DOI:
10.1039/D0RA04392C
(Paper)
RSC Adv., 2020,
10, 25155-25164
Recyclable MFe2O4 (M = Mn, Zn, Cu, Ni, Co) coupled micro–nano bubbles for simultaneous catalytic oxidation to remove NOx and SO2 in flue gas
Received
17th May 2020
, Accepted 20th June 2020
First published on 2nd July 2020
Abstract
NOx can be efficiently removed by micro–nano bubbles coupling with Fe3+ and Mn2+, but the catalyst cannot be reused and the adsorption wastewater should be treated. This work developed a new technology that uses micro–nano bubbles and recyclable MFe2O4 to simultaneously remove NOx and SO2 from flue gas, and clarified the effectiveness and reaction mechanism. MFe2O4 (M = Mn, Zn, Cu, Ni and Co) prepared by a hydrothermal method was characterized. The results show that MFe2O4 can be activated to produce ˙OH which can accelerate the oxidation absorption of NOx. Compared with no catalyst, the NOx conversion rate increased from 32.85% to 83.88% in the NOx–SO2–MFe2O4-micro–nano bubble system, while the removal rate of SO2 can reach 100% at room temperature. The catalytic activities of MFe2O4 showed the following trend: CuFe2O4 > ZnFe2O4 > MnFe2O4 > CoFe2O4 > NiFe2O4. The results provide a new idea for the application of advanced oxidation processes in flue gas treatment.
1. Introduction
With the development of industry, nitrogen oxides (NOx) and sulfur dioxide (SO2) have become the most common atmospheric pollutants.1,2 The emission of these pollutants causes various environmentally harmful effects such as acid rain, photochemical smog, ozone depletion and climate warming.3 At present, the main methods to control NOx in industry are dry denitration technology and wet denitration technology. Dry denitrification techniques include selective catalytic reduction (SCR) and selective non-catalytic reduction (SNCR).4–6 Wet denitrification techniques include oxidation, complexation absorption and acid–base absorption.7–9 There are many kinds of pollutants in the actual flue gas, which make it difficult to deal with. It is a new trend to study the oxidation and absorption of various pollutants in flue gas by simple devices.10 Flue gas desulfurization and denitrification integration refers to the process of simultaneous desulfurization and denitrification in the same equipment. Although this method can effectively improve the flue gas treatment efficiency and reduce the energy consumption, the main research is still in the research and development stage, there is still a long way to go before the large-scale industrialization. Micro–nano bubbles (MNB) coupled oxygen carrier (OCs) technology is a wet flue gas integrated removal technology, which can simplify the system, reduce the cost, and ensure a high removal efficiency of NOx and SO2,11,12 and has a strong application potential. It is a new direction of the comprehensive removal of multi-pollutant flue gas.13
Micro–nano bubble technology refers to the bubble with the diameter between tens of microns to hundreds of nanometers when the bubble occurs.14 Compared with ordinary bubbles, it has the following characteristics: small size leads to large surface area, low rising speed, high internal pressure, large gas solubility, and free radicals formed when crushed.13,15 Hydroxyl radicals generated by the breakdown of micro–nano bubbles are an important reactive oxygen species with an oxidation potential of 2.8V.16–18 Xiao19 et al. used micro–nano bubbles to remove SO2 and NO (1000 mg m−3), and NO removal efficiency reached 87.7%. It is well known that Fe2+ ions can promote the interaction between NOx and S(IV) in aqueous solution to form nitrosyl complexes, which lead to enhanced NOx removal.20,21 Although the activation of transition metals can accelerate the oxidative absorption of NOx, it is not suitable for recycling, and the product is heavy metal wastewater that causes secondary pollution. Therefore, it is necessary to use magnetic solid catalyst instead of ionic catalyst.
Previous studies have shown that spinel ferrite (chemical formula: M2+Fe23+O4) has better mechanical stability, stronger mechanical strength, reoxidation ability and environmental friendliness than single metal oxide.22–24 In actual use, MFe2O4 have acted as an oxygen supply and a catalyst.25,26 The standard spinel structure is a lattice composed of 32 divalent oxygen ions that form a closed-pack, face-centered cubic arrangement with 64 tetrahedral interstitial sites and 32 octahedral interstitial sites.27 In the spinel structure, M2+ and Fe3+ are respectively located in the sites of tetrahedron or octahedron composed of oxygen and have metal synergistic effects which determine good oxygen transferability and catalysis properties.28,29 Therefore, spinel ferrites were attractive candidates because of their rich crystal chemistry, low cost, nontoxicity and environmentally friendly properties.30,31 Xu et al.32 found that a small quantity of palladium-doped ferrite spinel could achieve high NO conversion and the catalyst cost reduction. However, the simultaneous use of transition metal oxide and MNB for NOx removal in a system has rarely been reported. Furthermore, the synergistic effects of the different metals in ferrites for NO and SO2 removal have been rarely mentioned.
In this study, the recyclable catalyst MFe2O4 (M = Mn, Co, Ni, Cu, Zn) synthesized with hydrothermal method and MNB were used to form the NOx–SO2–MFe2O4–MNB system for simultaneous removal of NOx and SO2 from flue gas. The structural, physical, and chemical properties of the as-prepared MFe2O4 before and after the reaction were characterized by X-ray diffraction (XRD), scanning electron microscopy (SEM), the Brunauer–Emmett–Teller (BET) method and X-ray photoelectron spectroscopy (XPS). The removal rates of NO and SO2 were monitored with the flue gas analyzer and ion chromatography to explore the actions of MNB and the effects of various divalent cations contained in the ferrite. The goal of this study was to evaluate the simultaneous NO and SO2 removal efficiencies, and elucidate the chemistry and reaction pathways of the NOx–SO2–MFe2O4–MNB system.
2. Experimental
2.1. Materials
All the materials used for synthesizing catalysts, including ethylene glycol, poly(ethylene glycol) (PEG), sodium acetate (CH3COONa), ferric chloride (FeCl3·6H2O), manganese chloride (MnCl2·H2O), zinc chloride (ZnCl2), cupric chloride (CuCl2·2H2O), nickel chloride (NiCl2·6H2O), cobalt chloride (CoCl2·6H2O), ethyl alcohol (C2H6O), 5,5-dimethyl-1-pyrroline N-oxide (DMPO), were purchased from Sinopharm Chemical Reagent Co., Ltd. All chemicals were used without further purification.
2.2. Synthesis of MFe2O4 (M = Mn, Co, Ni, Cu and Zn) by the hydrothermal method
The MFe2O4 (M = Mn, Co, Ni, Cu and Zn) microspheres were synthesized based on the hydrothermal method. Briefly, polyethylene glycol and sodium acetate were added into ethylene glycol under vigorous stirring conditions. Then partial chloride was added to reach the metal cation (Fe3+ and M2+) molar ratio of 2
:
1. The resultant homogeneous solution was obtained and then placed into the 500 mL Teflon-lined autoclave, reacted at 200 °C for 10 h. The black product was collected with a magnet, washed several times by ethanol and distilled water, and then dried at 80 °C for 6 h under vacuum. Finally, the resulting product was sufficiently grinded to obtain fresh MFe2O4 (M = Mn, Co, Ni, Cu and Zn).
2.3. Characterization
A scanning electron microscope (SEM, Hitachi S4800) was adopted to observe the morphologies. Energy dispersive spectrometer (EDS) with Horiba 7593-H was used to confirm the proportion of each element in the catalysts. Powder X-ray diffraction meter (XRD, D/max 2550 PC, Japan Rigaku) operation was completed with Cu Kα radiation in a 2θ angle between 10° and 80° at a rate of 2° min−1 to monitor crystal structure. The surface properties and valence state of the catalysts were determined through X-ray photoelectron spectroscopy (XPS) experiments by using the Escalab 250Xi spectrometer with an Al-Kα radiation source at an energy step size of 1 eV to obtain high-resolution XPS spectra. The spectra were calibrated with respect to C 1s at a binding energy of 284.8 eV. The specific surface area of the samples was determined by using the Brunauer–Emmett–Teller (BET) method on an automated area and pore size analyzer (Autosorb-iQ) based on nitrogen adsorption desorption isotherm. The samples were outgassed at 200 °C for 2 h to remove remaining moisture and then analyzed with N2 gas as an adsorbent at the temperature of liquid nitrogen. ˙OH and ˙O2− in the NOx–SO2–MFe2O4–MNB system were detected via electron paramagnetic resonance spectroscopy (EPR, JEOL-FA200), and DMPO was used for capturing ˙OH and ˙O2−.
2.4. Reactivity measurement in the NOx–SO2–MFe2O4–MNB system
Fig. 1 shows the NOx–SO2–MFe2O4–MNB system installation diagram. Firstly, NOx and SO2 in the cylinder are fed into the mixer in a certain proportion to mix evenly. Gas flow rate is controlled by the rotameter. The flue gas analyzer (Testo350) was adopted to observe the intake gas concentration. Then a certain amount of MFe2O4 is uniformly dispersed in the absorption solution by ultrasonic oscillation. Finally, the configured gas and absorption solution are passed through the micro–nano bubble generator together to form the NOx–SO2–MFe2O4–MNB system in the reaction tower, so as to complete the catalytic oxidation and absorption process of NOx and SO2. The removal efficiency of NOx was calculated by the concentrations of NO2− and NO3− in the absorption liquid measured with ion chromatography (ICS-1100 AS11-HC, Thermo Fisher Scientific).
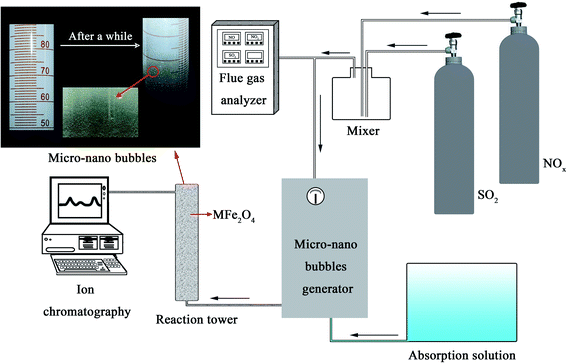 |
| Fig. 1 The NOx–SO2–MFe2O4–MNB system installation diagram. | |
The rated parameters of the micro–nano bubble generator were set as follows: the effluent pressure (0.5 MPa), the inlet water flow (300 mL min−1) and the intake air amount (48 mL min−1). The volume of the oxidation absorption reactor is 1.5 L. MFe2O4 was uniformly dispersed in a tubular reactor as a catalyst. A gas mixture containing 5113 mg m−3 NOx and 3371 mg m−3 SO2 was used as the intake gas. NOx is mainly composed of 3795 mg m−3 NO and 2021 mg m−3 NO2. In order to explore the practical application performance of the NOx–SO2–MFe2O4–MNB system, the effects of the addition of different recyclable MFe2O4 catalysts on the integrated desulfurization and denitrification efficiency were discussed by analyzing the IC test results of the absorption solution.
2.5. Analysis methods
The removal efficiency of NOx can be calculated as |
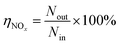 | (1) |
|
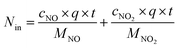 | (2) |
|
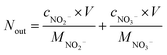 | (3) |
where ηNOx represents the removal efficiency of NOx, Nout is the amount of NO2− and NO3− in the absorption liquid, Nin is the amount of NOx entering the system, cNO and cNO2 are the concentrations of NO and NO2 in the intake air respectively,
are the concentrations of NO2− and NO3− in the absorption liquid respectively, q is the air inflow, V is the volume of water in the rection tower, M is the molecular weight, t is the system running time.
The removal efficiency of SO2 can be calculated as
|
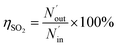 | (4) |
|
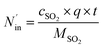 | (5) |
|
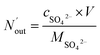 | (6) |
where
ηSO2 represents the removal efficiency of SO
2,

is the amount of SO
42− in the absorption liquid,

is the amount of SO
2 entering the system,
cSO2 are the concentrations of SO
2 in the intake air,

are the concentrations of SO
42− in the absorption liquid.
3. Results and discussion
3.1. Physicochemical characterization
The SEM images of MFe2O4 (M = Mn, Zn, Cu, Ni and Co) are shown in Fig. 2. The five spinel ferrites are similar to each other, exhibiting a spherical structure with a dense surface and abundant fine particles. Among the five OCs, CuFe2O4 and CoFe2O4 exhibited more agglomeration and sintering, possibly due to high temperature calcination and the small-dimension effect. Compared with CuFe2O4 and CoFe2O4, MnFe2O4, NiFe2O4 and ZnFe2O4 are spherical with the uniform shape and distribution. Fig. 2 gives a detailed description of MFe2O4. MFe2O4 with the grain diameter of 100–350 nm. Among them, CoFe2O4 and CuFe2O4 had the smallest average particle sizes (127 nm for CoFe2O4 and 151 nm for CuFe2O4), which might be the cause for their relatively serious agglomeration. The particle sizes of various MFe2O4 showed the following decreasing sequence: MnFe2O4 > ZnFe2O4 > NiFe2O4 > CuFe2O4 > CoFe2O4.
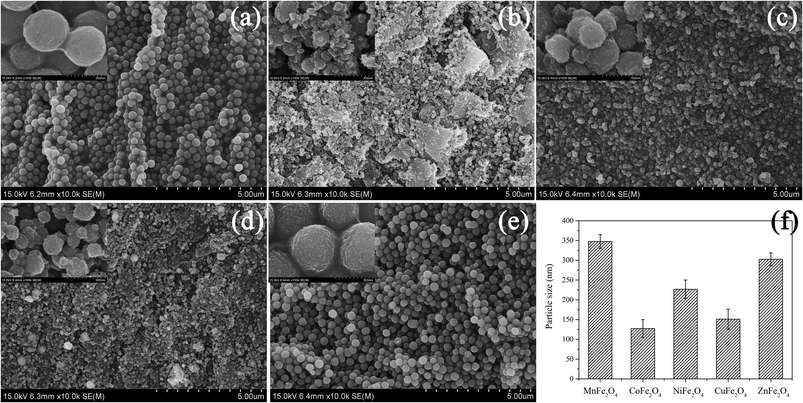 |
| Fig. 2 SEM images of MFe2O4: (a) MnFe2O4, (b) CoFe2O4, (c) NiFe2O4, (d) CuFe2O4 and (e) ZnFe2O4 (f) size distributions of MFe2O4 nanoparticles. | |
EDS analysis results (shown in Table 1) confirmed that the component contents of the samples are in reasonable agreement with the atomic percentages deduced from their molecular formulas. The elemental composition in Table 1 indicates that there are only three elements (O, M and Fe) in the composite. Furthermore, the M/Fe molar ratios of samples were approximately 0.5. The contents of the three elements in each complex MFe2O4 were 10.48 ± 0.99% (M, at%) and 22.18 ± 0.42% (Fe, at%), respectively.
Table 1 Physical and chemical properties of the investigated spinel catalysts
Catalysts |
SBET (m2 g−1) |
Elemental composition (atomic percentage, %) |
Crystallite size from XRD (nm) |
M cation (Mn/Zn/Cu/Ni/Co) |
Fe |
M/Fe |
MnFe2O4 |
22.243 |
10.83 |
22.26 |
0.487 |
15.94 |
CoFe2O4 |
121.352 |
11.94 |
22.72 |
0.458 |
11.61 |
NiFe2O4 |
72.727 |
10.21 |
21.63 |
0.472 |
10.07 |
CuFe2O4 |
30.009 |
9.24 |
21.92 |
0.422 |
12.78 |
ZnFe2O4 |
20.326 |
10.20 |
22.37 |
0.456 |
14.11 |
Table 1 shows the specific surface areas of the five spinel catalysts. The five ferrites exhibited different specific surface areas due to their different metal compositions. Among the five OCs, CoFe2O4 had the largest specific surface area (121.352 m2 g−1), followed by NiFe2O4 (72.727 m2 g−1). The ferrites of MnFe2O4, CuFe2O4 and ZnFe2O4 had the smallest specific surface areas (less than 30 m2 g−1). The smaller the diameter of MFe2O4 nanoparticles was, the larger the specific surface area was (Fig. 2f and Table 1).
XRD measurements were conducted to identify the crystal structures of the samples. The XRD patterns of MFe2O4 (M = Mn, Zn, Cu, Ni and Co) are shown in Fig. 3. X-ray powder diffraction patterns of MnFe2O4, CoFe2O4, NiFe2O4 and ZnFe2O4 samples were quite similar to those of cubic spinel-type structures belonging to the Fd
m (227) space group. The main diffraction reflection peaks included (220), (311), (400), (422), (511) and (440) crystal planes, which well matched with the standard XRD pattern representing the cubic spinel structure (JCPDS no. 38-0430,22-1086, 10-0325, 34-0425 and 10-1108), indicating the good crystallinity of these MFe2O4.33 Unlike these samples, however, CuFe2O4 exhibited a well-defined spinel ferrite structure with a strongest peak reflection peak at the plane (211) that matched well with the standard XRD pattern (JCPDS no. 34-0425) representing a tetragonal system (space group: I41/amd).34
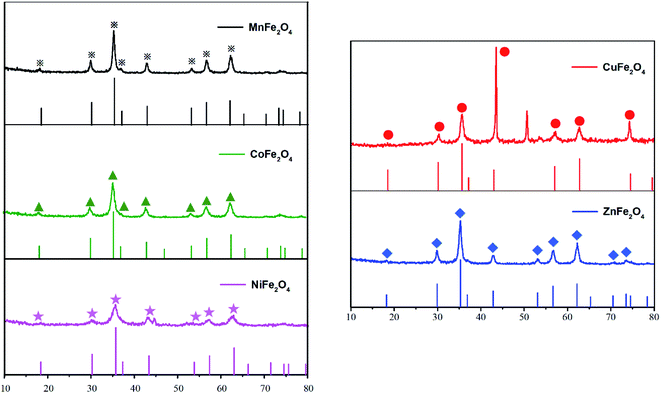 |
| Fig. 3 XRD patterns of the investigated spinel catalysts MFe2O4 and their standard profiles. | |
The crystallite size D for each sample was estimated according to the most intense reflection peak (311) with Scherrer's equation (eqn (7)):35
|
 | (7) |
where
D is the average crystallite size;
K is Scherrer constant, 0.89; corresponds to the wavelength of Cu-Kα radiation, 0.154 nm;
β is the full width at half maximum intensity for a reflection maximum;
θ is the diffraction angle.
With Scherrer's Equation, crystallite sizes of MFe2O4 nanocomposites were calculated to be 13 nm (Table 1). The crystallite sizes of these OCs ranged from 10.07 to 15.94 nm. The crystallite sizes of the five MFe2O4 showed the following decreasing sequence: MnFe2O4 > ZnFe2O4 > CuFe2O4 > CoFe2O4 > NiFe2O4. As the crystal grains of OCs increased, the crystallinity became better, but the probability of agglomeration also increased. CoFe2O4 and NiFe2O4 were most prone to agglomerate because of their smallest grain sizes, which were consistent with the SEM results (Fig. 2).
In order to give an insight into the elemental chemical valence and electronic properties on the surface layers of MFe2O4, XPS measurements of MFe2O4 before and after the reactions were conducted to examine the chemical compositions and electronic structures of the samples. Fig. 4 shows the XPS spectra of MFe2O4, respectively. In full-scale XPS spectra (Fig. 4a), the apparent peaks of Fe 2p, C 1s, O 1s, Mn 2p, Co 2p, Ni 2p, Cu 2p and Zn 2p illustrated that iron, manganese, cobalt, nickel, copper and zinc were the main elements of MFe2O4. The comparison of the peak differentiation-imitating analysis results of the nano-MFe2O4 before and after the reaction (Fig. 4b–e) indicated that the binding energy of M 2p3/2 was slightly reduced. After the reaction, the M(II) content in MFe2O4 decreased, whereas the M(III) content increased with a variation range of 8.5 ± 4.03%. Fe 2p3/2 was found to have characteristic peaks of Fe(II) and Fe(III) before the reaction, and the contents Fe(II) and Fe(III) were 72.46% and 27.53%, respectively. After the reaction, the contents of Fe(II) and Fe(III) were changed to 66.67% and 33.33% (Fig. 4f). The chemical states of Fe were changed in the catalytic process, so the catalyst always maintained a certain activity.36 These results demonstrated that Fe and M elements were chemically cyclically converted during the catalytic oxidation of NOx, while generating active sites for free radicals.
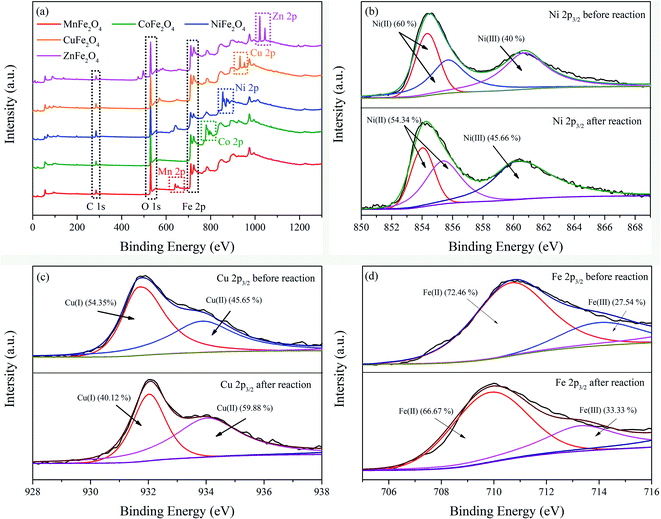 |
| Fig. 4 XPS patterns of MFe2O4 in the NOx–SO2–MFe2O4–MNB system before and after reactions: full-range scan of the samples (a), Ni 2p3/2 (b), Cu 2p3/2 (c), and Fe 2p3/2 of ZnFe2O4 (d). | |
3.2. Catalytic performance
The catalytic activities of the prepared samples of ZnFe2O4 nanocomposites against NOx and SO2 are shown in Fig. 5a. The ZnFe2O4 addition amount of 0.02 g promoted the removal of NOx by the NOx–SO2–MFe2O4–MNB system, whereas the addition amount of 0.05 g inhibited the removal of NOx. The addition of trace catalysts can promote the oxidation absorption of NOx. However, excessive catalysts need to consume reactive oxygen species in the process of transformation to high price state, which forms a competitive relationship with the oxidation of NOx and SO2, resulting in the reduction of NOx removal rate. In addition, with the increase of the amount of additives, the particle catalyst and micro nano bubbles collide with each other, leading to a part of bubbles in advance rupture, unable to produce hydroxyl radicals, resulting in a reduction of NOx removal rate. Therefore, 0.02 g of iron-based spinel samples were separately added to the NOx–SO2–MFe2O4–MNB system.
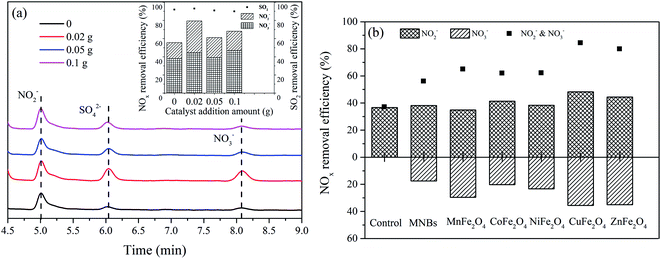 |
| Fig. 5 Effect of spinel catalyst on NOx and SO2 removal rate: ZnFe2O4 (a), MFe2O4 (b). | |
The NOx removal efficiency is shown in Fig. 5b. The NOx removal efficiency of the NOx–SO2–MFe2O4–MNB system was improved after the addition of MFe2O4. The combined efficiency of MNB and MFe2O4 in removing NOx reached 70.23 ± 10.63%, while the efficiency of SO2 removal reached 100% (eqn (17)–(21)). Oxidative absorption of NOx involves two steps. NOx is firstly oxidized into NO2−, which is then oxidized to NO3− (eqn (13)–(16)). MnFe2O4, CuFe2O4 and ZnFe2O4 significantly improved the efficiency of the second step. After adding CuFe2O4, the efficiency of converting NOx into NO2− increased by 10.16%, and the conversion into NO3− was 18.09%. Therefore, the comprehensive efficiency rate of NOx treatment by the NOx–SO2–CuFe2O4–MNB system reaches 83.88%. The catalytic activities showed the following decreasing sequence: CuFe2O4 > ZnFe2O4 > MnFe2O4 > CoFe2O4 > NiFe2O4.
3.3. Free radical identification
EPR test was conducted to detect the radicals and analyze the oxygen species of the NOx–SO2–MFe2O4–MNB system. As shown in Fig. 6a, weak signals were observed in MNB solution alone, and an intensive symmetrical four-line peak was detected in the NOx–SO2–MFe2O4–MNB system. The four-line peak with the intensity ratio of 1
:
2
:
2
:
1 in the spectrum is the typical spectral shape of the DMPO–˙OH adducts,37 which indicates the existence of ˙OH in the system. However, the characteristic 1
:
1
:
1 triplet assigned to DMPO–˙O2− adducts38 formed by ˙O2− and DMPO in Fig. 6b was very weak, indicating that the system mainly removes NOx through ˙OH instead of ˙O2−. The intensity of the peak represents the concentration of ˙OH in the system, indicating that the concentration of ˙OH decreases in turn: MNB/CuFe2O4 ≫ MNB > H2O. This result was consistent with the removal rate of NOx, which directly proved that ˙OH oxidized NOx is the key to denitrification of flue gas. It can also be proved that MFe2O4 can be activated by MNB to produce ˙OH, which is dominant in the process of NOx removal.
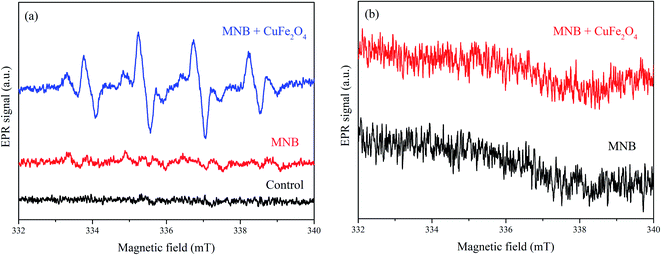 |
| Fig. 6 EPR spectra of the NOx–SO2–MFe2O4–MNB system: DMPO–˙OH (a), and DMPO–˙O2− (b). | |
3.4. Proposed reaction pathways
It can be found that the NOx–SO2–MFe2O4–MNB system has a high catalytic efficiency as a heterogeneous catalytic system, based on characterizing the physical and chemical properties of MFe2O4 (M = Mn, Zn, Cu, Ni and Co) before and after the reaction and analyzing the SO2 and NOx removal efficiency. Hydroxyl radical is the main oxidant during the chemical oxidation of SO2 and NOx in aqueous solution. In this system, the sources of hydroxyl radicals are divided into two parts. Some hydroxyl radicals are derived from the rupture of MNB,39,40 and the other part is derived from the activation of recyclable MFe2O4. The chemistry of simultaneous oxidation of NOx and SO2 by micro–nano bubbles breakdown and MFe2O4 activation is complex, since it takes place through electron transfer from the lattice oxygen, free radical oxidation reactions via ˙OH and ˙O2−, and the direct reaction of the NOx with HSO3−.41,42
The catalytic oxidation mechanism of NOx–SO2–MFe2O4–MNB system is described visually in the reaction scheme shown in Fig. 7. As the MNB slowly rise and shrink in the water, the charge ions (H+, OH−) rapidly concentrate at the very narrow gas–liquid interface. At the moment of MNB explosion, due to the drastic changes caused by the disappearance of the gas–liquid interface, the concentrated ions on the interface will immediately release the stored chemical energy, thus producing a large number of ˙OH. MFe2O4 is a magnetic semiconductor material with spinel structure. Oxygen in molecular state (O2) is adsorbed on the metal surface and then dissociated into adsorbed atoms (O ad) because MFe2O4 is magnetic.43 The type I adsorbed oxygen atom (O ad(I)) is first formed during dissociation, which is equivalent to a locally adsorbed oxygen atom on the metal surface. The oxygen atom in this state has extremely high reactivity to NOx. In addition, as a semiconductor material, MFe2O4 consists of a low energy valence band full of electrons and an empty high energy conduction band. In the excited state, the valence band electron jumps to the valence band, which is called the conduction band electron (eCB−). At the same time, a highly active hole in the valence band is generated, which is called the valence band electron hole (hVB+). The electron hole pair produces a large number of reactive oxygen species such as hydroxyl free radicals and hydrogen peroxide free radicals on the material surface, as shown in eqn (8)–(12). Reactive oxygen species is the main oxidant in the process of removing NOx and SO2. According to the double reduction oxidation mechanism, a kind of cations (Fe3+) perform the function of activating and oxidizing NOx and SO2, and their reoxidation depends on the lattice oxygen (O2−) transferred along the lattice. Another metal cation (M2+ (M = Mn, Co, Ni, Cu and Zn)) in the reduced state assumes the role of accepting gas-phase oxygen.
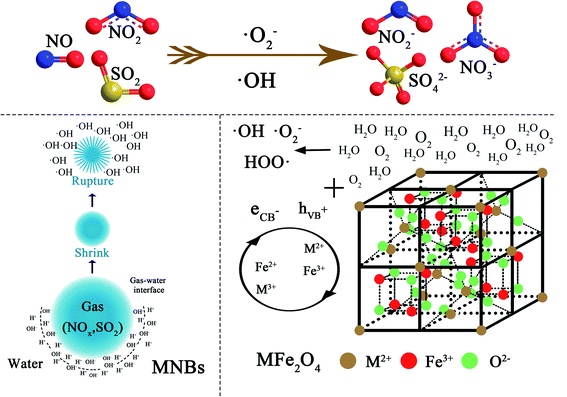 |
| Fig. 7 Schematic diagram of the NOx–SO2–MFe2O4–MNB reacting system. | |
The main reactions involved in spinel catalytic oxidation are:
|
˙O2− + H2O → HOO˙ + OH−
| (10) |
|
2HOO˙ + eCB−→ ˙OH + O2 + OH−
| (12) |
The main reactions involved in the simultaneous oxidation of NO
x and SO
2 are:
|
2NO2 + H2O → 2H+ + NO3− + NO2−
| (14) |
|
NO + ˙OH → H+ + NO2−
| (15) |
|
NO2− + ˙OH → NO2 + OH−
| (16) |
|
NO2 + ˙OH → H+ + NO3−
| (17) |
|
SO2 + H2O ↔ HSO3− + H+
| (19) |
|
HSO3− + ˙OH → H2O + SO3˙−
| (21) |
|
SO32− + ˙OH → SO3˙− + OH−
| (22) |
|
SO3˙− + 2˙OH → H2O + SO42−
| (23) |
|
2NO2 + SO32− + H2O → 2NO2− + SO42− + 2H+
| (24) |
|
2NO2 + 2SO32− + H2O → NO2− + NO3− + 2HSO3−
| (25) |
4. Conclusion
This work developed a new technology that uses micro–nano bubbles and MFe2O4 to simultaneously remove NOx and SO2 from flue gas. The physicochemical properties of MFe2O4 prepared by the hydrothermal method were characterized. The results indicated that both micro–nano bubbles and MFe2O4 improved NOx removal, while and the removal rate of SO2 can reached 100% at room temperature. NOx removal rate reached 83.88% in NOx–SO2–CuFe2O4–MNB system. At the moment when the MNB burst, the concentrated ions on the gas–water interface will release the stored chemical energy at once, thus producing a large number of hydroxyl radicals. Recyclable MFe2O4 (M = Mn, Zn, Cu, Ni and Co) can produce ˙OH and ˙O2− by being activated, thus accelerating the oxidation and absorption process of NOx and SO2. However, this research also points out many areas that require further research, such as using the system for organic gas and wastewater treatment, which is beyond the scope of current research, but is still the subject of future research.
Conflicts of interest
There are no conflicts to declare.
Acknowledgements
This work was supported by the National Natural Science Foundation of China [grant number U1660107], the Fundamental Research Funds for the Central Universities [grant number 2232020A-10].
References
- F. X. Qie, J. Y. Zhu, J. F. Rong and B. N. Zong, Biological removal of nitrogen oxides by microalgae, a promising strategy from nitrogen oxides to protein production, Bioresour. Technol., 2019, 292, 10 CrossRef PubMed.
- D. L. Goldberg, Z. F. Lu, D. G. Streets, B. de Foy, D. Griffin, C. A. McLinden, L. N. Lamsal, N. A. Krotkov and H. Eskes, Enhanced capabilities of TROPOMI NO2: estimating NOx from north American cities and power plants, Environ. Sci. Technol., 2019, 53, 12594–12601 CrossRef CAS PubMed.
- K. H. Bates and D. J. Jacob, A new model mechanism for atmospheric oxidation of isoprene: global effects on oxidants, nitrogen oxides, organic products, and secondary organic aerosol, Atmos. Chem. Phys., 2019, 19, 9613–9640 CrossRef CAS.
- J. Q. Xu, H. J. Yu, C. Zhang, F. Guo and J. Q. Xie, Development of cerium-based catalysts for selective catalytic reduction of nitrogen oxides: a review, New J. Chem., 2019, 43, 3996–4007 RSC.
- Z. Y. Wang, H. L. Kuang, J. F. Zhang, L. L. Chu and Y. L. Ji, Nitrogen oxide removal by non-thermal plasma for marine diesel engines, RSC Adv., 2019, 9, 5402–5416 RSC.
- M. Jablonska and R. Palkovits, Perovskite-based catalysts for the control of nitrogen oxide emissions from diesel engines, Catal. Sci. Technol., 2019, 9, 2057–2077 RSC.
- J. M. Shao, C. Q. Xu, Z. H. Wang, J. P. Zhang, R. T. Wang, Y. He and K. F. Cen, NOx reduction in a 130 t/h biomass-fired circulating fluid bed boiler using coupled ozonation and wet absorption technology, Ind. Eng. Chem. Res., 2019, 58, 18134–18140 CrossRef CAS.
- J. Chen, Y. Chen, D. Hrynsphan, Y. Mei, H. Pan, J. L. Wu, J. M. Chen and J. C. Yao, Fe-II(EDTA)-NO reduction by Mn powder in wet flue gas denitrification technology coupled with Mn2+ recycling: performance, kinetics, and mechanism, Energy Fuels, 2020, 34, 2590–2598 CrossRef CAS.
- X. Y. Zhu, F. Q. He, M. Xia, H. G. Liu and J. H. Ding, Evaluation of Fe(III)EDTA reduction with ascorbic acid in a wet denitrification system, RSC Adv., 2019, 9, 24386–24393 RSC.
- J. Johansson, F. Normann, N. Sarajlic and K. Andersson, Technical-scale evaluation of scrubber-based, Co-removal of NOx and SOx species from flue gases via gas-phase oxidation, Ind. Eng. Chem. Res., 2019, 58, 21904–21912 CrossRef CAS.
- Z. G. Xiao, D. X. Li, F. K. Wang, Z. H. Sun and Z. Y. Lin, Simultaneous removal of NO and SO2 with a new recycling micro-nano bubble oxidation-absorption process based on HA-Na, Sep. Purif. Technol., 2020, 242, 12 CrossRef.
- Z. G. Xiao, D. X. Li, Q. L. Zhu and Z. H. Sun, Simultaneous removal of NO and SO2 through a new wet recycling oxidation-reduction process utilizing micro-nano bubble gas-liquid dispersion system based on Na2SO3, Fuel, 2020, 263, 10 CrossRef.
- W. Fan, Z. Zhou, W. T. Wang, M. X. Huo, L. L. Zhang, S. Y. Zhu, W. Yang and X. Z. Wang, Environmentally friendly approach for advanced treatment of municipal secondary effluent by integration of micro-nano bubbles and photocatalysis, J. Clean. Prod., 2019, 237, 8 CrossRef.
- S. Hamamoto, T. Takemura, K. Suzuki and T. Nishimura, Effects of pH on nano-bubble stability and transport in saturated porous media, J. Contam. Hydrol., 2018, 208, 61–67 CrossRef CAS.
- Y. X. Liu, Y. P. Zhou, T. Z. Wang, J. C. Pan, B. Zhou, T. Muhammad, C. F. Zhou and Y. K. Li, Micro-nano bubble water oxygation: Synergistically improving irrigation water use efficiency, crop yield and quality, J. Clean. Prod., 2019, 222, 835–843 CrossRef CAS.
- Y. F. Wu, H. Lin, W. Z. Yin, S. C. Shao, S. H. Lv and Y. Y. Hu, Water Quality and Microbial Community Changes in an Urban River after Micro-Nano Bubble Technology in Situ Treatment, Water, 2019, 11, 14 Search PubMed.
- W. P. Zhang, G. Y. Li, H. L. Liu, J. Y. Chen, S. T. Ma and T. C. An, Micro/nano-bubble assisted synthesis of Au/TiO2@CNTs composite photocatalyst for photocatalytic degradation of gaseous styrene and its enhanced catalytic mechanism, Environ. Sci.: Nano, 2019, 6, 948–958 RSC.
- Z. R. Xia and L. M. Hu, Treatment of Organics Contaminated Wastewater by Ozone Micro-Nano-Bubbles, Water, 2019, 11, 10 Search PubMed.
- Z. G. Xiao, T. Bin Aftab, X. L. Yuan, H. L. Xia and D. X. Li, Experimental results of NO removal by the MBGLS, Micro & Nano Lett., 2019, 14, 721–726 CrossRef CAS.
- O. S. Furman, A. L. Teel and R. J. Watts, Mechanism of base activation of persulfate, Environ. Sci. Technol., 2010, 44, 6423–6428 CrossRef CAS PubMed.
- Y. G. Adewuyi, N. Y. Sakyi and M. A. Khan, Simultaneous removal of NO and SO2 from flue gas by combined heat and Fe2+ activated aqueous persulfate solutions, Chemosphere, 2018, 193, 1216–1225 CrossRef CAS PubMed.
- K. Q. Wang, B. L. Dou, B. Jiang, Q. Zhang, M. Li, H. S. Chen and Y. J. Xu, Effect of support on hydrogen production from chemical looping steam reforming of ethanol over Ni-based oxygen carriers, Int. J. Hydrogen Energy, 2016, 41, 17334–17347 CrossRef CAS.
- S. Golchinvafa, S. M. Masoudpanah and M. Jazirehpour, Magnetic and microwave absorption properties of FeCo/CoFe2O4 composite powders, J Alloy Compd, 2019, 809, 7 CrossRef.
- Y. Wang, X. Gao, X. M. Wu, W. Z. Zhang, C. Y. Luo and P. B. Liu, Facile design of 3D hierarchical NiFe2O4/N-GN/ZnO composite as a high performance electromagnetic wave absorber, Chem. Eng. J., 2019, 375, 10 CrossRef.
- M. Taei, E. Havakeshian, H. Salavati and M. Azemati, Highly active electrocatalysts for ethanol oxidation based on gold nanodendrites modified with NiFe2O4 nanoparticles decorated multi-walled carbon nanotubes, Chem. Pap., 2019, 73, 2687–2695 CrossRef CAS.
- J. G. Kim, Y. Noh, Y. Kim, S. Lee and W. B. Kim, Formation of ordered macroporous ZnFe2O4 anode materials for highly reversible lithium storage, Chem. Eng. J., 2019, 372, 363–372 CrossRef CAS.
- E. Finley, M. W. Gaultois and J. Brgoch, Unlocking the key to persistent luminescence with X-ray absorption spectroscopy: a local structure investigation of Cr-substituted spinel-type phosphors, Phys. Chem. Chem. Phys., 2019, 21, 19349–19358 RSC.
- X. C. Huang, J. Y. Zhang, M. Wu, S. Zhang, H. Y. Xiao, W. Q. Han, T. L. Lee, A. Tadich, D. C. Qi, L. Qiao, L. Chen and K. H. L. Zhang, Electronic structure and p-type conduction mechanism of spinel cobaltite oxide thin films, Phys. Rev. B, 2019, 100, 9 Search PubMed.
- Y. Liu, D. P. Xu, T. Cui, H. M. Yu, X. F. Li and L. Li, Growth and properties of spinel structure Zn1.8Co0.2TiO4 single crystals by the optical floating zone method, RSC Adv., 2019, 9, 26436–26441 RSC.
- Y. F. Li, J. H. Shen, Y. J. Hu, S. J. Qiu, G. Q. Min, Z. T. Song, Z. Sun and C. Z. Li, General flame approach to chainlike MFe2O4 spinel (M = Cu, Ni, Co, Zn) nanoaggregates for reduction of nitroaromatic compounds, Ind. Eng. Chem. Res., 2015, 54, 9750–9757 CrossRef CAS.
- T. Wang, J. Y. Wang, Y. M. Sun, Y. Duan, S. N. Sun, X. Hu, S. B. Xi, Y. H. Du, C. Wang and Z. C. J. Xu, Origin of electronic structure dependent activity of spinel ZnNixCo2-xO4 oxides for complete methane oxidation, Appl. Catal. B-Environ., 2019, 256, 9 Search PubMed.
- C. C. Xu, W. Sun, L. M. Cao and J. Yang, Highly efficient Pd-doped ferrite spinel catalysts for the selective catalytic reduction of NO with H-2 at low temperature, Chem. Eng. J., 2016, 289, 231–238 CrossRef CAS.
- J. Li, J. F. Yan, G. Yao, Y. H. Zhang, X. Li and B. Lai, Improving the degradation of atrazine in the three-dimensional (3D) electrochemical process using CuFe2O4 as both particle electrode and catalyst for persulfate activation, Chem. Eng. J., 2019, 361, 1317–1332 CrossRef CAS.
- R. Z. Zhang, J. M. Liu, S. F. Wang, J. Z. Niu, C. G. Xia and W. Sun, Magnetic CuFe2O4 Nanoparticles as an Efficient Catalyst for C-O Cross-Coupling of Phenols with Aryl Halides, ChemCatChem, 2011, 3, 146–149 CrossRef CAS.
- J. H. Sui, C. Zhang, D. Hong, J. Li, Q. Cheng, Z. G. Li and W. Cai, Facile synthesis of MWCNT-ZnFe2O4 nanocomposites as anode materials for lithium ion batteries, J. Mater. Chem., 2012, 22, 13674–13681 RSC.
- Y. B. Ding, L. H. Zhu, N. Wang and H. Q. Tang, Sulfate radicals induced degradation of tetrabromobisphenol A with nanoscaled magnetic CuFe2O4 as a heterogeneous catalyst of peroxymonosulfate, Appl. Catal. B-Environ., 2013, 129, 153–162 CrossRef CAS.
- B. C. Yang, S. X. Ma, R. J. Cui, S. J. Sun, J. Wang and S. C. Li, Simultaneous removal of NOx and SO2 with H2O2 catalyzed by alkali/magnetism-modified fly ash: High efficiency, low cost and catalytic mechanism, Chem. Eng. J., 2019, 359, 233–243 CrossRef CAS.
- Z. H. Meng, C. Y. Wang, X. R. Wang and H. Q. Li, Efficient and stable catalyst of alpha-FeOOH for NO oxidation from coke oven flue gas by the catalytic decomposition of gaseous H2O2, RSC Adv., 2020, 10, 8207–8211 RSC.
- M. Takahashi, ζ potential of microbubbles in aqueous solutions: Electrical properties of the gas-water interface, J. Phys. Chem. B, 2005, 109, 21858–21864 CrossRef CAS PubMed.
- M. Takahashi, K. Chiba and P. Li, Formation of hydroxyl radicals by collapsing ozone microbubbles under strongly acidic conditions, J. Phys. Chem. B, 2007, 111, 11443–11446 CrossRef CAS.
- N. Chaibakhsh and Z. Moradi-Shoeili, Enzyme mimetic activities of spinel substituted nanoferrites (MFe2O4): A review of synthesis, mechanism and potential applications, Mater. Sci. Eng., C, 2019, 99, 1424–1447 CrossRef CAS.
- S. Chandrasekaran, C. Bowen, P. X. Zhang, Z. L. Li, Q. H. Yuan, X. Z. Ren and L. B. Deng, Spinel photocatalysts for environmental remediation, hydrogen generation, CO2 reduction and photoelectrochemical water splitting, J. Mater. Chem. A, 2018, 6, 11078–11104 RSC.
- W. K. Zhao, S. L. Zhang, J. Ding, Z. Y. Deng, L. Guo and Q. Zhong, Enhanced catalytic ozonation for NOx removal with CuFe2O4 nanoparticles and mechanism analysis, J. Mol. Catal. A: Chem., 2016, 424, 153–161 CrossRef CAS.
|
This journal is © The Royal Society of Chemistry 2020 |
Click here to see how this site uses Cookies. View our privacy policy here.