Cell. Longevity, 2019, 2109, 1–13 Search PubMed
. - S. Birtic, S. Régis, C. Le Bourvellec and C. M. G. C. Renard, Food Chem., 2019, 296, 142–149 CrossRef CAS PubMed
. - T. H. Stanley, C. B. Van Buiten, S. A. Baker, R. J. Elias, R. C. Anantheswaran and J. D. Lambert, Food Chem., 2018, 255, 414–420 CrossRef CAS PubMed
. - B. W. Bolling, J. B. Blumberg and C. Y. Oliver Chen, Food Chem., 2010, 123, 1040–1047 CrossRef CAS PubMed
. - A. Srivastava, C. C. Akoh, W. Yi, J. Fischer and G. Krewer, J. Agric. Food Chem., 2007, 55, 2705–2713 CrossRef CAS PubMed
. - B. M. Schmidt, J. W. Erdman Jr and M. A. Lila, J. Food Sci., 2005, 70, S389−S394 Search PubMed
. - J. Shi and X. H. Zhao, Food Funct., 2019, 10, 2010–2021 RSC
. - L. Wang, E. T. Chiang, J. T. Simmons, J. G. N. Garcia and S. M. Dudek, Eur. Respir. J., 2011, 38, 78–88 CrossRef CAS PubMed
. - K. M. Argraves, P. J. Gazzolo, E. M. Groh, B. A. Wilkerson, B. S. Matsuura, W. O. Twal, S. M. Hammad and W. S. Argraves, J. Bio. Chem., 2008, 283, 25074–25081 CAS
. - Q. Yin, Y. Xia and G. Wang, Biochem. Biophys. Res. Commun., 2016, 477, 881–886 CrossRef CAS PubMed
. - K. Wang, X. Jin, Y. Chen, Z. Song, X. Jiang, F. Hu, M. A. Conlon and D. L. Topping, Nutrients, 2016, 8, e272 CrossRef PubMed
. - X. Wang, X. Zhao, T. Feng, G. Jin and Z. Li, Planta Med., 2016, 82, 1252–1257 CrossRef CAS PubMed
. - A. Hall, Biochem. Soc. Trans., 2012, 40, 1378–1382 CrossRef CAS PubMed
. - A. I. Ivanov, C. A. Parkos and A. Nusrat, Am. J. Pathol., 2010, 177, 512–524 CrossRef CAS PubMed
. - F. Wang, B. T. Schwarz, W. V. Graham, Y. Wang, L. Su, D. R. Clayburgh, C. Abraham and J. R. Turner, Gastroenterology, 2006, 131, 1153–1163 CrossRef CAS PubMed
. - S. Feng, L. Zou, H. Wang, R. He, K. Liu and H. Zhu, Molecules, 2018, 23, e2371 CrossRef PubMed
. - G. Yang, S. Bibi, M. Du, T. Suzuki and M. J. Zhu, Crit. Rev. Food Sci. Nutr., 2016, 57, 3830–3839 CrossRef PubMed
.
|
This journal is © The Royal Society of Chemistry 2020 |
Click here to see how this site uses Cookies. View our privacy policy here.