DOI:
10.1039/D0RA05230B
(Paper)
RSC Adv., 2020,
10, 27538-27551
2D black phosphorus and tungsten trioxide heterojunction for enhancing photocatalytic performance in visible light
Received
14th June 2020
, Accepted 13th July 2020
First published on 23rd July 2020
Abstract
A novel, efficient and stable 2D black phosphorus and tungsten trioxide heterojunction (WO3–BPNs) was successfully synthesized using a combined hydrothermal, liquid phase exfoliating and co-precipitation method. The as-obtained WO3–BPNs composite was characterized by using XRD, SEM, XPS, UV-vis, etc. The results showed that the bandgap energy of the WO3–BPNs50 sample was 2.2 eV, which was lower than that of pure WO3. BPNs in the WO3–BPNs heterojunction as a co-catalyst effectively enhanced photo-generated electron–hole pairs separation. The synthesized WO3–BPNs sample significantly improved the photocatalytic performance in degrading rhodamine B (RhB) and metoprolol (MET) compared to pure WO3 and BPNs under visible-light. The maximum RhB and MET removal efficiencies were 92% and 87%, respectively, in the WO3–BPNs50 (added 50 mL BPNs dispersion) sample within 120 minutes. The relevant photocatalysis mechanisms were discussed. In addition, the intermediate products in the MET photodegradation process were investigated by LC-MS technology, and the degradation pathway of MET was proposed.
1 Introduction
Recently, a large number of toxic and hazardous organic pollutants have been released into aquatic environments, creating more and more environmental concerns.1,2 The photodegradation of organic contaminants under solar radiation using semiconductor materials has been considered as a promising method for treating such pollutants.3 Many semiconductor materials such as TiO2,4 WO3,5 ZnO,6 and CdS7 have been extensively investigated as photocatalysts for degrading organic pollutants under solar radiation. However, the low utilization rate of visible-light and the rapid recombination of photoelectron–hole pairs under photodegradation still inhibit the practical applications of semiconductor photocatalysts in the treatment of organic pollutants.1,2
Many investigations have been conducted to overcome these disadvantages and improve the photocatalytic performance, mainly including ion doping and the formation of heterojunction structures between two semiconductor materials.5,8 Previous studies demonstrated that photocatalytic performance of semiconductor materials was significantly enhanced due to the doping of non-metal elements N,9 S,5 and P,10 or metal elements Cu,11 Ag,12 and Ce.13 Liang et al. proposed that Ag/TiO2 and Ce/TiO2 have high photocatalytic degradation performance for formaldehyde gas under UV-light.13 The photocatalytic performance of catalyst can also be effectively improved by preparing a heterojunction structure between two semiconductor materials.8 The formation of a heterojunction structure between two different semiconductors could broaden the spectral response range and reduce the recombination of photogenerated carriers.14 Gao et al. found that the photodegradation efficiency promoted for the WO3/TiO2 heterojunction photocatalyst compared to WO3 and TiO2 under visible-light.8 More importantly, two-dimensional (2D) semiconductor materials attract extensive attentions benefiting from high charge-carrier mobility and adjustable bandgap energy.14 Ali et al. reported the graphene–TiO2 nanocomposites to enhance the photodegradation of rhodamine B dye.15 Kanda et al. prepared MoS2–TiO2 nanocomposite using a photo deposition-based technique for improving photocatalytic performance.16 However, the zero band gap of graphene17 and the low carrier mobility of MoS2 (200 cm2 V−1 s−1)18 limited their further development in the field of optoelectronics.
Black phosphorus (BP) has been recently attracted considerable attention due to its unique in-plane anisotropic structure, high carrier mobility, and adjustable direct bandgap.19,20 The adjustable direct bandgap of BP mainly depends on the exfoliation of BP layers, for instance the bandgap energy of bulk and single-layer BP is 0.3 eV and 1.5 eV, respectively.21,22 Thus, it is possible to expand the spectrum response of BP to visible, mid-infrared and near-infrared light by controlling the thickness of BP. Lee et al. reported BP@TiO2 photocatalysts had excellent photocatalytic performance under sunlight and little effect on temperature and pH conditions.23 Hu et al. prepared a heterojunction structure of 2D/2D BP/monolayer Bi2WO6 to improve the photocatalytic performance to produce H2.24 A BP–Au/LTO nanostructure prepared by Majima et al. was proved to be a high-efficiency photocatalyst for generating hydrogen under visible-light.25 In fact, despite BP has excellent optoelectronic properties, its photocatalytic performance remains largely unexplored in the application of degrading organic pollutants in aquatic environments under both UV and visible-lights.
In this study, a novel heterojunction of two-dimensional black phosphorus and WO3 (WO3–BPNs) was synthesized using a combined method of hydrothermal, liquid phase exfoliating and co-precipitation for the first time. The as-obtained WO3–BPNs composite was characterized by using X-ray diffraction (XRD), scanning electron microscopy (SEM), atomic force microscopy (AFM), X-ray photoelectron spectroscopy (XPS), specific surface aperture analysis (BET) and diffuse reflectance spectroscopy (UV-vis). The photocatalytic performance of the prepared WO3–BPNs was evaluated with rhodamine B (RhB) and metoprolol (MET) degradation experiments under visible-light. The photocatalysis mechanisms were also revealed and discussed. Finally, MET was selected to study the intermediate products in the photodegradation process with LC-MS technique, and a scheme of MET degradation pathway had been proposed.
2 Materials and methods
2.1 Chemicals
The BP crystals were supplied by Kunming Black Phosphorus Technology Co., Ltd. Rhodamine B (RhB) and N-methyl-2-pyrrolidone (NMP) (≥99.0%) were supplied by Shanghai Aladdin Biochemical Technology Co., Ltd. Metoprolol tartrate salt (≥98.0%) supplied by Shanghai Macklin Biochemical Co., Ltd. Na2WO4·2H2O and NaOH were supplied by Tianjin Yongda Chemical Reagent Co., Ltd.
2.2 Sample preparation
2.2.1 Preparation of the monoclinic WO3 nanocrystals and BP nanosheets. Monoclinic WO3 was synthesized with a hydrothermal method. First, 1.1547 g Na2WO4·2H2O was dispersed in 60 mL deionized water, and 35 mL HCl was mixed into the above-mixed solution. Then, the mixture had been placed in a stainless steel autoclave lined with Teflon and kept at 180 °C for 12 h. Finally, the hydrothermal product was repeatedly washed with deionized water and dried for further use.The BP nanosheets (BPNs) were obtained by solvent exfoliation process. Finally, 30 mg bulk BP was ground into the powder with an agate mortar. The BP powder was dispersed into 80 mL NMP solution, then added 10 mg NaOH. The dispersion was sonicated in an ice bath for 6 hours, and the output power of the sonicator was 300 W. The non-exfoliated bulk BP was removed from the obtained dispersion solution by centrifugation at 2000 rpm for 20 minutes. Then the supernatant after centrifugation was sonicated in an ice bath for 2 hours and the BP quantum dot had been removed though centrifugation at 7000 rpm for 20 minutes to obtain the BPNs. The product was resuspended in 30 mL NMP solution for further use. The concentration of BPNs dispersion was 0.2 mg mL−1.
2.2.2 Preparation of the WO3–BPNs sample. The WO3–BPNs sample was synthesized by co-precipitation in aqueous solution. In detail, 231.84 mg monoclinic WO3 was added to the 30 mL, 50 mL, and 100 mL BPNs dispersion (0.2 mg mL−1), which were marked as WO3–BPNs30, WO3–BPNs50, WO3–BPNs100 respectively. WO3 and BPNs dispersion were stirred for 2 hours and ultrasonicated for another 2 hours. The product was collected from the dispersion by high-speed centrifugation (7000 rpm). The product was sufficiently washed with deionized water to neutrality. Finally, the WO3–BPNs sample was obtained by freeze-dried.
2.3 Characterization
The analysis of the crystal structure of the obtained sample was performed by XRD at 20 °C using an X-ray diffractometer (D8-Advance), and the diffraction angle ranged from 10° to 60°. The morphology of the obtained sample was characterized by using an SEM (sigma 300 SEM, Japan), with scanning voltage of 8 and 5 kV for BP and other samples. The binding energy was analyzed by an XPS (Thermo ESCALAB 250XI, Japan). The thickness of BP nanosheet was analyzed with AFM images (Bruker Dimension Edge). The UV-vis diffuse reflectance spectra were tested using a UV-2450 with an integrating sphere (ISR-240, Japan).
2.4 Photocatalytic performance experiments
The photocatalytic efficiency of WO3–BPNs samples were tested by photodegrading RhB and MET with visible-light. The 350 W Xe-lamp (1900 mW cm−2) was used in the photocatalysis experiment as the light source. The Xe-lamp was equipped with a 420 nm cut-off filter to eliminate the effect of ultraviolet light. The sample (50 mg WO3–BPNs) was added into a quartz tube with 50 mL RhB (10 mg L−1) or MET (10 mg L−1). The solution was placed without light for 30 minutes to achieve the equilibrium of adsorption and desorption. The 5 mL mixed solution was taken every 30 minutes for testing, and the suspended photocatalyst was removed though centrifugation. The concentrations of RhB and MET solution were evaluated utilizing a UV-vis spectrophotometer (UV-2450) and a high-efficiency liquid chromatography (Shimadzu, LC-10AT, Japan), respectively.
2.5 Analysis of metoprolol and its degradation products
MET and its oxidation by-products were identified by using the Ultimate 3000 UHPLC-Q Exactive LC/MS (Thermo Scientific, US) with Eclipse Plus C18 column, 250 × 4.6 mm during the photoreaction. The mobile phase used in the test was a 0.1% mixture of formic acid and acetonitrile with the volume ratio of 20
:
80. The device measurement mode was positive ion mode, using ESI ion source, the range: 50–750 m/z; the flow rate: 0.4 mL min−1; DL tube temperature: 300 °C; gas lift rate: 40 mL min−1; auxiliary gas rate: 10 mL min−1; spray voltage: positive ion 4.0 kV; and fragmentation energy: NCE 30.
3 Results and discussions
3.1 Characterization of the WO3–BPNs sample
3.1.1 Structural and morphological analysis. The XRD patterns of bulk BP, WO3, and WO3–BPN were depicted in Fig. 1. It could be detected clearly that the characteristic diffraction peaks of the obtained WO3 and bulk BP match well with the monoclinic WO3 crystal structure (JCPDS card no. 043-1035) and BP crystal structure (JCPDS card no. 76-1957). The diffraction peaks at 17.3°, 34.6° and 52.7° were assigned to the (020), (040) and (060) planes of BP, respectively.23 The diffraction peaks at 23.1°, 23.6°, 24.4°, and 26.6° were assigned to the (002), (020), (200) and (120) planes of the monoclinic WO3, respectively.26 All the diffraction peaks of WO3–BPNs composite can be ascribed to WO3 and bulk BP, without any other peaks. In addition, the diffraction peaks of the composite samples were very similar to those of pure WO3, indicating that the intervention of BPNs had little effect on the lattice parameters of WO3. The intensity of the diffraction peak of the (020) crystal plane also increased with the increase of the amount of BPNs doping.
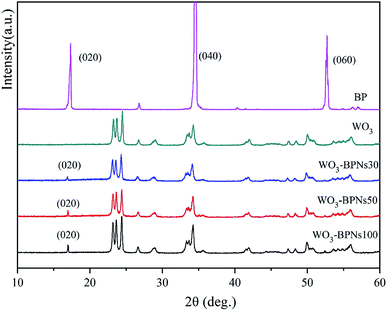 |
| Fig. 1 XRD patterns of bulk BP, WO3 and WO3–BPNs composites. | |
Fig. 2a–c show SEM images of WO3, BP and WO3–BPNs, respectively. In Fig. 2a, WO3 nanoparticles are 50–100 nm in length and width. The SEM image of the exfoliated BP sample exhibited the two-dimensional layered structure in Fig. 2b. Fig. 2c shows the morphology of WO3–BPNs composites sample, where WO3 particles were attached to the surface of BP. The SEM image confirmed the formation of WO3–BPNs composite material between WO3 and BPNs. Fig. 3a shows the AFM image of the prepared BPNs. The one-dimensional cross-sectional scan of the prepared BPNs surface thickness was shown in Fig. 3b. It could be found that the thickness of the obtained BPN was 3.2 nm. The obtained BPNs was approximately six layers of thickness due to a BP single layer of 0.53 nm.27
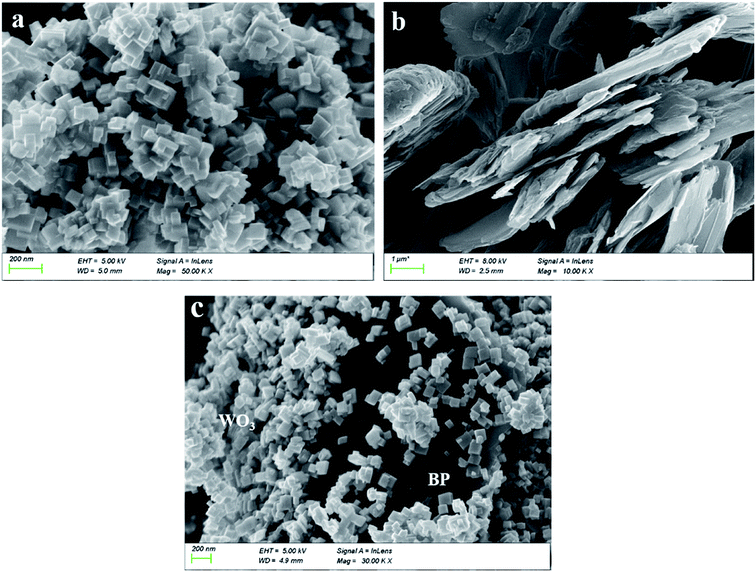 |
| Fig. 2 SEM images of WO3 (a), BPNs (b) and WO3–BPNs50 composites (c). | |
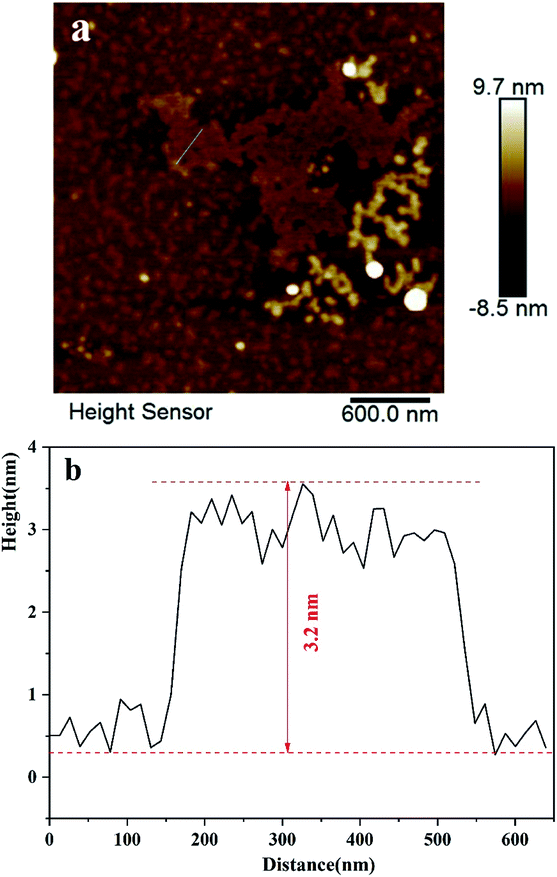 |
| Fig. 3 AFM images (a) and 1D cross-sectional scan of surface profile thickness of the BPNs sample (b). | |
3.1.2 XPS and UV-vis analysis. The element composition and valence of the WO3–BPNs samples were analyzed with XPS. The XPS spectra for W 4f, O 1s and P 2p of the all samples are listed in Fig. 4. There were two strong characteristic peaks ascribed to the binding energies for W 4f7/2 and W 4f5/2 in Fig. 4a. The binding energy of W 4f7/2 and W 4f5/2 were measured at 35.6 eV and 37.7 eV, respectively, which were following the previous reports.5 The two peaks of both W 4f7/2 and W 4f5/2 for WO3–BPNs were shifted by 0.2 eV towards lower energy compared with those of WO3, as presented in Fig. 4a(1) and (2). The O 1s XPS spectra of WO3 and WO3–BPNs are shown in Fig. 4b. The peaks at 530.4 eV and 530.2 eV corresponded to the O2− ion in the W–O bond of WO3 and WO3–BPNs, respectively. It can be found that the peak of O 1s for WO3–BPNs shifted to the low energy of 530.2 eV, which was related to the adsorbed oxygen at the sample surface.9,23 Fig. 4c presents, three peaks of BPNs detected at 129.2 eV, 130.2 eV and 134.2 eV, which were attributed to the P 2p3/2, P 2p1/2, and oxidized phosphorus (PxOy), respectively.28 The two characteristic peaks of P 2p1/2 and P 2p2/3 of WO3–BPNs shifted to the higher binding energy of 130.3 eV and 129.6 eV, respectively, while the binding energy of PxOy decreased to 132.9 eV.
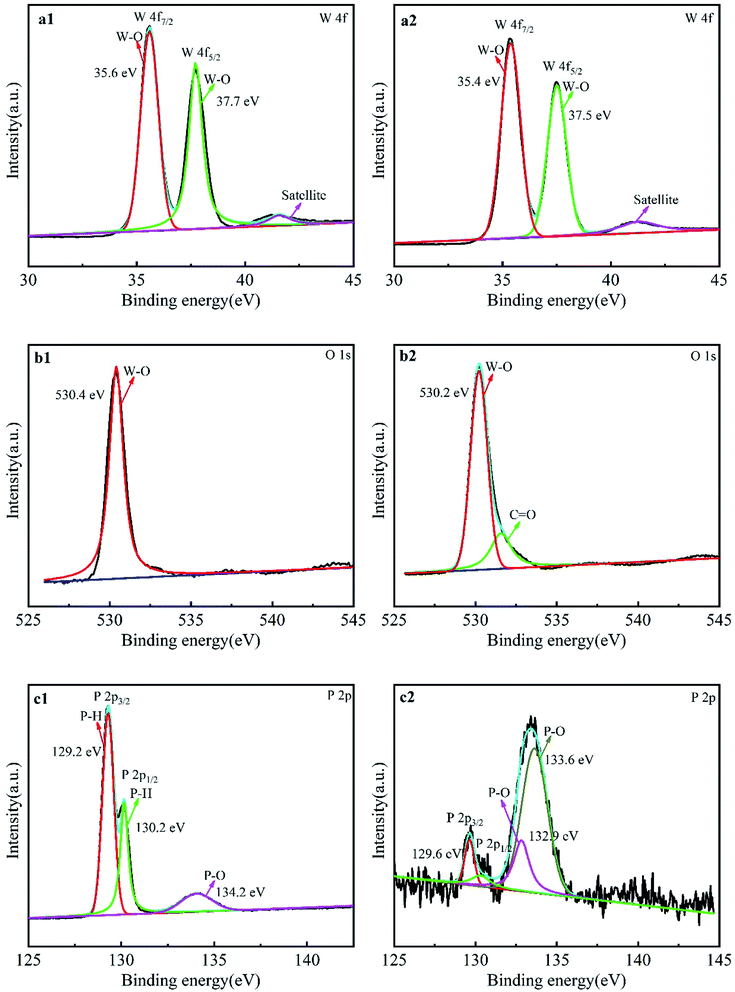 |
| Fig. 4 XPS spectra for W 4f of WO3 (a1) and WO3–BPNs50 (a2), O 1s of WO3 (b1) and WO3–BPNs (b2), and P 2p of BP (c1) and WO3–BPNs (c2). | |
The change in binding energy in the composite means that there was a close interaction between WO3 and BPNs.23 In the composite material, the binding energy of O 1s decreased and the binding energy of P 2p increased, suggesting the interaction between WO3 and BPNs in the WO3–BPNs composites.28 The WO3–BPNs composite showed a P 2p binding energy at 133.6 eV, suggesting that phosphorus in the composites existed in the form of a pentavalent oxidation state, and was obviously in the form of a P–O bond.23 In addition, XPS analysis showed that phosphorus ions on the surface of WO3–BPNs were in the states of P5+ and P3+, which provided direct evidence for the electronic transfer between WO3 and BPNs.
The absorption edge was affected by the bandgap width of the photocatalyst, which determined the light absorption region of the sample.29 Fig. 5a exhibits the UV-vis absorption spectra of WO3, BPNs and WO3–BPNs samples. The absorption value of BPNs in different wavelength ranges did not change significantly, and the absorption value of BPNs was much higher than that of WO3 in the visible range. The presence of BPNs enhanced the light absorption significantly for the WO3–BPNs sample in the region of wavelength λ > 400 nm. Such an effect generally increased with the increase of the amount of BPNs. The reason was that BP could absorb a wide range of radiation from visible to infrared light because the bandgap energy of BP depended on the thickness.21 Fig. 5b shows the calculation of bandgap energy of WO3 and the WO3–BPNs by Tauc equation.8 The results show that the calculated bandgap energy of the WO3–BPNs50 and WO3 was 2.2 eV and 2.5 eV, respectively. However, BPNs belonged to black crystals and the calculated bandgap energy could not obtained be by diffuse reflection spectroscopy. The bandgap energy of BPNs depended on its thickness. AFM results showed that the thickness of BPNs was about 6 layers of nanosheets. According to the theoretical calculation method reported previously,30 the bandgap energy of the BPNs was about 0.5 eV.
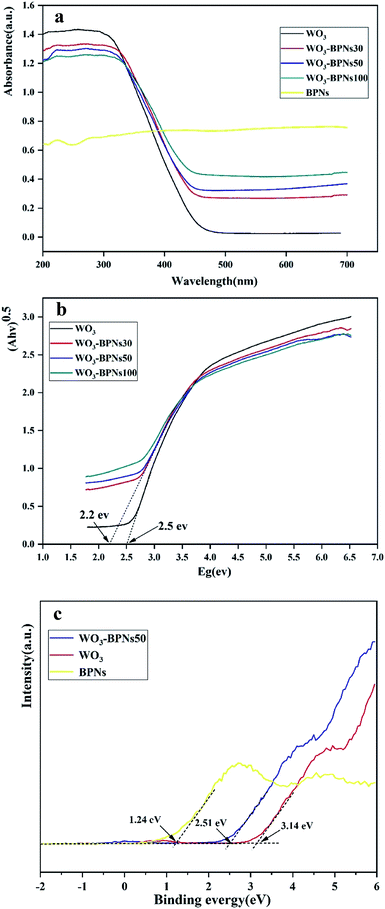 |
| Fig. 5 UV-vis diffuse reflectance spectra of WO3 and WO3–BPNs (a), and plot of the (αhv)0.5 versus hv (b), VBXPS spectra of WO3, BPNs and WO3–BPNs50 (c). | |
The XPS valence band spectrum shows that the valence band edge potentials (EVB) of WO3, BPNs and WO3–BPNs50 are 3.14, 1.24 and 2.51 eV, as shown in Fig. 5c. The valence band edge potential (EVB) of WO3–BPNs50 was shifted to a lower energy by 0.63 eV compared to that of WO3. According to the bandgap energy and empirical formulas (EVB = ECB + Eg), the conduction band edge potentials (ECB) of WO3, BPNs and WO3–BPNs were 0.63, 0.74 and 0.31 eV respectively. The reduction of the WO3–BPNs50 bandgap energy could broaden the spectral response range and be photoexcited by long-wavelength light.
3.1.3 Adsorption experiment and specific surface aperture analysis (BET). The adsorption capacity of the WO3–BPNs sample was studied by adsorption experiment and specific surface pore size analysis. As shown in Fig. 6a, the adsorption of RhB and MET by the samples mainly occurred in the first 10 minutes, and the subsequent adsorption gradually became gentle and reached equilibrium within 30 minutes. The WO3–BPNs sample had a relatively strong adsorption capacity for RhB and the maximum adsorption capacity was 19.8%, while the maximum adsorption for MET was only 11.8%. The adsorption capacity of WO3–BPNs sample for RhB increased with the increased of BPNs doping amount in the composite sample. However, the photosensitization phenomenon occurred on the RhB surface under visible light, and a large amount of RhB adsorbed on the catalyst surface may have a negative impact on the degradation performance.31
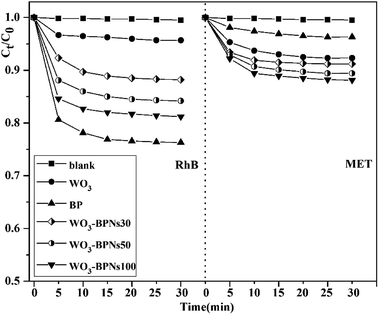 |
| Fig. 6 Adsorption of RhB and MET by all samples. | |
Fig. 7 shows the nitrogen absorption–desorption isotherms and corresponding pore size distribution curves of WO3 and WO3–BPNs50 sample. The adsorption and desorption isotherms of WO3 and WO3–BPNs50 sample were all of type IV, and there was a sharp rise at the relative pressure of about 0.9 Pa. In addition, there was no obvious difference in the pore size distribution of WO3 and WO3–BPNs50 sample. The BET analysis results showed that the specific surface area of WO3 and WO3–BPNs50 samples were 13.97 and 10.50 m2 g−1, respectively. The specific surface area of WO3–BPNs50 sample was lower than that of WO3 due to the presence of BPNs.
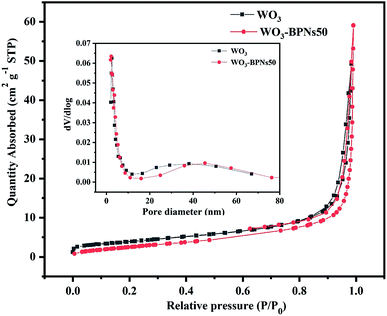 |
| Fig. 7 N2 absorption–desorption isotherms and corresponding pore-size distribution curves for WO3 and WO3–BPNs50. | |
3.2 Photocatalytic activities study
All samples were examined for photocatalytic activity by photo-degrading RhB and MET solutions under visible-light. The photocatalytic activities of RhB and MET for all samples (WO3, BP, and various amounts (mL) of BPNs dispersion in WO3–BPNs composites) are presented in Fig. 8a and b. It can be observed that the photodegradation performance was optimal when the dispersion amount of BPNs was 50 mL. As observed in Fig. 8a, 92% RhB was photodegraded by WO3–BPNs50 composite material within 120 minutes, while only 47% reduction was achieved by pure WO3. Similarly, 87% MET was photodegraded by WO3–BPNs composite material, while it was only 37% by pure WO3, as shown in Fig. 8b. More doping of BPNs (WO3–BPNs100) decreased the photocatalytic efficiency compared with the WO3–BPNs50 sample. Excessive BPNs will stimulate more photo-generated holes on the surface of BPNs. The results of VBXPS tests indicated that the valence band edge potential of BPNs was only 1.24 eV, and the oxidation ability of photogenerated holes (h+) on the surface of BPNs was consequently poor.
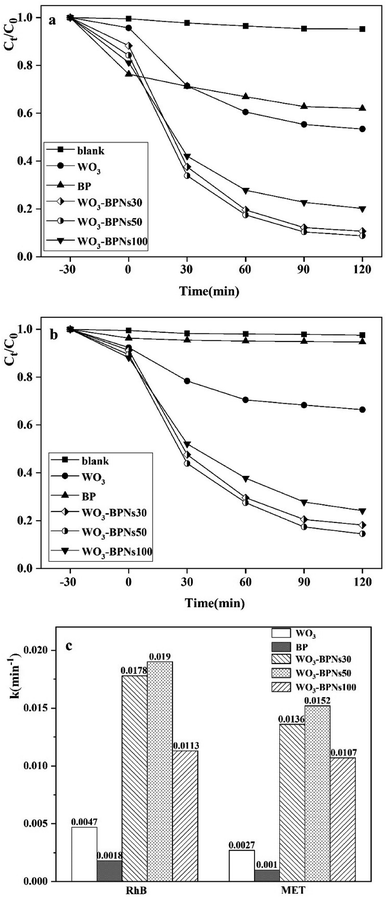 |
| Fig. 8 Photocatalytic degradation of RhB (a) and MET (b) using WO3, BP and WO3–BPNs samples. (c) First order rate constant k (min−1) of all samples for RhB and MET. | |
The first-order rate constants k (min−1) of photodegradation RhB and MET of all samples were calculated by the first-order equation,32 as shown in Fig. 8c.
where
C0 is the initial concentration of RhB and MET, and
C is the concentration of the solution at time
t. During the photodegradation of RhB and MET, the maximum values of the first-order reaction rate constants
k were 0.019 and 0.0152 min
−1 respectively, corresponding to the WO
3–BPNs50 sample.
The recycling experiments were conducted to explore the stability of the synthesized WO3–BPNs50 composite under visible-light. The photocatalyst WO3–BPNs50 was recovered by centrifugation after each trial, and a recycling test was performed under the same conditions as in the photodegradation experiment of RhB and MET described above. As shown in Fig. 9, WO3–BPNs50 could still maintain 97.9% and 97.4% degradation performance of RhB and MET after three cycles of experiments compared with their first photodegradation results. In addition, the X-ray diffraction pattern confirmed that there was no change in the structure of the sample before and after the photocatalytic reaction in Fig. 10. These indicate that the WO3–BPNs50 composite had high stability after the photocatalytic reaction.
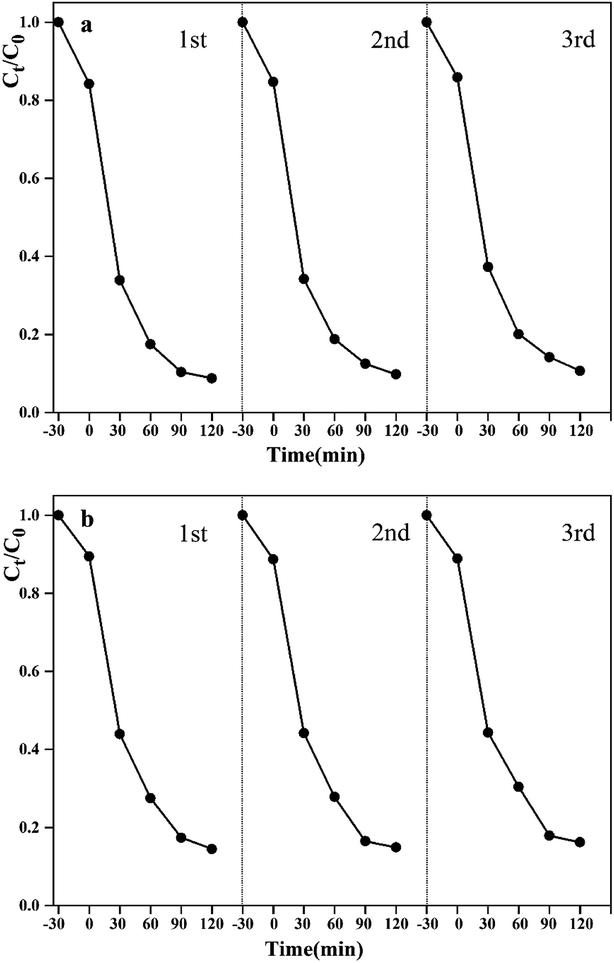 |
| Fig. 9 Stability experiment of WO3–BPNs50 on photocatalytic degradation of RhB (a) and MET (b) under visible-light. | |
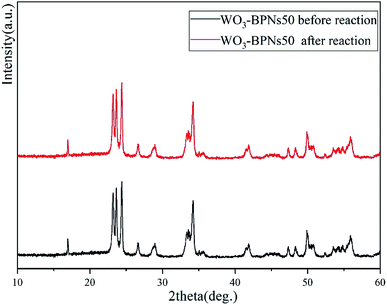 |
| Fig. 10 XRD patterns of the WO3–BPNs50 sample before reaction and after photocatalytic reaction under visible-light. | |
3.3 The photocatalytic mechanisms of WO3–BPNs
The photocatalytic species capture experiment was carried out under visible-light. Methanol, p-benzoquinone and isopropanol were selected as scavengers for the holes (h+), superoxide radicals (·O2−), and hydroxyl radicals (·OH) respectively in degrading RhB and MET.8 It can be observed that the photodegradation performance of the WO3–BPNs composite was not significantly inhibited by adding p-benzoquinone, while that was inhibited after adding methanol and isopropanol in Fig. 11a and b. The results suggested that the h+ and ·OH were the main active species during the photodegradation of RhB and MET.
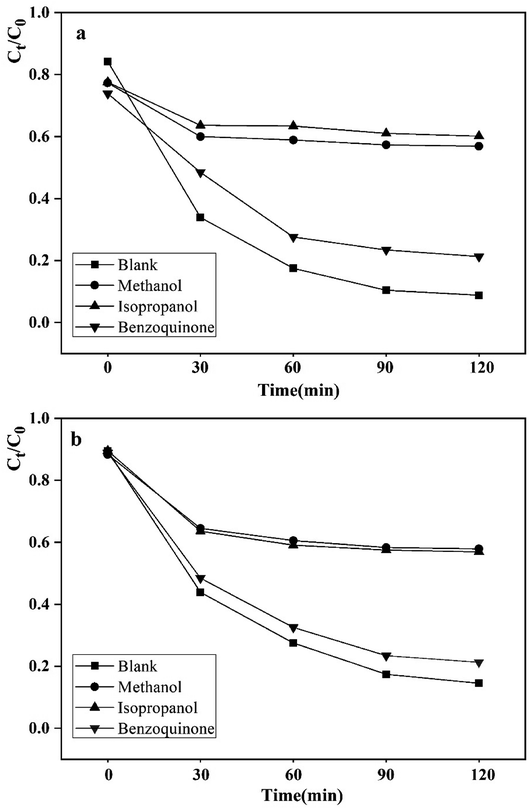 |
| Fig. 11 Effects of radical scavengers on the performance of the WO3–BPNs50 in degrading RhB (a) and MET (b). | |
The photocatalytic degradation mechanism of the WO3–BPNs composite is schematically described in Fig. 12. Photo-generated electron–hole pairs will be produced inside the semiconductor as light is irradiated on the surface of the WO3–BPNs composite. The photogenerated electrons (e−) in the conduction band of WO3 were transferred to the surface of BPNs, because the conduction band edge potential of BPNs (0.74 eV) was higher than WO3 (0.64 eV).28 However, the photogenerated holes (h+) in the WO3 valence band were difficult to transfer to the surface of the BPNs since the valence band edge potential of the WO3 (3.14 eV) was much higher than that of BPNs (1.24 eV), resulting in a longer photogenerated hole (h+) transfer path. Photogenerated holes (h+) on the surface of BPNs cannot oxidize OH− to form ·OH radicals (EOH−/·OH = 2.4 eV vs. NHE).33 Therefore, photogenerated holes (h+) on the surface of WO3 played a major role to oxidize OH− to form ·OH radicals, which could further oxidize RhB and MET. In addition, the photo-generated electrons (e−) on the conduction band of BPNs and WO3 could not react with O2 to generate ·O2− radicals, because the redox potential of O2 to ·O2− (−0.33 eV vs. NHE) was lower than the conduction edge potential of BPNs (0.74 eV) and WO3 (0.64 eV).34 ·O2− radicals were not the main active species of photodegradation reaction of RhB and MET, corresponding to the results of the active species capture experiment.
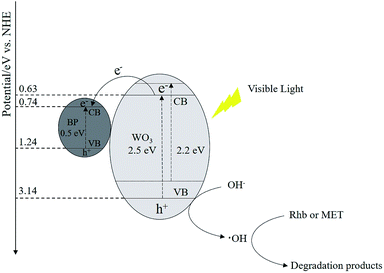 |
| Fig. 12 Schematic photodegradation mechanism of the synthesized WO3–BPNs. | |
The photo-generation of electrons and holes mainly depends on the absorption of light energy higher than the bandgap energy of the semiconductor material, and the lower bandgap energy is more likely to stimulate the generation of photogenerated electron–hole pairs and improve photocatalytic activity.9 The calculated bandgap energy of WO3–BPNs50 and WO3 was 2.2 eV and 2.5 eV, respectively. The valence band edge potential (EVB) and calculated band gap energy of the WO3–BPNs50 sample were lower than those of WO3, which could broaden the spectral response range and be photoexcited by long-wavelength light. Moreover, BPNs in the WO3–BPNs50 heterojunction acted as a co-catalyst to receive and transfer photogenerated electrons in the photocatalytic reaction process, which effectively reduced the recombination of photogenerated electron–hole pairs and significantly enhanced the photocatalytic performance.23 The XPS results suggested that the electron density increased and decreased in WO3 and BPNs, which also explained the transfer of electrons in the WO3–BPNs composite. Therefore, the reason for improving photocatalytic performance of the WO3–BPNs composite was majorly attributed the lower optical bandgap energy and BPNs as a co-catalyst in the WO3–BPNs heterojunction.
3.4 Degradation pathways and intermediates of MET
The toxic and harmful intermediate products may be generated during the degradation of organic pollutants.35,36 MET, unlike industrial dyes (RhB), is widely used in both hospitals and households, which eventually enters surface waters, including drinking water sources. Therefore, the identification of MET degradation intermediates is significantly for ensuring the safety of water supply. In this study, LC-MS technology was used to detect and identify potential MET intermediates.
As shown in Fig. 13, the chromatograms of total ion (TIC), MET, and six intermediates were obtained with LC-MS at unequal retention times. The mass-to-charge ratios (m/z) of these seven compounds were 268, 134, 252, 238, 254, 284, and 300 m/z, respectively. Combined with the structural characteristics of the MET molecule, the structures of the six intermediates were identified (Table 1). The photocatalytic degradation pathway for MET was proposed according to the determination of MET intermediates and the photodegradation mechanism of the WO3–BPNs composites in Fig. 14.
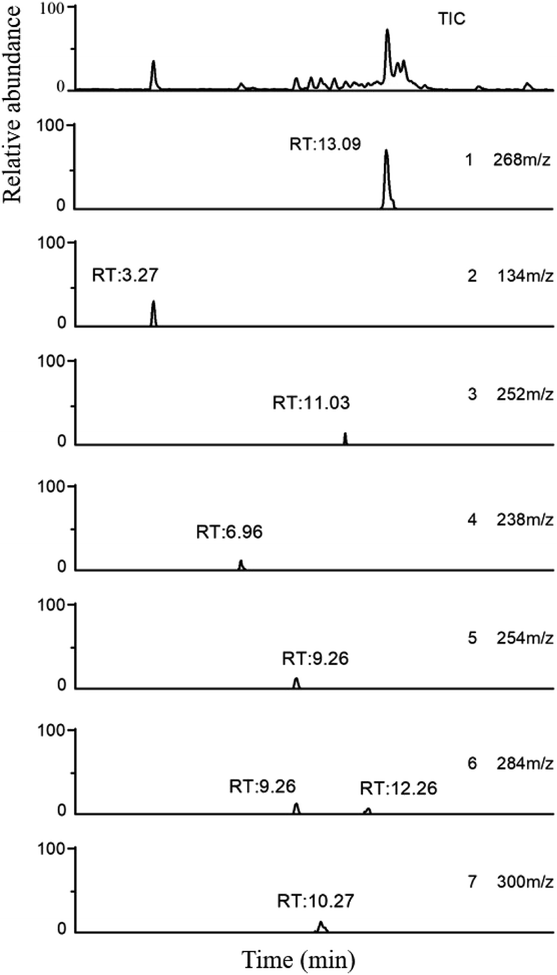 |
| Fig. 13 LC-MS total ion chromatogram (TIC) and extracted ions chromatograms of MET (1) and its intermediates (2–7) using WO3–BPNs. | |
Table 1 Proposed structures of the intermediates for the photocatalytic degradation of MET using WO3–BPNs
Compound |
Molecular formula |
[M + H]+ m/z |
Structural formula |
1 |
C15H25NO3 |
268 |
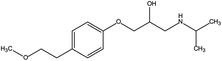 |
2 |
C6H15NO2 |
134 |
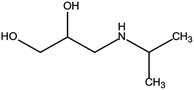 |
3 |
C14H21NO3 |
252 |
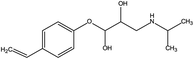 |
4 |
C13H19NO3 |
238 |
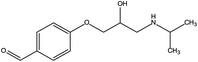 |
5 |
C13H19NO4 |
254 |
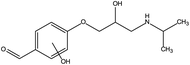 |
6 |
C15H25NO4 |
284 |
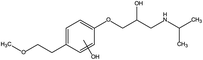 |
7 |
C15H25NO5 |
300 |
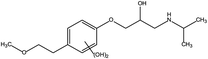 |
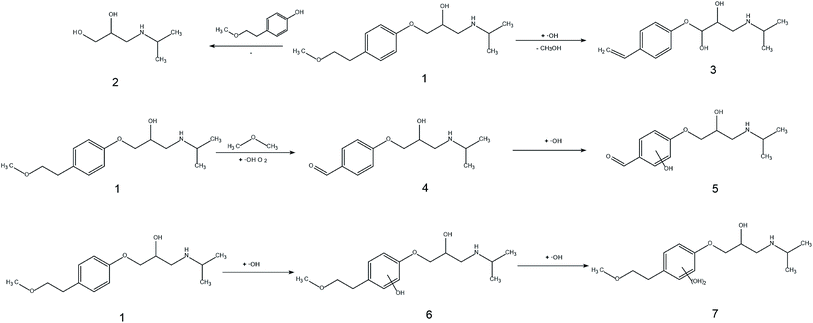 |
| Fig. 14 Tentative pathways for the photocatalytic degradation of MET using WO3–BPNs. | |
First, the MET molecule (1) was attacked by the ·OH radical, which caused the broken of the C–C bond in the aliphatic portion of the MET molecule and the producing amino-diol (2) intermediates.37,38 The corresponding phenol produced by MET was also intermediate.39 Intermediate (3) may be formed due to the oxidation of ·OH radicals in the aliphatic part of MET to C atoms near ether oxygen and the loss of methanol, which formed a characteristic peak at the retention time of 11.03 minutes (Fig. 11). Second, intermediate (4) and (5) with 238 and 254 m/z were identified from the peaks formed at the retention time of 6.96 minutes and 9.26 minutes, respectively, in Fig. 11. The combine O-atom addition and the loss of ether group, owing to ·OH attacking the alkyl group, led to form intermediate (4). Intermediate (5) could be formed by the attack of ·OH on the aromatic ring of intermediate (4).39 Intermediate (4) had two substituents on the aromatic ring, in which the –CHO substituent had a meta-orientating character and electron-withdrawing, and the other substituent was ortho/para orientating character and an alkoxy group with an electron-donating.40 Therefore, ·OH was more conducive to attack the meta position of –CHO group under the combined action of two substituents, forming the intermediate (5). In addition, the mono-hydroxylated intermediate (6) with 284 m/z and the di-hydroxylated intermediate 7 with 300 m/z was identified, which was also caused by the ·OH radical attack on the benzene ring of the MET molecule.
4 Conclusion
The WO3–BPNs heterojunction composite was successfully prepared using a combined hydrothermal, liquid phase exfoliating and co-precipitation method. The as-obtained WO3–BPNs composite was characterized by using XRD, SEM, AFM, XPS, BET and UV-vis. The results showed that the valence band edge potential of the WO3–BPNs50 was shifted to a lower energy and the bandgap energy of the WO3–BPNs50 was lower than that of WO3, which could broaden the spectral response range and be photoexcited by long-wavelength light. The WO3–BPNs sample exhibited promoted photocatalytic activity in photo-degrading RhB and MET as comparison with pure WO3 and BPNs under visible-light. The maximum RhB and MET removal efficiency were achieved at 92% and 87% for the WO3–BPNs50 sample within 120 minutes. Three cycles of experiments and XRD measurements of the samples before and after photodegradation suggested the excellent stability of the WO3–BPNs50 sample. The photodegradation mechanisms of the WO3–BPNs composite were also proposed. BPNs in WO3–BPNs50 heterojunction acted as a co-catalyst to receive and transfer photogenerated electrons in the photocatalytic reaction process, which effectively reduced the recombination of photogenerated electron–hole pairs and significantly enhanced the photocatalytic performance. Finally, six intermediates in the MET photodegradation process were detected by LC-MS, and the tentative pathways were proposed for the photo-degradation of MET.
Conflicts of interest
There are no conflicts to declare.
Acknowledgements
The work was supported by the National Natural Science Foundation of China (No. 41771502).
References
- L. Jianga, X. Yuana, G. Zenga, Z. Wua and L. Jie, Metal-free efficient photocatalyst for stable visible-light photocatalytic degradation of refractory pollutant, Appl. Catal., B, 2017, 221, 715–725 CrossRef.
- A. Fujishima and K. Honda, Electrochemical Photolysis of Water at a Semiconductor Electrode, Nature, 1972, 238, 37–38 CrossRef CAS PubMed.
- A. Mills and S. L. Hunte, An Overview of Semiconductor Photocatalysis, J. Photochem. Photobiol., A, 1997, 108, 1–35 CrossRef CAS.
- D. Jiang, S. Zhang and H. Zhao, Photocatalytic Degradation Characteristics of Different Organic Compounds at TiO2 Nanoporous Film Electrodes with Mixed Anatase/Rutile Phases, Environ. Sci. Technol., 2007, 41, 303–308 CrossRef CAS PubMed.
- F. Han, H. Li, F. Li, J. Yang and L. Zhong, Synthesis of S-doped WO3 nanowires with enhanced photocatalytic performance towards dye degradation, Chem. Phys. Lett., 2016, 651, 183–187 CrossRef CAS.
- X. Zhang, et al., Effect of aspect ratio and surface defects on the photocatalytic activity of ZnO nanorods, Sci. Rep., 2014, 4, 4596 CrossRef PubMed.
- L. Mao, X. Cai, S. Yang, K. Han and J. Zhang, Black phosphorus-CdS-La2Ti2O7 ternary composite: effective noble metal-free photocatalyst for full solar spectrum activated H2 production, Appl. Catal., B, 2018, 242, 441–448 CrossRef.
- L. Gao, et al., Preparation of heterostructured WO3/TiO2 catalysts from wood fibers and its versatile photodegradation abilities, Sci. Rep., 2017, 7, 1102 CrossRef PubMed.
- R. Asahi, T. Morikawa, T. Ohwaki, K. Aoki and Y. Taga, Visible-light photocatalysis in nitrogen-doped titanium oxides, Science, 2001, 293, 269–271 CrossRef CAS PubMed.
- Q. Shi, D. Yang, Z. Jiang and J. Li, Visible-light photocatalytic regeneration of NADH using P-doped TiO2 nanoparticles, J. Mol. Catal. B: Enzym., 2006, 43, 44–48 CrossRef CAS.
- A. Garzon-Roman, C. Zuñiga-Islas and E. Quiroga-González, Immobilization of doped TiO2 nanostructures with Cu or In inside of macroporous silicon using the solvothermal method: morphological, structural, optical and functional properties, Ceram. Int., 2020, 46, 1137–1147 CrossRef CAS.
- C. S. Park, V. R. Shrestha, S. S. Lee, E. S. Kim and D. Y. Choi, Omnidirectional color filters capitalizing on a nano-resonator of Ag-TiO2-Ag integrated with a phase compensating dielectric overlay, Sci. Rep., 2015, 5, 8467 CrossRef CAS PubMed.
- W. Liang, L. Jian and Y. Jin, Photo-catalytic degradation of gaseous formaldehyde by TiO2/UV, Ag/TiO2/UV and Ce/TiO2/UV, Build. Environ., 2012, 51, 345–350 CrossRef.
- E. S. Reich, Phosphorene excites materials scientists, Nature, 2014, 506, 19 CrossRef PubMed.
- M. H. H. Ali, A. D. Al-Afify and M. E. Goher, Preparation and characterization of graphene-TiO2 nanocomposite for enhanced photodegradation of rhodamine-B dye, Egypt. J. Aquat. Res., 2018, 44, 263–270 CrossRef.
- S. Kanda, et al., Facile synthesis and catalytic activity of MoS2/TiO2 by a photodeposition-based technique and its oxidized derivative MoO3/TiO2 with a unique photochromism, J. Colloid Interface Sci., 2011, 354(2), 607–610 CrossRef CAS PubMed.
- L. Liao, et al., High-speed graphene transistors with a self-aligned nanowire gate, Nature, 2010, 467, 305–308 CrossRef CAS PubMed.
- B. Radisavljevic, A. Radenovic, J. Brivio, V. Giacometti and A. Kis, Single-layer MoS2 transistors, Nat. Nanotechnol., 2011, 6, 147–150 CrossRef CAS PubMed.
- F. Xia, H. Wang and Y. Jia, Rediscovering black phosphorus as an anisotropic layered material for optoelectronics and electronics, Nat. Commun., 2014, 5, 4458 CrossRef CAS PubMed.
- J. Qiao, X. Kong, Z. X. Hu, F. Yang and W. Ji, High-mobility transport anisotropy and linear dichroism in few-layer black phosphorus, Nat. Commun., 2014, 5, 4475 CrossRef CAS PubMed.
- D. J. Perello, S. H. Chae, S. Song and Y. H. Lee, High-performance n-type black phosphorus transistors with type control via thickness and contact-metal engineering, Nat. Commun., 2015, 6, 7809 CrossRef CAS PubMed.
- L. Li, et al., Black phosphorus field-effect transistors, Nat. Nanotechnol., 2014, 9, 372–377 CrossRef CAS PubMed.
- H. Uk Lee, et al., Stable semiconductor black phosphorus (BP)@titanium dioxide (TiO2) hybrid photocatalysts, Sci. Rep., 2015, 5, 8691 CrossRef PubMed.
- J. Hu, et al., Z-Scheme 2D/2D Heterojunction of Black Phosphorus/Monolayer Bi2WO6 Nanosheets with Enhanced Photocatalytic Activities, Angew. Chem., 2019, 131, 2095–2099 CrossRef.
- M. Zhu, X. Cai, M. Fujitsuka, J. Zhang and T. Majima, Au/La2Ti2O7 Nanostructures Sensitized with Black Phosphorus for Plasmon-Enhanced Photocatalytic Hydrogen Production in Visible and Near-Infrared Light, Angew. Chem., Int. Ed., 2017, 56, 2064 CrossRef CAS PubMed.
- Z. Ying, D. Zhang, X. Xu and B. Zhang, Morphology control and photocatalytic characterization of WO3 nanofiber bundles, Chin. Chem. Lett., 2018, 29, 40–44 CrossRef.
- P. Yasaei, et al., High-quality black phosphorus atomic layers by liquid-phase exfoliation, Adv. Mater., 2015, 27, 1887–1892 CrossRef CAS PubMed.
- M. Zhu, C. Zhai, M. Fujitsuka and T. Majima, Noble Metal-Free Near-Infrared-Driven Photocatalyst for Hydrogen Production Based on 2D Hybrid of Black Phosphorus/WS2, Appl. Catal., B, 2017, 221, 645–651 CrossRef.
- G. Carbajal-Franco, M. Eastman and C. V. Ramana, Structure and optical properties of iron oxide films prepared by a modified wet-chemical method, Ceram. Int., 2013, 39, 4581–4587 CrossRef CAS.
- T. Sakthivel, X. Y. Huang, Y. C. Wu and S. Rtimi, Recent progress in black phosphorus nanostructures as environmental photocatalysts, Chem. Eng. J., 2020, 379, 122297 CrossRef CAS.
- J.-M. Wu and T.-W. Zhang, Photodegradation of rhodamine B in water assisted by titania films prepared through a novel procedure, J. Photochem. Photobiol., A, 2004, 162, 171–177 CrossRef CAS.
- Y. Xu, W. Wen and J. M. Wu, Titania nanowires functionalized polyester fabrics with enhanced photocatalytic and antibacterial performances, J. Hazard. Mater., 2018, 343, 285–297 CrossRef CAS PubMed.
- S. Meng, J. Zhang, S. Chen, S. Zhang and W. Huang, Perspective on construction of heterojunction photocatalysts and the complete utilization of photogenerated charge carriers, Appl. Surf. Sci., 2019, 476, 982–992 CrossRef CAS.
- M. J. Islam, et al., An oxygen-vacancy rich 3D novel hierarchical MoS2/BiOI/AgI ternary nanocomposite: enhanced photocatalytic activity through photogenerated electron shuttling in a Z-scheme manner, Phys. Chem. Chem. Phys., 2016, 18, 24984–24993 RSC.
- X. Cai, J. Ye, G. Sheng and W. Liu, Time-dependent degradation and toxicity of diclofop-methyl in algal suspensions: emerging contaminants, Environ. Sci. Pollut. Res. Int., 2009, 16, 459–465 CrossRef CAS PubMed.
- H. Li, Y. Yuan, C. Shen, Y. Wen and H. Liu, Enantioselectivity in toxicity and degradation of dichlorprop-methyl in algal cultures, J. Environ. Sci. Health, Part B, 2008, 43, 288–292 CrossRef CAS PubMed.
- H. Yang, et al., Photocatalytic degradation kinetics and mechanism of environmental pharmaceuticals in aqueous suspension of TiO2: a case of beta-blockers, J. Hazard. Mater., 2010, 179, 834–839 CrossRef CAS PubMed.
- V. Romero, et al., Photocatalytic treatment of metoprolol and propranolol, Catal. Today, 2011, 161, 115–120 CrossRef CAS.
- B. Abramovic, et al., Photocatalytic degradation of metoprolol tartrate in suspensions of two TiO2-based photocatalysts with different surface area. Identification of intermediates and proposal of degradation pathways, J. Hazard. Mater., 2011, 198, 123–132 CrossRef CAS PubMed.
- M. M. Huber, S. Canonica, G. Y. Park and U. von Gunten, Oxidation of pharmaceuticals during ozonation and advanced oxidation processes, Environ. Sci. Technol., 2003, 37, 1016–1024 CrossRef CAS PubMed.
|
This journal is © The Royal Society of Chemistry 2020 |
Click here to see how this site uses Cookies. View our privacy policy here.