DOI:
10.1039/D0RA05581F
(Paper)
RSC Adv., 2020,
10, 29745-29750
Detection of tyrosine and monitoring tyrosinase activity using an enzyme cascade-triggered colorimetric reaction†
Received
26th June 2020
, Accepted 30th July 2020
First published on 12th August 2020
Abstract
The aromatic amino acid tyrosine is an essential precursor for the synthesis of catecholamines, including L-DOPA, tyramine, and dopamine. A number of metabolic disorders have been linked to abnormal tyrosine levels in biological fluids. In this study, we developed an enzyme cascade-triggered colorimetric reaction for the detection of tyrosine, based on the formation of yellow pigment (betalamic acid) and red fluorometric betaxanthin. Tyrosinase converts tyrosine to L-DOPA, and DOPA-dioxygenase catalyzes oxidative cleavage of L-DOPA into betalamic acid. Response is linear for tyrosine from 5 to 100 μM, and the detection limit (LOD) is 2.74 μM. The enzyme cascade reaction was applied to monitor tyrosinase activity and tyrosinase inhibition assays. Lastly, the performance of the proposed biosensor proved successful in the analysis of urine samples without the need for pre-treatment.
1. Introduction
Tyrosine (Tyr) is an aromatic amino acid critical to the synthesis of compounds such as neurotransmitters and melanin.1,2 Abnormal Tyr concentrations in plasma/urine can also be used as a biomarker in the detection of various diseases, such as alkaptonuria, tyrosinemia, and liver disease.3–5 Therefore, an easy quantification assay of Tyr is important and necessary.
Researchers have developed numerous methods for the quantification of Tyr, including fluorometric methods,6–10 high-performance liquid chromatography,11 electrochemical detection,12–14 colorimetric assays,15 and chemiluminescence;16 however, implementing such methods is expensive and complex. In this study, we sought to facilitate the quantification of Tyr for diagnostic purposes by developing an enzyme-based colorimetric biosensor to reduce the possible interferences from catecholamine metabolites. The approach has proven highly effective, particularly in real-time detection.
Tyrosinase is a copper-containing oxidase, capable of catalyzing the hydroxylation of monophenol to catechol, and the subsequent oxidation to o-quinone,17 which carries a chromophoric group suitable as a biosensor readout.18 However, tyrosine and tyrosine analogs (e.g. tyramine) are also substrates of tyrosinase, and o-quinone is susceptible to non-enzymatic reactions resulting in the spontaneous formation of melanin.19 We therefore developed a sequential cascade reaction involving multiple enzymes to serve as a signal filter and amplifier.20,21
Tyrosinase converts Tyr to L-DOPA; i.e., the substrate of DOPA-dioxygenase (DOD). Note that DOD is an oxidase that opens the cyclic ring between carbon 4 and 5 on L-DOPA, resulting in the synthesis of yellow betalamic acid,22–24 which is a precursor of betalains/betaxanthins used as readouts of optical biosensors (Scheme 1).25–27 Thus, we developed an enzyme cascade-triggered colorimetric biosensor for Tyr involving the synthesis of yellow betalamic acid and red betaxanthins products. We then evaluated the proposed biosensor in terms of Tyr detection.
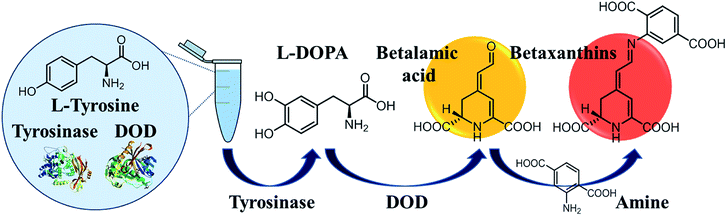 |
| Scheme 1 Enzyme cascade-triggered colorimetric reaction for Tyr analysis based on the synthesis of betalamic acid and betaxanthins. | |
2. Experimental methods
2.1 Chemicals
2-Aminophthalic acid (2-AIPA), epinephrine, glucose oxidase, lysozyme, norepinephrine, para-aminobenzoic acid (PABA), tyrosinase, and tryptophan were purchased from Sigma-Aldrich. L-Ascorbic acid (AA), carbenicillin, iron(II) sulfate, isopropyl β-D-1-thiogalactopyranoside (IPTG), and protease inhibitor cocktail were purchased from Amresco. Glutamic acid (Glu), L-DOPA, and phenylalanine (Phe) were purchased from ACROS. para-Phenylenediamine and tyramine were purchased Alfa Aesar. Calcium chloride, magnesium chloride hexahydrate, potassium chloride, and tyrosine were purchased from Merck. Sodium chloride and trypsin were purchased from VWR Life Science and Promega, respectively. Artificial urine (AU) was purchased from GE Healthcare Life Sciences.
2.2 Expression and purification of DOPA-dioxygenase
E. coli BL21 (DE3) (Invitrogen) carrying pET21a-DOD-histag plasmid was inoculated in LB medium containing 1 mM of carbenicillin at 37 °C for 16–18 h. Then, the overnight culture was diluted in fresh LB (1
:
100; v%) with 1 mM of carbenicillin and incubated at 37 °C until O.D.600 reached 0.4–0.6, and 1 mM of IPTG was used to induce protein expression at 18 °C for 22 h. Next, the pellets were lysed in lysis buffer containing protease inhibitor by sonication after the harvest of the cells by centrifugation at 9000 rpm and 4 °C (lysis buffer containing 25 mM of Tris–HCl with 200 mM of NaCl). Then, the lysate was centrifuged at 9000 rpm and 4 °C for 1 h to remove cell debris. After that, HisPur™ Ni-NTA Resin Spin Columns (Thermo Scientific) were used to purify the proteins in the supernatant, which was filtered through a 0.22 μm syringe filter, and the purified proteins were dialyzed against 25 mM of phosphate buffer with 100 mM of NaCl (pH 6.8) for 24 h.
2.3 Detection of tyrosine by the enzyme cascade-triggered colorimetric reaction and interference study
This colorimetric biosensor for Tyr analysis was incubated with various concentrations of Tyr in the reaction medium containing sodium phosphate buffer (25 mM) with NaCl (100 mM) (pH 6.8), iron(II) sulfate (0.5 mM), ascorbic acid (10 mM),27 tyrosinase (224 μM), and DOD (12.5 μM). The optical signal was detected by measuring the absorption spectroscopy in 96-well plates in a Synergy HT microplate reader (BioTek, Winooski, VT) after the enzyme cascade-triggered reaction at room temperature for 0.5 h. The reaction medium without Tyr was mixed with tyramine, norepinephrine, epinephrine, phenethylamine, phenylalanine, tryptophan and ascorbic acid at 100 μM for interference tests respectively.
2.4 Characterization and quantification of betaxanthins
The reaction solutions containing Tyr at various concentrations were mixed with 10 mM primary amines. The fluorescence intensity of betaxanthins was detected on F-7000 fluorescence spectrophotometer (HITACHI) after the enzymatic conversion of Tyr to betalamic acid and further the non-enzymatic condensation of betalamic acid with amines to synthesis betaxanthins at room temperature for 1 h.
2.5 Preparation of artificial urine samples
Artificial urine was diluted 10-fold with sodium phosphate buffer before all measurements.
3. Results and discussion
3.1 Characterization and optimization of betalamic acid production
Using absorption spectroscopy, we began by determining the optimal conditions for the enzyme cascade system based on the detection of the yellow betalamic acid. Absorption intensity at 430 nm was shown to increase with the concentration of Tyr. The colorimetric sensor system was also assessed under various pH values in order to determine the optimal buffer conditions (Fig. S1A†). Our results indicate that pH 6.8 is ideal for this system.
We then sought to optimize the concentrations of tyrosinase and DOD in the proposed system. We began by varying the concentration of tyrosinase between 0 to 224 μM in the presence of DOD (8 μM) and Tyr (50 μM) following incubation for a period of 0.5 h. We observed a notable increase in absorption intensities at 430 nm at the highest tyrosinase concentration of 224 μM (Fig. S1B†). We then increased the concentrations of DOD from 8 to 20 μM in the presence of tyrosinase (224 μM) and Tyr (50 μM) (Fig. S1C†). We did not observe a notable increase in λ430 signal intensity when the DOD concentration was increased beyond 12.5 μM. Thus, throughout the remainder of the study, the enzyme cascade-based biosensor was implemented using 224 μM of tyrosinase and 12.5 μM of DOD.
We closely monitored the λ430 signal intensity throughout the time-course of the reaction (Fig. S1D†). Note that the initial increase in intensity plateaued within 1 h. Thus, in all subsequent experiments, we recorded the absorption intensity at 430 nm after incubation for 0.5 h in order to enable rapid detection with reasonable incubation. The results suggest the completion time of proposed sensor is comparable to methods which are commonly used.
3.2 Determining substrate specificity of the tyrosine sensing system
Under optimal experimental conditions, a dramatic increase in the absorption intensity of betalamic acid was observed upon the concentration of Tyr increased (Fig. 1A). The absorption intensity of betalamic acid was shown to increase linearly with the addition of Tyr at concentrations of 5 to 100 μM (R2 = 0.99) (Fig. 1B). According to the 3sb/slope, the detection limit (LOD) was 2.74 μM (sb: standard deviation of background and the slope in the calibration plot).
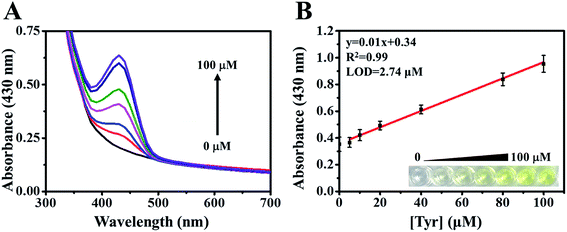 |
| Fig. 1 (A) Absorption spectra of reaction mixtures with various concentrations of Tyr (0, 5, 10, 20, 40, 80, and 100 μM). (B) The linear range for Tyr was between 5 and 100 μM, and LOD was 2.74 μM. The corresponding photograph under the natural light in the presence of Tyr. | |
The selectivity of the biosensor was evaluated by mixing the reaction medium with interfering substrates, including structural analogs (tyramine, norepinephrine, epinephrine, and phenethylamine) and metabolic intermediates (phenylalanine, tryptophan, and ascorbic acid) at concentrations of 100 μM, respectively. It was found that tyramine (structurally similar to Tyr) subtlety increased the intensity of the λ430 signal (Fig. 2A). Therefore, to test the response to interference in the co-presence of tyramine and Tyr, we compared the enhanced intensities of λ430 with that of a single substrate and a mixture of the two. In addition, we studied the responses using various tyramine to Tyr ratios (1, 10, 50, 100 μM) and Tyr (100 μM). As shown in Fig. 2B, the absorbance intensity at 430 nm has not interfered. Thus, the effect of tyramine can be considered negligible.
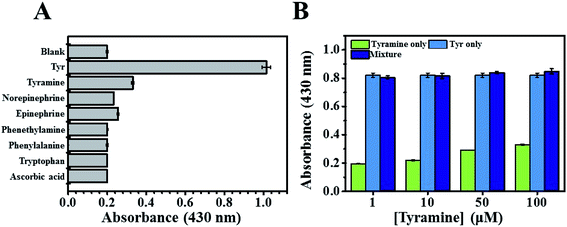 |
| Fig. 2 (A) The selectivity of the enzyme cascade-based system in the presence of other substrates at 100 μM was investigated. The intensity of absorbance at 430 nm after 0.5 h reaction was recorded. (B) The intensities of λ430 in the co-presence of Tyr at 100 μM and 1/10/50/100 μM of tyramine. | |
3.3 Stability of the enzyme cascade-based sensor
Stability is a crucial concern in assessing any biosensor. In this study, we compared the stability of the proposed enzyme cascade-triggered biosensor in the detection of Tyr (based on the formation of betalamic acid) and the tyrosinase system (based on the formation of dopachrome). Tyrosinase could lead to the hydroxylation of Tyr to L-DOPA and subsequently catalyze the oxidation of L-DOPA to dopachrome leading to an increase in absorption at 475 nm (Fig. S2A†).17,18 We therefore added Tyr (at various concentrations) to a reaction medium containing phosphate buffered saline (pH 7.4) and 224 μM tyrosinase. We then observed the stability of the two systems at regular intervals by measuring λ430 of betalamic acid and λ475 of dopachrome in the presence of Tyr at a concentration of 100 μM (Fig. S2B†). The intensity of λ475 (tyrosinase system biosensor) presented a steady decrease after 7 h incubation. By contrast, the intensity of λ430 (enzyme cascade-triggered biosensor) remained constant for more than 24 h.
Again, the co-presence of tyramine and Tyr was examined by monitoring intensities of λ475 with that of a single substrate and a mixture of the two using conventional tyrosinase assays. The intensity of λ475 was shown enhancement than normal in the co-presence of tyramine at 10 μM (Fig. S2C†), indicating interference can occur for the samples containing tyramine greater than 10 μM.
3.4 Condensation of betalamic acid with amines
It has been shown that the color of betanin depends on the structural motifs in the cyclo-DOPA moiety of the pigments.28,29 We therefore mixed betalamic acid with 10 mM of primary amines phenylalanine (Phe), glutamic acid (Glu), para-aminobenzoic acid (PABA), and 2-aminoterephthalic acid (2-AIPA), which respectively present green (Phe-, Glu-) and red (PABA-, 2-AIPA-) fluorescence.27 We then monitored the green and red fluorescence intensity of betaxanthins at various time points (Fig. S3A and S3B†). The green fluorescence intensity plateaued after 21 h; however, the red fluorescence intensity peaked after 4 h. To increase detection efficiency, we selected 2-AIPA–betaxanthin for the quantification of Tyr. The optimal concentration for 2-AIPA was examined in Fig. S3C.† The red fluorescence intensities of 2-AIPA–betaxanthin was shown to increase linearly with the concentration of Tyr (from 2.5 to 20 μM) with R2 = 0.96 (Fig. 3A).
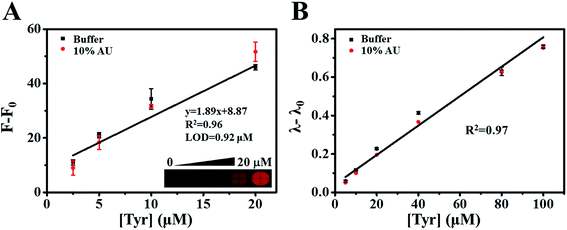 |
| Fig. 3 The intensity of (A) the red fluorescence intensities of 2-AIPA–betaxanthin (F − F0) and (B) λ − λ0 for betalamic acid was observed as a linear function with the concentrations of Tyr (F0 and λ0 represent the background absorbance and fluorescence of blank matrices). (A) The linear ranges for Tyr was from 2.5 to 20 μM, and LOD was 0.92 μM. The effectiveness of enzyme cascade-based biosensor in buffer and 10% artificial urine matrix was compared (red dots). Inset: the corresponding fluorescence photograph in the presence of Tyr. | |
3.5 The colorimetric biosensor for tyrosine detection using artificial urine matrix
Normally, the concentration of Tyr in healthy individuals ranges from 30 to 120 μM;30 however, it can exceed 200 μM in the biological fluids of patients with tyrosine metabolism disorders. We therefore tested the effectiveness of the proposed enzyme cascade-based biosensor using 10% diluted artificial urine spiked with various concentrations of Tyr. Our results revealed that the linearity, dynamic range, and sensitivity of fluorescence and absorbance intensity in complex matrices were similar to those in the buffer system (Fig. 3A and B). The calculated relative standard deviation (RSD) and recoveries using standard curves were obtained (Tables S1 and S2†). The accuracy ranges from 88–101% and 100–120% based on absorbance and fluorescence assays, respectively. This is a clear demonstration that this proposed method could be applied to the detection of Tyr in biological specimens.
3.6 Monitoring tyrosinase activity using the enzyme cascade reaction
Next, we employed the enzyme cascade reaction for the visual observation of tyrosinase activity. We first determined the optimal concentration of Tyr for the enzyme cascade reaction. We began by varying the concentration of Tyr between 125 to 1000 μM in the presence of DOD (12.5 μM) and tyrosinase (1.5 U mL−1) following incubation for a period of 1 h. As shown in Fig. S4A,† the intensities of λ430 were significantly increased when 1000 μM Tyr was introduced. Under optimal experimental conditions, a good linear relationship was observed between the intensities of λ430 and tyrosinase concentrations (Fig. S4B†). The red fluorescence intensities of 2-AIPA–betaxanthin was recorded as well to determine the tyrosinase activity (Fig. 4A). The corresponding regression coefficient is 0.99, and the LOD was 0.04 U mL−1. The selectivity and activity of the proposed sensor was investigated by using the interfering substances including trypsin, lysozyme, glucose oxidase (GOx), and metal ions (Fig. 4B). There is no noticeable change of red fluorescence intensity in the presence of interfering substances.
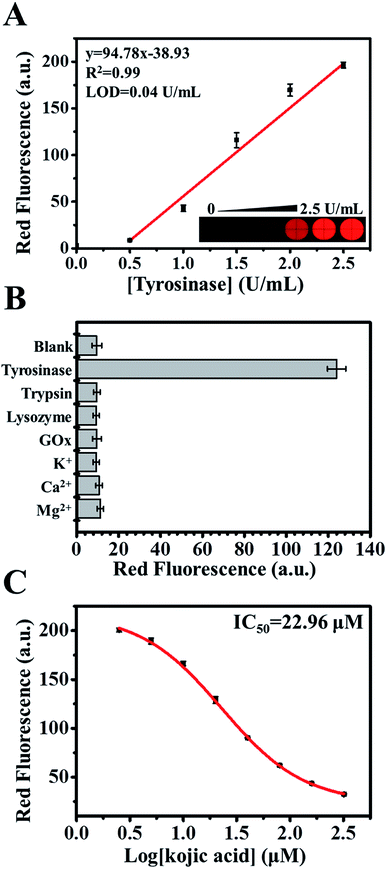 |
| Fig. 4 (A) The linear relationships between the red fluorescence intensities of 2-AIPA–betaxanthin and tyrosinase at various concentrations. Inset: the corresponding fluorescence photograph in the presence of tyrosinase. (B) The selectivity of the proposed enzyme cascade-based system in the presence of various substrates (tyrosinase, trypsin, lysozyme, GOx: 6 U mL−1; K+, Ca2+, Mg2+: 100 μM) was investigated. (C) The red fluorescence intensities of 2-AIPA–betaxanthin in the presence of Tyr (1000 μM), tyrosinase (9 U mL−1), and kojic acid at various concentrations. | |
The development of synthetic and naturally occurring tyrosinase inhibitors has attracted an increased research in the medical, agricultural, and cosmetic industries. Among many naturally occurring tyrosinase inhibitors, kojic acid has been intensively studied. We sought to examine the effectiveness of the proposed sensors in screening tyrosinase inhibitors by using kojic acid as a model compound. The red fluorescence of 2-AIPA–betaxanthin was recorded in the presence of Tyr (1000 μM), tyrosinase (9 U mL−1), and kojic acid at various concentrations (Fig. 4C). The calculated IC50 value was 22.96 μM, which is consistent with the previous reports.31,32
4. Conclusions
In this study, we have developed an enzyme cascade-triggered colorimetric biosensor for the quantification of Tyr. Tables S3 and S4† present a comparison with several sensors in terms of the linear ranges, LOD, and analysis duration. The formation of dopachrome using tyrosinase is commonly used for the detection of Tyr. However, tyrosinase shows both the hydroxylase and the oxidase activities. To overcome the specificity issues, we developed cascade-triggered sensor, which has satisfactory sensitivity and selectivity to Tyr. The proposed biosensor also proved effective in the detection of Tyr in realistic samples, and makes it possible to detect Tyr using the naked eye. Two types of signals including colorimetric and fluorescent method were examined. Although fluorescent method of 2-AIPA–betaxanthin showed a better sensitivity than colorimetric method, the analysis duration is relative longer than the colorimetric method. These findings suggest that the effectiveness and simplicity of the proposed scheme make it an attractive strategy for the development of biosensors for Tyr and tyrosinase analysis.
Conflicts of interest
There are no conflicts to declare.
Acknowledgements
This work was funded by the Ministry of Science and Technology of Taiwan under the project number 107-2113-M-003-013-MY3.
References
- J. P. Greenstein, Chemistry of the amino acids, 1961 Search PubMed.
- C. R. Scriver, The metabolic & molecular bases of inherited disease, McGraw-Hill, New York; Montreal, 2001 Search PubMed.
- A. Chakrapani and E. Holme, Inborn metabolic diseases, 2006, pp. 233–243 Search PubMed.
- K. Michitaka, A. Hiraoka, M. Kume, T. Uehara, S. Hidaka, T. Ninomiya, A. Hasebe, Y. Miyamoto, M. Ichiryu and T. Tanihira, Hepatol. Res., 2010, 40, 393–398 CrossRef CAS PubMed.
- J. B. Mistry, M. Bukhari and A. M. Taylor, Rare Dis., 2013, 1, e27475 CrossRef PubMed.
- J. A. Ambrose, Clin. Chem., 1974, 20, 505–510 CrossRef CAS.
- F. Wang, Y. Qing and Y.-X. Ci, Anal. Lett., 1992, 25, 1469–1478 CrossRef CAS.
- Y. Li, N. Cai, M. Wang, W. Na, F. Shi and X. Su, RSC Adv., 2016, 6, 33197–33204 RSC.
- X. Qin, Y. Lu, M. Bian, Z. Xiao, Y. Zhang and Y. Yuan, Anal. Chim. Acta, 2019, 1091, 119–126 CrossRef CAS PubMed.
- M. Halawa, J. Lai and G. Xu, Mater. Today Nano, 2018, 3, 9–27 CrossRef.
- Y. Ishii, M. Iijima, T. Umemura, A. Nishikawa, Y. Iwasaki, R. Ito, K. Saito, M. Hirose and H. Nakazawa, J. Pharm. Biomed. Anal., 2006, 41, 1325–1331 CrossRef CAS PubMed.
- L. Jiang, S. Gu, Y. Ding, D. Ye, Z. Zhang and F. Zhang, Colloids Surf., B, 2013, 107, 146–151 CrossRef CAS PubMed.
- P. Kanchana, N. Lavanya and C. Sekar, Mater. Sci. Eng., C, 2014, 35, 85–91 CrossRef CAS PubMed.
- Y. Wang, C. Xiong, H. Qu, W. Chen, A. Ma and L. Zheng, J. Electroanal. Chem., 2017, 799, 321–326 CrossRef CAS.
- G. Vyas, S. Bhatt, M. K. Si, S. Jindani, E. Suresh, B. Ganguly and P. Paul, Spectrochim. Acta, Part A, 2020, 230, 118052 CrossRef CAS PubMed.
- S. Li, M. Xing, H. Wang, L. Zhang, Y. Zhong and L. Chen, RSC Adv., 2015, 5, 59286–59291 RSC.
- T. Hasegawa, Int. J. Mol. Sci., 2010, 11, 1082–1089 CrossRef CAS PubMed.
- A. S. Saini, J. Kumar and J. S. Melo, Anal. Chim. Acta, 2014, 849, 50–56 CrossRef CAS PubMed.
- P. Agarwal, M. Singh, J. Singh and R. Singh, Applied Microbiology and Bioengineering, 2019, pp. 3–19 Search PubMed.
- Y. Zhang, S. Tsitkov and H. Hess, Nat. Commun., 2016, 7, 13982 CrossRef CAS PubMed.
- J. Zhao, S. Wang, S. Lu, X. Bao, J. Sun and X. Yang, Anal. Chem., 2018, 90, 7754–7760 CrossRef CAS PubMed.
- N. Sasaki, Y. Abe, Y. Goda, T. Adachi, K. Kasahara and Y. Ozeki, Plant Cell Physiol., 2009, 50, 1012–1016 CrossRef CAS PubMed.
- F. Gandía-Herrero and F. García-Carmona, Trends Plant Sci., 2013, 18, 334–343 CrossRef PubMed.
- T. Nakatsuka, E. Yamada, H. Takahashi, T. Imamura, M. Suzuki, Y. Ozeki, I. Tsujimura, M. Saito, Y. Sakamoto and N. Sasaki, Sci. Rep., 2013, 3, 1970 CrossRef PubMed.
- P.-H. Chen, C. Lin, K.-H. Guo and Y.-C. Yeh, RSC Adv., 2017, 7, 29302–29305 RSC.
- Y.-K. Lin and Y.-C. Yeh, Anal. Chem., 2017, 89, 11178–11182 CrossRef CAS PubMed.
- Y.-C. Chou, C.-I. Shih, C.-C. Chiang, C.-H. Hsu and Y.-C. Yeh, Sens. Actuators, B, 2019, 126717 CrossRef CAS.
- F. Gandía-Herrero, J. Escribano and F. García-Carmona, Planta, 2010, 232, 449–460 CrossRef PubMed.
- P. S. Grewal, C. Modavi, Z. N. Russ, N. C. Harris and J. E. Dueber, Metab. Eng., 2018, 45, 180–188 CrossRef CAS PubMed.
- K. Nakamura, S. Matsumoto, H. Mitsubuchi and F. Endo, Pediatr. Int., 2015, 57, 37–40 CrossRef CAS PubMed.
- G. Liu, J. Zhao, S. Lu, S. Wang, J. Sun and X. Yang, ACS Sens., 2018, 3, 1855–1862 CrossRef CAS PubMed.
- T.-I. Kim, J. Park, S. Park, Y. Choi and Y. Kim, Chem. Commun., 2011, 47, 12640–12642 RSC.
Footnote |
† Electronic supplementary information (ESI) available. See DOI: 10.1039/d0ra05581f |
|
This journal is © The Royal Society of Chemistry 2020 |
Click here to see how this site uses Cookies. View our privacy policy here.