DOI:
10.1039/D0RA04280C
(Paper)
RSC Adv., 2020,
10, 22257-22263
Photodynamic properties of tungsten iodide clusters incorporated into silicone: A2[M6I8L6]@silicone†
Received
4th March 2020
, Accepted 20th May 2020
First published on 10th June 2020
Abstract
The light-induced antibacterial and antifungal properties of A2[M6I8L6] with M = Mo and W, A = organic cation, L = ligand have been studied. The photoactive compounds (TBA)2[W6I8(C7H7SO3)6] and (TBA)2[W6I8(COOCF3)6] have been incorporated into a permeable silicone matrix and were measured for their application in the decomposition of multi-resistant bioactive species (hospital germs) such as S. aureus and P. aeruginosa as well as fungi. In addition, we present a new high volume synthesis route for these types of cluster compounds departing from the soluble compound W6I22.
Introduction
A large number of binary tungsten iodide clusters has been recently reported, of which the well-known W6I12 with an octahedral cluster core is the most stable one.1 The connectivity between clusters in the layered structure of W6I12 preclude its solubility and thus any solution chemistry, in contrast to the [W6I14]2− ion.
A2[W6X8iX6a] type compounds with A = organic cation, X = Cl, Br and I were reported for their versatile photophysical properties.2–9 There structures are based on an octahedral tungsten cluster with eight μ3-bridging (or i = inner) and six terminal (or a = outer) halide ligands over the corners of the cluster core.
Ligand substituted clusters of the type A2[W6X8L6] with L being an organic or inorganic ligand, can be synthesized by ligand exchange reactions in solution, mostly performed with silver salts, e.g. Ag(C7H7SO3) to yield A2[W6X8(C7H7SO3)6].
Upon excitation by means of UV/vis radiation, all these clusters emit light with a broad emission band in the deep red region of the visible spectrum (650–700 nm). As a competing process, the luminescence (in fact phosphorescence) is quenched in the presence of molecular oxygen.10 The quenching process involves energy transfer from cluster triplet states to ground-state oxygen (X3Σg). The quantum yield of the O2 (a1Δg) emission can be determined via the characteristic 1275 nm phosphorescence.11 The dependency of the photoluminescence intensity with the oxygen partial pressure is fully reversible, qualifying this material type to sense oxygen.12,13
Similar properties are reported for basic compounds A2[W6X8X6]. However, they show luminescence quenching by molecular oxygen, respectively singlet oxygen generation in solution, but not in solid state. Among mixed A2[W6X8X6] clusters with X = Cl, Br, I the all-iodide compounds are of particular interest because they reveal the highest quantum yields.14
Preparations of A2[W6X8L6] require several steps, following some standard procedures. The bottle neck of the reaction chain involves the transformation of (insoluble) Cs2[W6I8I6] into A2[W6I8I6] in which A typically is TBA (n-tetrabutylammonium). In this work we present a new synthesis route departing from W6I22, allowing for an efficient high-volume synthesis of A2[W6I8L6] compounds.
These compounds as well as molybdenum analogues A2[Mo6I8L6] have been subject of extensive research including phosphorescence,15 disinfection,16 photodynamic therapy17 and photodynamic reduction.18,19 The photodynamic inactivation of bacteria or cells is mostly limited by the poor cellular uptake without the use of carriers, but there is an emerging research to address this problem.20
For antibacterial and antifungal studies performed in this work, compounds are incorporated into silicone matrices. Tungsten iodide compounds (TBA)2[W6I8(C7H7SO3)6] and (TBA)2[W6I8(COOCF3)6] are selected because their photophysical properties were well studied and they are known to behave sufficiently stable in moist air. A corresponding molybdenum iodide compound is also employed.
Results and discussion
Several routes have been reported to synthesize ternary tungsten iodides.21–23 A limiting step in the synthesis of ligand-substituted cluster compounds is the transformation of insoluble Cs2[W6I8I6] into the soluble precursor (TBA)2[W6I8I6] by cation exchange, because this step does not proceed in solution but from a slurry of Cs2[W6I8I6] powder.24 (TBA)2[W6I8I6] is then reacted with a corresponding silver salt (e.g. Ag(C7H7SO3), Ag(COOCF3), etc.) to replace the six terminal iodido ligands to yield (TBA)2[W6I8L6] (Fig. 1). Following a new reactions strategy we have employed soluble W6I22
25 instead of Cs2[W6I14], allowing for a higher reaction rate and a high volume synthesis (≥5 g) of (TBA)2[W6I8I6] and finally the ligand-substituted cluster.
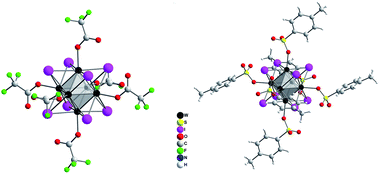 |
| Fig. 1 View of used cluster anions [W6I8(COOCF3)6]2− (left) and [W6I8(C7H7SO3)6]2− (right). | |
The luminescence spectrum of such microcrystalline (TBA)2[W6I8(C7H7SO3)6] exhibits a broad emission band at 678 nm (1.83 eV) from excited spin triplet states upon excitation with 400 nm radiation. The phosphorescence is quenched in the presence of oxygen. Hence, the phosphorescence intensity as well as the decay time decrease with increasing oxygen partial pressure. The decay time of (TBA)2[W6I8(C7H7SO3)6] in pure nitrogen is 24 μs and in pure oxygen 11.9 μs. Similar properties are observed for (TBA)2[W6I8(CF3COO)6] with a maximum emission at 660 nm (1.88 eV) and luminescence lifetimes of 29.2 μs and 9.9 μs. The corresponding molybdenum species (TBA)2[Mo6I8(C7H7SO3)6] shows an emission band at 676 nm (1.83 eV) and decay times of 147 μs and 64 μs in nitrogen and pure oxygen atmosphere.
Encapsulation of A2[M6I8L6] into silicone
Molybdenum and tungsten clusters of the type A2[M6I8L6] are insoluble in water and tend to hydrolyse in a humid environment. Therefore, for a possible application outside and inside medical applications the compounds either have to be equipped with an appropriate ligand or incorporated into a carrier. There are different approaches already described, the incorporation into functionalized polystyrene (PS-SH, PS-Py, PS-COOH) microspheres26–28 where the labile NO3− ligand is substituted for a polymer matrix or for biological applications into mitochondrion20 to prevent degeneration. In case of [Mo6I8@PS-SH], however, this compound does not show luminescence quenching by neither solvent nor oxygen and therefore no singlet oxygen generation. Another approach is the incorporation into fluorinated matrices to increase oxygen diffusion and therefore singlet oxygen production.29 Since we are aiming for biological applications we incorporated the cluster compounds into a silicone matrix (Fig. 2), thereby maintaining the production of singlet oxygen, to measure the dark- and photo-induced antibacterial and antifungal activities of these materials.
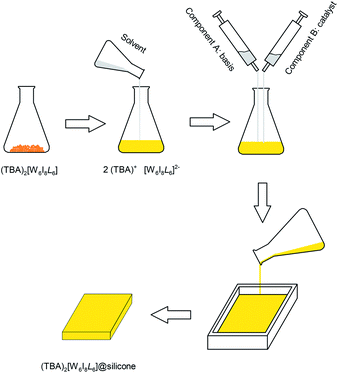 |
| Fig. 2 Procedure for the incorporation of (TBA)2[W6I8L6] into silicone. | |
Dimethyl silicone is one of the permeable elastomers available.30 Common usage for this kind of polymer are in blood oxygenation, gas separation and drug delivery.31 We use a common available two component RTV silicone (room temperature vulcanization) which offers great oxygen permeability due to the high flexibility of the oxygen–silicone chains which create openings and holes in the matrix for gas diffusion (570 cm3 mm m−2 h−1 bar−1 at room temperature). However, this flexibility is dependent on the polarity of the residues attached to the Si–O skeleton, in the latter case methyl. As a catalyst for the polymerization, either a diacetyl peroxide or acetic acid is used. In our case a platinum cross-linker is used, which is common especially in medical products because they produce less unwanted side products.32 Another advantage of elastomers is the fact that it is possibly to create almost any imaginable shapes. The respective cluster compound was incorporated by dissolution in dichloromethane under dissociation into TBA+ ions and [M6I8L6]2− clusters. Solidification of the matrix starts on adding the second component (see Experimental section). Fig. 3 shows an example of (TBA)2[W6I8(C7H7SO3)6] incorporated into silicone.
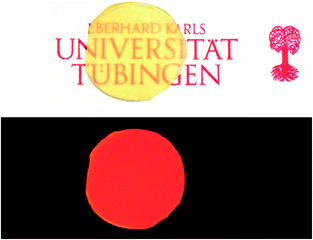 |
| Fig. 3 Photograph of a circular cut silicone disc (adopted from Fig. 2) implanted with (TBA)2[W6I8(C7H7SO3)6] under daylight (top) and under UV irradiation (bottom, 366 nm). | |
Luminescence of A2[M6I8L6]@silicon
Clusters incorporated into silicone (A2[M6I8L6]@silicone) show similar broad excitation and emission bands as in solid state or solution. Crystalline samples of ligand substituted compounds [W6I8iL6a]2− show luminescence quenching rates in the order of 50% for L = tosylate and 66% for L = trifluoroacetate (Fig. 4A and B).10,33 The dependency of the emission, i.e. luminescence quenching rate with the oxygen partial pressure can be regarded as a measure for the singlet oxygen production rate. The recorded spectra of clusters incorporated into silicone are showing only smaller deviations to those of the respective crystalline powders (36% and 57%, respectively). Monitored phosphorescence quantum yields (ΦP) tend to increase in case of (TBA)2[W6I8(C7H7SO3)6] from 0.028 in solution to 0.14 @silicone and for (TBA)2[W6I8(COOCF3)6] from 0.015 in solution to 0.21 @silicone. This is not unexpected because it has been shown that oxygen quenching is always more efficient in solution. (TBA)2[Mo6I8(C7H7SO3)6] did not show a significant difference in the quantum yield after being incorporated into silicone.
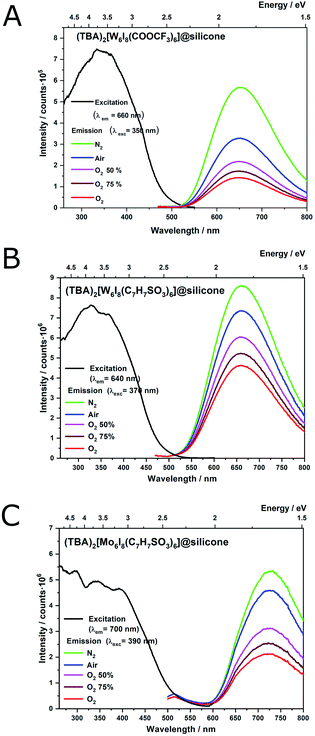 |
| Fig. 4 Excitation and emission spectra of (TBA)2[W6I8(COOCF3)6] (A), (TBA)2[W6I8(C7H7SO3)6] (B), and (TBA)2[Mo6I8(C7H7SO3)6] (C) encapsulated into silicone, recorded under various O2 partial pressures, ranging from 0 mbar O2 (in N2) to 1013 mbar O2 (pure O2). | |
The photoluminescence spectrum of (TBA)2[Mo6I8(C7H7SO3)6]@silicone (Fig. 4C) shows a significant shift in the emission maximum. While a maximum emission is expected at around 660 to 670 nm for (TBA)2[Mo6I8(C7H7SO3)6], a red shift towards 731 nm is observed. The lifetimes of excited states decrease from 147 μs (solid state) to 47 μs (@silicone), suggesting some kind of transformation of the cluster compound. This finding is most likely due to hydrolysis of the cluster, respectively substitution of terminal ligands to yield aqua hydroxo complexes, as has been described in previous literature.34 This degradation is significantly faster in the case of molybdenum compared to tungsten cluster compounds.34 The same behaviour is likely here in the sense that the clusters@silicone are “diluted” into the silicone matrix which contains traces of oxygen and water and is furthermore permeable for small molecules and gases.30 Hydrolysed and aqua complexes tend to show a significant decrease in phosphorescence intensity (due to non-radiative deactivation by water), including a red shift in the emission spectrum.17 Luminescence spectra of tungsten compounds remained more or less the same in solid state, solution and in silicone (Table 1).
Table 1 Luminescence properties of (TBA)2[W6I8(C7H7SO3)6], (TBA)2[Mo6I8(C7H7SO3)6] and (TBA)2[W6I8(COOCF3)6] in solid state, in solution, and after incorporation into silicone
Compound |
Emission maximum in/nm |
τ1/2/μs |
N2/O2a quenching |
ΦP |
Powder |
Pure N2 |
Air |
Pure O2 |
Air |
N2/O2 quenching: luminescence quenching when switching the atmosphere from N2 to O2. |
(TBA)2[W6I8(COOCF3)6] |
In solid state10 |
660 |
29.2 |
|
9.9 |
66.1% |
0.04 |
In solution10 |
677 |
36 |
1.08 |
0.23 |
99.4% |
0.015 |
@silicone |
651 |
30.2 |
|
12.9 |
57.3% |
0.21 |
![[thin space (1/6-em)]](https://www.rsc.org/images/entities/char_2009.gif) |
(TBA)2[W6I8(C7H7SO3)6] |
In solid state33 |
678 |
24 |
|
11.9 |
50.4% |
|
In solution33 |
675 |
40.2 |
1.94 |
0.91 |
97.7% |
0.028 |
@silicone |
660 |
28 |
24 |
18 |
35.7% |
0.14 |
![[thin space (1/6-em)]](https://www.rsc.org/images/entities/char_2009.gif) |
(TBA)2[Mo6I8(C7H7SO3)6] |
In solid state35 |
676 |
147 |
111 |
64 |
56.5% |
|
In solution35 |
671 |
232 |
4.94 |
2.11 |
99.1% |
0.011 |
@silicone |
731 |
47 |
38 |
27 |
42.6% |
0.02 |
Antibacterial and antifungal studies of the silicone materials
An evaluation of antimicrobial photodynamic inactivation (PDI)36 activity on the surface of the neat silicone and the cluster-doped materials on Gram-negative E. coli, S. typhimurium, P. aeruginosa and on Gram-positive S. aureus bacteria was investigated. Also, an antifungal photodynamic inactivation activity on C. albicans fungi was evaluated. Microorganism-infected samples were then irradiated with a white spot-light source (400–800 nm) for 10 min (Fig. 5). Data obtained (Table 2) shows that (TBA)2[W6I8(COOCF3)6]@silicone and (TBA)2[W6I8(C7H7SO3)6]@silicone exhibit noticeable light-induced antimicrobial activity, while (TBA)2[Mo6I8(C7H7SO3)6]@silicone and neat silicone have very slight activity against a negative control i.e. neat silicon that was not exposed to light. Since the luminescence quenching by oxygen for (TBA)2[W6I8(COOCF3)6] (57.3%) is highest among investigated, (TBA)2[Mo6I8(C7H7SO3)6] (42.6%) and (TBA)2[W6I8(C7H7SO3)6] (35.7%), is assumed that the PDI efficacy has the same order. However, it was unexpected that the PDI efficacy for (TBA)2[W6I8(C7H7SO3)6]@silicone was higher than for others, while molybdenum one has worst PDI activity. The possible reason of this behaviour could be the difference in the singlet oxygen lifetime due to the different self-quenching effect that was previously demonstrated on the hexacyanido rhenium cluster complexes.37 Moreover, it was previously reported that octahedral iodide tungsten cluster complexes [W6I8L6]2− under illumination can produce not only singlet oxygen but also superoxide ion O2−.38 Such reactive oxygen radical has significantly higher lifetime than singlet oxygen (minutes vs. microseconds in aqueous media),39,40 and hence may have a longer effect on microorganisms.
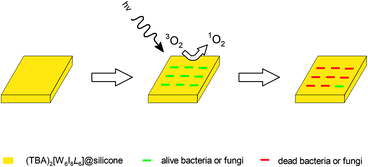 |
| Fig. 5 Schematic overview of the antibacterial properties of cluster@silicone. | |
Table 2 The percentage of colony-forming units in comparison to control for the cluster doped silicon after irradiation with a white light source. The confidence interval is calculated for P = 0.95
Sample |
S. aureus |
E. coli |
S. typhimurium |
P. aeruginosa |
C. albicans (Fungi) |
Negative control |
100 ± 7 |
100 ± 6 |
100 ± 8 |
100 ± 11 |
100 ± 3 |
Neat silicone |
89.9 ± 7.1 |
99.4 ± 3.9 |
93.1 ± 4.8 |
88.0 ± 4.4 |
82.5 ± 6.8 |
(TBA)2[W6I8(COOCF3)6]@silicone |
17.8 ± 2.4 |
1.7 ± 0.3 |
1.09 ± 0.05 |
9.2 ± 0.9 |
4.6 ± 0.5 |
(TBA)2[W6I8(C7H7SO3)6]@silicone |
0.89 ± 0.09 |
0.8 ± 0.1 |
0.93 ± 0.05 |
2.1 ± 0.4 |
2.6 ± 0.2 |
(TBA)2[Mo6I8(C7H7SO3)6]@silicone |
99.7 ± 4.5 |
90.2 ± 9.8 |
83.6 ± 4.8 |
67.6 ± 8.5 |
90.5 ± 5.4 |
These both possibilities may be the reason for such a low PDI activity for molybdenum material (TBA)2[Mo6I8(C7H7SO3)6]@silicone that comparable to PDI for the neat silicone. The presented data for bacterial cultures is in good agreement with earlier reported for cluster-doped fluoroplast F-32L29 i.e. the most resistant microorganisms are Gram-positive S. aureus and Gram-negative P. aeruginosa. Nevertheless, (TBA)2[W6I8(C7H7SO3)6]@silicone demonstrated significant PDI activity even for S. aureus and P. aeruginosa with a microorganisms proliferation inhibition up to 98%. The data of antifungal PDI activity on cluster-based materials are presented for the first time.
Conclusions
(TBA)2[W6I8L6] cluster compounds are known for their photophysical properties including their activity as efficient singlet oxygen sensitizers with atmospheric oxygen. The employment of singlet oxygen as a residue-free agent in the neutralization of bacteria, fungi or even viruses is a great challenge.
Cluster compounds under consideration belong to the small group of inorganic singlet oxygen sensitizers which remain widely intact in the presence of singlet oxygen, compared to the multitude of organic counterparts. Within the current exploration we implanted cluster compounds into a silicon basin to explore their light-induced antimicrobial activity. Composite (TBA)2[W6I8L6]@silicon materials are showing good chemical and photochemical stabilities with similar properties as their respective crystalline powders. (TBA)2[W6I8(COOCF3)6]@silicone and (TBA)2[W6I8(C7H7SO3)6]@silicone exhibit high light-induced antimicrobial activity against the sections of bacteria including multi-resistant hospital germs such as S. aureus and P. aeruginosa as well as fungi. The somewhat lower efficiency of (TBA)2[W6I8(COOCF3)6]@silicone could be attributed to a longer singlet oxygen lifetime.
Experimental Section
The preparation and structural characterization of (TBA)2[W6I8(C7H7SO3)6],33, (TBA)2[Mo6I8(C7H7SO3)6]33,41 and (TBA)2[W6I8(COOCF3)6]10 were already reported. Herein we present a high-volume synthesis for tungsten iodide phosphors with the [W6I8]-core, departing from W6I22 instead of Cs2[W6I14]. W6I22 was prepared according to the literature.1,42 Purities of products from individual reaction steps were explored by means of X-ray powder diffraction. W6I22 was obtained in a high-volume synthesis for the first time in high purity (see ESI†). The identity of compounds obtained therefrom, (TBA)2[W6I8(C7H7SO3)6] and (TBA)2[W6I8(COOCF3)6] was proven by comparison with single crystal data from literature and ESI-MS measurements.
Synthesis of (TBA)2[W6I8L6] with L = C7H7SO3, COOCF3
W6I22 (5 g, 1.28 mmol) was dissolved in acetone (100 ml, HPLC grade) and (TBA)I (0.47 g, 1.28 mmol) was added under air. The solution was stirred for one day and the solvent was left to evaporate at room temperature. The product was obtained as a dark green powder. Traces of iodine can be separated by column chromatography to obtain an orange powder.43 Ligand exchange reactions were performed by exchanging the terminal cluster ligands by adding silver p-toluene sulfonate or silver trifluoroacetate, respectively, according to the following equation on the example of (TBA)2[W6I8(C7H7SO3)6].33,41 All reactions (1–5) proceed in high yields (>95%). |
 | (1) |
|
 | (2) |
|
 | (3) |
|
 | (4) |
|
 | (5) |
Synthesis of (TBA)2[Mo6I8L6] with L = C7H7SO3
The synthesis was carried out according to literature33 procedure starting from a halide exchange with MoCl5 and SiI4 according to the following equations on the example of (TBA)2[W6I8(C7H7SO3)6].33,41,44 All reactions (6–10) proceed in high yields (>95%). |
 | (6) |
|
 | (7) |
|
 | (8) |
|
 | (9) |
|
 | (10) |
Synthesis of cluster@silicone composites
The silicone matrix was prepared from a commercial room temperature vulcanization silicone (RTV2 silicone SF33). Typically, 4 mg of (TBA)2[M6I8L6] with M = Mo, W and L = C7H7SO3, COOCF3 was dissolved into 1 ml dichloromethane (solution A). Solution A was mixed with 2 ml component basis and 2 ml component catalyst of silicone. The mixture was placed into a homemade casting box and slowly evacuated for 5–10 minutes to remove air bubbles from silicone. After 24 h the composite was removed. All silicone discs of A2[M6I8L6]@silicone appeared transparent with a yellow body colour (Fig. 3) typically obtained for the diluted solid (ground with BaSO4), or dissolved cluster compound.
Tungsten iodide clusters in silicone show very good stability. The maximum emission observed for (TBA)2[W6I8(C7H7SO3)6]@silicone only shifts within the error of the measurement, from 660 nm (1.88 eV) to 662 nm (1.87 eV) after six months. In the same timeframe the maximum emission of (TBA)2[Mo6I8(C7H7SO3)6]@silicone shows a shift from 731 nm (1.70 eV) to 744 nm (1.67 eV) indicating an ongoing hydrolysis of the compound.
Luminescence measurements
Excitation and emission spectra on (TBA)2[M6I8L6]@silicone with M = Mo, W and L = C7H7SO3, COOCF3 were collected with a fluorescence spectrometer FLS920 (Edinburgh Instruments) equipped with a 450 W ozone-free xenon discharge lamp (OSRAM) and a cryostat “MicrostatN” from Oxford Instruments sample chamber installed with a mirror optic for powder samples. For detection, an R2658P single-photon-counting photomultiplier tube (Hamamatsu) was used. All luminescence spectra were recorded with a spectral resolution of 1 nm, a dwell time of 0.4 s in 1 nm steps and 3 repeats. Quantum yields were measured according to Yuichiro Kawamura45 upon excitation of 450 nm, 3 nm spectral resolution, while respective emission intensity from 420 nm to 870 nm with a spectral resolution of 0.5 nm with 0.5 nm steps was recorded.
Photodynamic inactivation
The antibacterial and antifungal photodynamic inactivation activity of the materials on Escherichia coli (ATCC25922), Staphylococcus aureus (ATCC25923), Salmonella typhimurium (ATCC14028) and Pseudomonas aeruginosa (ATCC27853) bacteria, and Candida albicans (ATCC10231) fungi was tested. The bacteria and fungi were cultivated in Lysogeny broth (LB) medium at 37 °C. Microorganisms diluted in saline at concentrations of 1–1.2 × 106 CFU ml−1 (CFU – colony-forming units) were used in the experiments. The number of viable microorganisms was estimated via counting of the colony-forming units (CFU) after 24 h of cultivation. All experiments were performed in triplicate with a confidence interval for P = 0.95.
All samples were cut into strips with lateral size of 0.5 cm × 2.0 cm. The strips were coated into a solution with microorganisms, then they were placed on a dry surface of the Petri dishes and irradiated with a spot light source L8253 (Hamamatsu) at a distance of 20 cm for 10 min (400–800 nm, 220 mW cm−2) for each side, i.e. the strips were transferred to a new dry dish and turned over to process the second side. For the negative control, all the procedures were similarly performed, but the coated strips were kept in the dark for the same time. After irradiation, strips were placed into test tubes and suspended with 1000 μL of saline for 1 min. 1000 μL of the culture media was collected from the test tube and cultivated in LB agar culture media. The number of colonies were counted after 24 h. Fig S5† shows images of the microorganisms' growth in LB agar culture media.
Electron spray ionisation (ESI) measurements
All ESI-MS measurements were performed on a Bruker Esquire 3000 plus Ion Trap LC/MS system in positive mode in methanol solution. ESI-MS: (TBA)2[W6I8(CF3COO)6] revealed m/z = 3037.6 for (TBA)[W6I8(CF3COO)6]−; theoretical values: m/z = 3038.8 for (TBA)[W6I8(CF3COO)6]−. ESI-MS: (TBA)2[W6I8(C7H7SO3)6] revealed m/z = 1572.8 for [W6I8(O3SC7H7)6]2−; theoretical values: m/z = 1572.7 for [W6I8(O3SC7H7)6]2−.
Conflicts of interest
There are no conflicts to declare.
Acknowledgements
This work was supported by the Russian Foundation for Basic Research (grant number 19-53-12019) and the Deutsche Forschungsgemeinschaft (grant number ME914-31-1).
Notes and references
- M. Ströbele and H.-J. Meyer, Dalton Trans., 2019, 48, 1547–1561 RSC.
- D. Bublitz, W. Preetz and M. K. Simsek, Z. Anorg. Allg. Chem., 1997, 623, 1–7 CrossRef CAS.
- M. N. Sokolov, M. A. Mihailov, E. V. Peresypkina, K. A. Brylev, N. Kitamura and V. P. Fedin, Dalton Trans., 2011, 40, 6375–6377 RSC.
- M. N. Sokolov, M. A. Mikhailov, K. A. Brylev, A. V. Virovets, C. Vicent, N. B. Kompankov, N. Kitamura and V. P. Fedin, Inorg. Chem., 2013, 52, 12477–12481 CrossRef CAS PubMed.
- K. Kirakci, K. Fejfarova, M. Kucerakova and K. Lang, Eur. J. Inorg. Chem., 2014, 2014, 2331–2336 CrossRef CAS.
- O. A. Efremova, M. A. Shestopalov, N. A. Chirtsova, A. I. Smolentsev, Y. V. Mironov, N. Kitamura, K. A. Brylev and A. J. Sutherland, Dalton Trans., 2014, 43, 6021–6025 RSC.
- O. A. Efremova, Y. A. Vorotnikov, K. A. Brylev, N. A. Vorotnikova, I. N. Novozhilov, N. V. Kuratieva, M. V. Edeleva, D. M. Benoit, N. Kitamura, Y. V. Mironov, M. A. Shestopalov and A. J. Sutherland, Dalton Trans., 2016, 45, 15427–15435 RSC.
- M. A. Mikhailov, K. A. Brylev, P. A. Abramov, E. Sakuda, S. Akagi, A. Ito, N. Kitamura and M. N. Sokolov, Inorg. Chem., 2016, 55, 8437–8445 CrossRef CAS PubMed.
- M. A. Mikhaylov and M. A. Sokolov, Eur. J. Inorg. Chem., 2019, 2019, 4181–4197 CrossRef CAS.
- L. Riehl, A. Seyboldt, M. Ströbele, D. Enseling, T. Jüstel, M. Westberg, P. R. Ogilby and H.-J. Meyer, Dalton Trans., 2016, 45, 15500–15506 RSC.
- A. U. Khan and M. Kasha, J. Chem. Phys., 1963, 39, 2105–2106 CrossRef CAS.
- D. J. Osborn, G. L. Baker and R. N. Ghosh, J. Sol-Gel Sci. Technol., 2005, 36, 5–10 CrossRef CAS.
- R. N. Ghosh, P. A. Askeland, S. Kramer and R. Loloee, Appl. Phys. Lett., 2011, 98, 221103 CrossRef.
- T. C. Zietlow, D. G. Nocera and H. B. Gray, Inorg. Chem., 1986, 25, 1351–1353 CrossRef CAS.
- K. Kirakci, P. Kubat, M. Kucerakova, V. Sicha, H. Gbelcova, P. Lovecka, P. Grznarova, T. Ruml and K. Lang, Inorg. Chim. Acta, 2016, 441, 42–49 CrossRef CAS.
- A. Barras, S. Cordier and R. Boukherroub, Appl. Catal., B, 2012, 123, 1–8 CrossRef.
- D. V. Evtushok, A. R. Melnikov, N. A. Vorotnikova, Y. A. Vorotnikov, A. A. Ryadun, N. V. Kuratieva, K. V. Kozyr, N. R. Obedinskaya, E. I. Kretov, I. N. Novozhilov, Y. V. Mironov, D. V. Stass, O. A. Efremova and M. A. Shestopalov, Dalton Trans., 2017, 46, 11738–11747 RSC.
- M. Feliz, M. Puche, P. Atienzar, P. Concepcion, S. Cordier and Y. Molard, ChemSusChem, 2016, 9, 1963–1971 CrossRef CAS PubMed.
- P. Kumar, S. Kumar, S. Cordier, S. Paofai, R. Boukherroub and S. L. Jain, RSC Adv., 2014, 4, 10420–10423 RSC.
- K. Kirakci, J. Zelenka, M. Rumlova, J. Cvacka, T. Ruml and K. Lang, Biomater. Sci., 2019, 7, 1386–1392 RSC.
- J. D. Franolic, J. R. Long and R. H. Holm, J. Am. Chem. Soc., 1995, 117, 8139–8153 CrossRef CAS.
- M. A. Riche, Ann. Chim. Phys., 1857, 3, 5 Search PubMed.
- A. D. Westland and N. Muriithi, Inorg. Chem., 1973, 12, 2356–2361 CrossRef CAS.
- T. Hummel, M. Ströbele, D. Schmid, D. Enseling, T. Jüstel and H.-J. Meyer, Eur. J. Inorg. Chem., 2016, 2016, 5063–5067 CrossRef CAS.
- H. Schäfer and H. G. Schulz, Z. Anorg. Allg. Chem., 1984, 516, 196–200 CrossRef.
- K. Kirakci, P. Kubat, K. Fejfarova, J. Martincik, M. Nikl and K. Lang, Inorg. Chem., 2016, 55, 803–809 CrossRef CAS PubMed.
- N. A. Vorotnikova, M. V. Edeleva, O. G. Kurskaya, K. A. Brylev, A. M. Shestopalov, Y. V. Mironov, A. J. Sutherland, O. A. Efremova and M. A. Shestopalov, Polym. Int., 2017, 66, 1906–1912 CrossRef CAS.
- N. A. Vorotnikova, O. A. Efremova, A. R. Tsygankova, K. A. Brylev, M. V. Edeleva, O. G. Kurskaya, A. J. Sutherland, A. M. Shestopalov, Y. V. Mironov and M. A. Shestopalov, Polym. Adv. Technol., 2016, 27, 922–928 CrossRef CAS.
- N. A. Vorotnikova, A. Y. Alekseev, Y. A. Vorotnikov, D. V. Evtushok, Y. Molard, M. Amela-Cortes, S. Cordier, A. I. Smolentsev, C. G. Burton, P. M. Kozhin, P. Zhu, P. D. Topham, Y. V. Mironov, M. Bradley, O. A. Efremova and M. A. Shestopalov, Mater. Sci. Eng., C, 2019, 105, 110150 CrossRef CAS PubMed.
- W. L. Robb, Ann. N. Y. Acad. Sci., 1968, 146, 119–137 CrossRef CAS PubMed.
- E. L. Warrick, O. R. Pierce, K. E. Polmanteer and J. C. Saam, Rubber Chem. Technol., 1979, 52, 437–525 CrossRef CAS.
- L. N. Lewis, J. Stein, Y. Gao, R. E. Colborn and G. Hutchins, Platinum Met. Rev., 1997, 41, 66–75 CAS.
- A. Seyboldt, D. Enseling, T. Jüstel, M. Ivanovic, H. Peisert, T. Chasse and H.-J. Meyer, Eur. J. Inorg. Chem., 2017, 2017, 5387–5394 CrossRef CAS.
- E. V. Svezhentseva, Y. A. Vorotnikov, A. O. Solovieva, T. N. Pozmogova, I. V. Eltsov, A. A. Ivanov, D. V. Evtushok, S. M. Miroshnichenko, V. V. Yanshole, C. J. Eling, A. M. Adawi, J. S. G. Bouillard, N. V. Kuratieva, M. S. Fufaeva, L. V. Shestopalova, Y. V. Mironov, O. A. Efremova and M. A. Shestopalov, Chem.–Eur. J., 2018, 24, 17915–17920 CrossRef CAS PubMed.
- M. A. Mikhailov, A. L. Gushchin, M. R. Gallyamov, A. V. Virovets, M. N. Sokolov, D. G. Sheven and V. V. Pervukhin, Russ. J. Coord. Chem., 2017, 43, 172–180 CrossRef CAS.
- M. R. Hamblin, Curr. Opin. Microbiol., 2016, 33, 67–73 CrossRef CAS PubMed.
- A. O. Solovieva, K. Kirakci, A. A. Ivanov, P. Kubat, T. N. Poznaogova, S. M. Miroshnichenko, E. V. Vorontsova, A. V. Chechushkov, K. E. Trifonova, M. S. Fufaeva, E. I. Kretov, Y. V. Mironov, A. F. Poveshchenko, K. Lang and M. A. Shestopalov, Inorg. Chem., 2017, 56, 13491–13499 CrossRef CAS PubMed.
- J. A. Jackson, C. Turro, M. D. Newsham and D. G. Nocera, J. Phys. Chem., 1990, 94, 4500–4507 CrossRef CAS.
- M. Hayyan, M. A. Hashim and I. M. AlNashef, Chem. Rev., 2016, 116, 3029–3085 CrossRef CAS PubMed.
- E. Appiani, R. Ossola, D. E. Latch, P. R. Erickson and K. McNeill, Environ. Sci.: Process. Impacts, 2017, 19, 507–516 CAS.
- A.-D. Fuhrmann, A. Seyboldt, A. Schank, G. Zitzer, B. Speiser, D. Enseling, T. Jüstel and H.-J. Meyer, Eur. J. Inorg. Chem., 2017, 2017, 4259–4266 CrossRef CAS.
- M. Ströbele and H.-J. Meyer, Inorg. Chem., 2019, 58, 12867–12872 CrossRef PubMed.
- T. Hummel, M. Ströbele, A. D. Fuhrmann, D. Enseling, T. Jüstel and H.-J. Meyer, Eur. J. Inorg. Chem., 2019, 2019, 4014–4019 CrossRef CAS.
- M. Ströbele, R. Thalwitzer and H.-J. Meyer, Inorg. Chem., 2016, 55, 12074–12078 CrossRef PubMed.
- Y. Kawamura, H. Sasabe and C. Adachi, Jpn. J. Appl. Phys., 2004, 43, 7729–7730 CrossRef CAS.
Footnote |
† Electronic supplementary information (ESI) available: Fig. S1–S3; emission integrals and decay times of clusters incorporated into silicone. Fig. S4; X-ray pattern of W6I22. Fig. S5; photographs of microorganisms' growth in LB agar culture media. See DOI: 10.1039/d0ra04280c |
|
This journal is © The Royal Society of Chemistry 2020 |
Click here to see how this site uses Cookies. View our privacy policy here.