DOI:
10.1039/D0RA05739H
(Paper)
RSC Adv., 2020,
10, 42340-42348
A fungus-derived biomass porous carbon–MnO2 nanocomposite-modified electrode for the voltammetric determination of rutin
Received
1st July 2020
, Accepted 3rd October 2020
First published on 20th November 2020
Abstract
In this study, we designed a simple procedure for the synthesis of fungus-derived biomass porous carbon (FBPC), which was further used to prepare a MnO2@FBPC composite by a hydrothermal method. The MnO2@FBPC nanocomposite showed a porous structure, large specific surface area, and high conductivity, and was modified on the carbon ionic liquid electrode (CILE) to obtain a working electrode for the sensitive voltammetric determination of rutin. The electrochemical response of rutin was studied via cyclic voltammetry with electrochemical parameters calculated. Under the optimal conditions, the linear range for the rutin analysis was obtained by the differential pulse voltammetry from 0.008 to 700.0 μmol L−1 with the detection limit of 2.67 nmol L−1 (3σ). This MnO2@FBPC/CILE was applied to directly detect the rutin concentration in drug and human urine samples with satisfactory results.
1 Introduction
Rutin is one of the most important flavonoid glycoside compounds that is widely present in legumes, vegetables, fruits, nuts, and seeds of plants.1 Due to the physiological activities such as anti-inflammatory, anti-bacterial, and anti-tumor,2–4 rutin has been used in pharmaceutical applications. Therefore, it is necessary to develop sensitive methods to detect the rutin content in various samples. Numerous analytical techniques have been established for the analysis of rutin, such as capillary electrophoresis,5 adsorptive stripping voltammetry,6 chemiluminescence,7 high-performance liquid chromatography,8 sequential injection analysis,9 and spectrophotometry.10 However, some of these techniques exhibit shortcomings including time-consuming, high cost, and complicated operation processes. Among these methods, electrochemical procedures have been applied to rutin detection due to its high sensitivity, convenient and fast procedure, cheap instrumentation, and on-site monitoring.11 For example, He et al. fabricated an electrochemical sensor for the rutin detection based on amine-functionalized Fe3O4 nanoparticles and electrochemically reduced graphene oxide nanocomposite-modified glassy carbon electrode.12 Apetrei et al. presented a graphene–gold nanoparticle-based screen-printed voltammetric sensor for the determination and quantification of rutin in pharmaceutical samples.13 Xing et al. presented a gold nanoparticle-loaded ZnS nanocomposite-based voltammetric sensor for the sensitive determination of rutin.14 Niu et al. constructed a graphene and gold nanoparticle-modified acupuncture needle electrode to detect the concentration of rutin.15 Sun et al. synthesized a graphene–MnO2 nanocomposite-modified electrode for the sensitive determination of rutin.16 Among the modified electrodes reported for rutin detection, numerous types of nanocomposites have been synthesised and used as modifiers that can adsorb more rutin on the electrode surface, exhibit fast electron transfer rate for rutin electrochemistry, and show certain electrocatalytic activity towards rutin. However, the modifiers have disadvantages such as expensive raw materials or complicated synthesis procedures.
Biomass porous carbon (BPC) is defined as a carbon-rich, porous solid produced by the thermal decomposition of biomass in a reactor with little or no available air at moderate temperatures.17 It has received considerable attention in the fields of environment, energy, and sensing due to its large active surface areas, pore volumes, cheap price, high chemical, and thermal stability.18,19 Recently, numerous synthetic methods have been proposed to prepare porous carbon by the pyrolysis of biomass in the presence of chemical agents such as KOH, ZnCl2, and H3PO4.20 For example, Sevilla et al. prepared nitrogen-doped porous carbon with a surface area of up to 3480 m2 g−1 by the KOH activation of polypyrrole as a carbon source.21 Chang et al. synthesised biomass-activated carbon via the ZnCl2 chemical activation of a recycled filter paper with specific capacitance as high as 302.3 F g−1.22 Dai et al. prepared argy wormwood-based porous carbon electrode materials through H3PO4 pre-treatment, hydrothermal carbonization, and subsequent KOH activation for high-performance supercapacitors.23
The electrode material is a major factor that affects the performance of electrochemical sensors. Numerous types of metal oxides have been used as electrode materials.24–27 As an attractive inorganic semiconductive material with electrocatalytic ability, MnO2 has aroused widespread concern in sensing electrodes owing to its low toxicity, inexpensive cost, wide potential range, natural abundance, and high specific capacitance.28,29 Also, MnO2 can be combined with other conductive materials to get composites with improved conductivity.30 Jafta et al. prepared an asymmetric electrochemical device with an MnO2/graphene oxide composite by a hydrothermal reaction.31 Yuan et al. prepared an excellent electrochemical supercapacitor with MnO2 and rice husk-based carbon composite as the active material.32
In this study, fungus was used as the raw material to prepare fungus-derived biomass porous carbon (FBPC) by alkali-treatment activation and carbonization. Then, the hydrothermal method was used to get an MnO2 decorated FBPC composite MnO2@FBPC, which was further applied on the top of a carbon ionic liquid electrode (CILE) to obtain the modified electrode. CILE is prepared by using a highly conductive ionic liquid (IL) as the modifier, which has been used as the basic electrode in electroanalysis due to its specific characteristics such as faster electron transfer, higher stability, and wider potential window. Our group fabricated numerous types of modified electrodes using CILE for electrochemical sensors and electrocatalysis.33–37 Therefore, CILE is used as the substrate electrode with the MnO2@FBPC nanocomposite as the modifier. The electrochemical responses of rutin on MnO2@FBPC/CILE were investigated via cyclic voltammetry (CV) and differential pulse voltammetry (DPV) with electrochemical parameters calculated. The modified electrode was further applied for the analysis of samples with satisfactory results.
2 Materials and methods
2.1 Reagents
1-Hexylpyridinium hexafluorophosphate (HPPF6 > 99%, Lanzhou Yulu Fine Chemical Co., Ltd., China), graphite powder (average particle size 30 μm, Shanghai Colloid Chemical Co., China), KMnO4 (Tianjin Zhiyuan Chemical Reagent Co., Ltd., China), MnSO4·H2O (Guangdong Xiqiao Chemical Co., Ltd., China), rutin (Sinopharm Chemical Reagent Co., Ltd., China) and rutin tablet sample (Shanxi Yunpeng Pharmaceutical Co., Ltd., China, and Shanghai Zhaohui Pharmaceutical Co., Ltd., China) were used as received. Fungus was purchased from a local agricultural market of Haikou. A 0.1 mol L−1 phosphate buffer solution (PBS) with different pH values was used as a supporting electrolyte. All the other chemicals were of analytical reagent grade without further purification, and ultrapure water (Milli-Q, IQ-7000, USA) was used in all the experiments.
2.2 Instruments
The morphologies of the as-prepared materials were examined using a JSM-7100F scanning electron microscope (JEOL, Japan). X-ray diffraction (XRD) measurements were performed on a D2 phaser advance diffractometer (Bruker, Germany) with Cu Kα (λ = 1.5406 nm) radiation. X-ray photoelectron spectroscopy (XPS) and the surface elemental compositions were performed on an AXIS HIS 165 spectrometer (Kratos Analytical, UK). Raman spectrum was obtained on a LabRAM HR system (Horiba, France) at an excitation wavelength of 532 nm. Electrochemical measurements were carried out on a CHI 660E electrochemical workstation (Shanghai CH Instruments, China) with a traditional three-electrode system, including MnO2@FBPC/CILE as the working electrode, Ag/AgCl (saturated KCl) as the reference electrode, and a platinum wire electrode as the counter electrode. An Agilent 1200 (Agilent Technologies, USA) was used for HPLC with chromatographic separation performed on the Agilent Diamonsil C18 column (250 mm × 4.6 mm, 5 μm, USA). HPLC mobile phase consisted of a 0.4% phosphoric acid–methanol (48
:
52, v/v) mixture, which was filtered through a 0.45 μm filter, followed by degassing under vacuum and passed at a flow rate of 0.5 mL min−1 at an injection volume of 10 μL. The measurement process was carried out in a chromatographic column at 30 °C with the detector wavelength set at 368 nm.
2.3 Synthesis of FBPC
The fungus was washed with water and dried at 100 °C for 12 h. Then, 2.0 g fungus was mixed with 50 mL of 0.1 mol L−1 KOH, transferred into a Teflon lined stainless-steel autoclave (100 mL), and heated at 120 °C for 6 h. After the product was cooled to room temperature naturally and dried at 100 °C for 24 h, the sample was heated in a KMTF-100 tube furnace (Anhui Kemi Instruments, China) under an Ar flow at 800 °C (10 °C min−1) for 1 h. Finally, the sample was washed three times with water and 1.0 mol L−1 HCl, respectively, until the pH of the solution reached 7.0. Then, the product was dried at 100 °C in an oven for 12 h to obtain FBPC.
2.4 Synthesis of MnO2@FBPC
The MnO2@FBPC composite was synthesized by a hydrothermal method.38 Briefly, 0.17 g KMnO4, 0.28 g MnSO4, and 0.24 g FBPC were dispersed in 10 mL water to form a homogeneous solution under constant magnetic stirring. Then, the mixture was transferred into a Teflon lined stainless-steel autoclave and heated at 140 °C for 12 h. The sample was filtered and washed three times with water and dried at 60 °C for 12 h to obtain MnO2@FBPC.
2.5 Preparation of the modified electrode
According to the previous literature,39 CILE was prepared with HPPF6 and graphite powder, which was smoothed to a mirror-like surface before use. 2.0 mg mL−1 of the MnO2@FBPC suspension was sonicated for 4 h to obtain a uniform dispersion. Then, 8.0 μL of the MnO2@FBPC suspension was cast on the CILE surface and dried at room temperature in air to construct MnO2@FBPC/CILE.
3 Results and discussion
3.1 Principles
Scheme 1 illustrates the fabrication process of the MnO2@FBPC composite, which was modified on the surface of CILE and used for the rutin analysis. The fungus is activated in 0.1 mol L−1 KOH and then carbonized at 800 °C to form a loose and porous structure. Subsequently, MnO2 nanoneedles are formed on FBPC by the hydrothermal method. The as-prepared materials are used for electrode modification, and the electrochemical behaviour of rutin is further investigated on the MnO2@FBPC modified electrode.
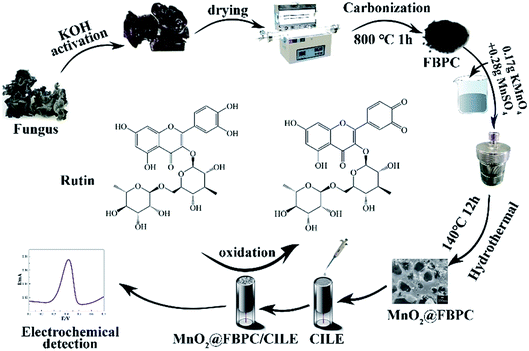 |
| Scheme 1 Fabrication process of MnO2@FBPC composites and the electrochemical detection of rutin. | |
3.2 Characterization of MnO2@FBPC
SEM images of FBPC and MnO2@FBPC were recorded and are exhibited in Fig. 1. As for FBPC (Fig. 1A), a tubular channel and three-dimensional porous structure could be observed, which was attributed to activation by KOH and further high-temperature carbonization. This porous structure can provide a good interface for the further attachment or growth of other nanomaterials on and inside its structure. As for MnO2@FBPC, MnO2 nanoneedles could be observed on the FBPC surface (Fig. 1B and C). The possible formation mechanism of MnO2 can be described with the following chemical equation:
3MnSO4 + 2KMnO4 + 2H2O → 5MnO2 + 2H2SO4 + K2SO4. |
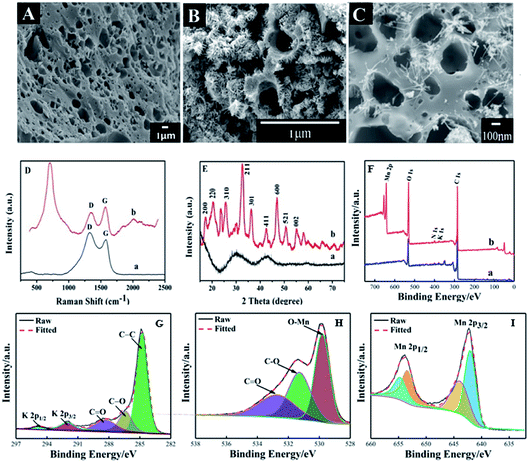 |
| Fig. 1 SEM images of (A) FBPC and (B and C) MnO2@FBPC at various magnification, (D) Raman spectra, (E) XRD survey and (F) XPS patterns of FBPC (a) and MnO2@FBPC (b); XPS spectra of the MnO2@FBPC (G) C 1s, (H) O 1s, (I) Mn 2p. | |
Raman spectroscopy is an effective approach to study the structure of carbon materials and the curves are shown in Fig. 1D. The characteristic peaks of the Raman spectrum are located at 1603 cm−1 and 1337 cm−1 (curve a and b), which are attributed to the G and D bands of the carbon material, respectively. Because the intensity ratio of ID/IG represents the disorder/defect degree of the carbon material, the ID/IG values of FBPC and MnO2@FBPC were obtained as 1.2 and 0.7, respectively. Obviously, the ID/IG value decreased in the presence of MnO2 nanoneedles, indicating a relatively high degree of graphitization. Compared with that of FBPC, a new peak could be found at 631 cm−1 (curve b), which belonged to the characteristic peak of MnO2.
XRD patterns were recorded to determine the crystal structure of FBPC and MnO2@FBPC, and the curves are shown in Fig. 1E. In the XRD pattern of FBPC (curve a), two typical diffraction peaks at 2θ values of 29.1° and 42.4° could be ascribed to reflections from the (002) and (110) crystal planes of the carbon material. For the MnO2@FBPC nanocomposite, strong diffraction peaks appeared at 18.0°, 22.4°, 28.7°, 37.6°, 41.9°, 49.8°, 56.0°, 60.1°, and 65.5°, which were attributed to the (200), (220), (310), (211), (301), (411), (600), (521), and (002) crystal faces of MnO2. Therefore, the MnO2 crystal face is retained in MnO2@FBPC.
XPS was performed to obtain the elemental composition of FBPC and MnO2@FBPC, and the resulting curves are shown in Fig. 1F. The C 1s spectra were fitted with five component peaks, as shown in Fig. 1G. The peaks located at 284.78 eV, 286.38 eV, 288.38 eV, 292.02 eV and 294.78 eV are attributed to the structure of graphite carbon (C–C), carbon–oxygen (C–O), carboxyl group (C
O), K 2p2/3 and K 2p1/2, respectively. The characteristic peaks (529.80 eV, 531.28 eV, and 532.72 eV) of O 1s indicated that the structures of Mn–O, C–O, and C
O, respectively, as shown in Fig. 1H existed in the composite. Mn 2p was combined with the fitted peaks (642.00 eV and 643.90 eV) of Mn 2p2/3 and the fitted peaks (653.40 eV and 654.70 eV) of Mn 2p1/2. Therefore, it was concluded that MnO2 was synthesized and coated on FBPC. Moreover, the atomic ratio of Mn in the MnO2@FBPC composite was calculated as 9.95%.
3.3 Electrochemical investigations
Cyclic voltammetric responses of different electrodes were recorded using a 1.0 mmol L−1 [Fe (CN)6]3− solution as an electrochemical probe, and the results are shown in Fig. 2A. A pair of symmetric redox peaks could be observed on bare CILE (curve a), and the redox peaks increased gradually on FBPC/CILE (curve b) and MnO2@FBPC/CILE (curve c), indicating that the highly conductive FBPC and MnO2@FBPC composite were beneficial for the electron transfer and further effectively improved the interfacial conductivity. The linear relationships between Ip and the square-root of the scan rate (υ1/2) was obtained with the equations as Ipc (μA) = 46.408υ1/2 − 14.348 (n = 17, γ = 0.992) and Ipa (μA) = −40.043υ1/2 + 11.628 (n = 17, γ = 0.990). According to the Randles–Sevcik formula, Ipa (A) = (2.69 × 105)n3/2AD1/2C0υ1/2,40 the effective surface area (A) of MnO2@FBPC/CILE was calculated to be 0.241 cm2, which was 1.67 times larger than that of CILE (0.144 cm2), indicating that the presence of the MnO2@FBPC composite on the electrode increased the effective area and provided more active sites on the electrochemical surface with improved electrode performance.
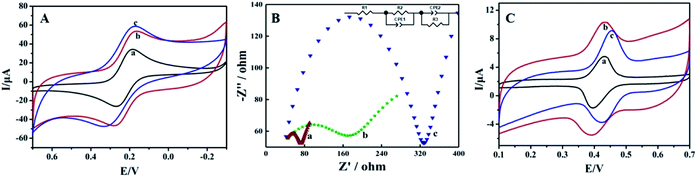 |
| Fig. 2 (A) Cyclic voltammograms of CILE (a), FBPC/CILE (b) and MnO2@FBPC/CILE (c) in 1.0 mmol L−1 [Fe(CN)6]3− and 0.5 mol L−1 KCl solution at a scan rate of 100 mV s−1. (B) EIS of MnO2@FBPC/CILE (a), FBPC/CILE (b) and CILE (c) in 10.0 mmol L−1 [Fe(CN)6]3− and 0.5 mol L−1 KCl solution with frequency ranging from 104 to 0.1 Hz. (C) Cyclic voltammograms of 1.0 × 10−5 mol L−1 rutin in PBS (pH 4.0) on CILE (a), FBPC/CILE (b) and MnO2@FBPC/CILE (c) at a scan rate of 100 mV s−1. | |
To get the impedance information, electrochemical impedance spectroscopy (EIS) was performed in a 10 mmol L−1 [Fe(CN)6]3− and 0.5 mol L−1 KCl solution with frequency ranging from 104 to 0.1 Hz. The experimental data were fitted by the R(CR)(CR) model in Fig. 2B. The Ret of MnO2@FBPC/CILE (35.0 Ω, curve a) was smallest among those of three electrodes, and the Ret of FBPC/CILE (182.3 Ω, curve b) was lower than that of CILE (302.4 Ω, curve c), indicating an improved electron transfer, which was attributed to the presence of porous FBPC and MnO2.
The electrochemical behaviour of 1.0 × 10−5 mol L−1 rutin on different electrodes was studied via CV in PBS (0.1 mol L−1, pH 4.0), and the curves are shown in Fig. 2C. A couple of well-defined redox peaks could be observed, which was the typical electrochemical reaction of rutin. On CILE, the peak currents were obtained as 3.206 μA (Ipa) and 3.268 μA (Ipc) with the peak-to-peak separation (ΔEp) of 35 mV (curve a). On FBPC/CILE, the redox peak currents of rutin were increased to 4.100 μA (Ipa) and 3.474 μA (Ipc) with ΔEp as 39 mV (curve b), proving the positive effect of FBPC to the rutin electrochemistry. Also, the increase in the background currents was due to the porous structure and large surface area of FBPC, indicating that the presence of the porous structure increased the interfacial capacitance. Moreover, on MnO2@FBPC/CILE (curve c), the electrochemical responses were further increased with the decrease of ΔEp to 31 mV, indicating that the redox reaction of rutin become more reversible. The corresponding redox peak currents were 5.338 μA (Ipa) and 3.997 μA (Ipc), and the currents were enlarged 1.7 times for Ipa and 1.2 times for Ipc, as compared to that of CILE. The modification of MnO2 nanoneedles on the surface of FBPC significantly increased the electrocatalytic activity towards the oxidation of rutin with the enhancement of the electrochemical responses. Therefore, the presence of the high conductivity of MnO2@FBPC promoted the electron transfer ability towards rutin.
3.4 Effect of pH and scan rate
The effect of buffer pH on the electrochemical responses of 1.0 × 10−5 mol L−1 rutin on MnO2@FBPC/CILE was investigated, and the results are shown in Fig. 3A. With the change in pH from 2.0 to 8.0, the redox peak potential shifted towards the negative direction and the peak current achieved a maximum value at pH 4.0, which was selected as the supporting electrolyte. The formal peak potential (E0′) had a good linear relationship with the pH value and the regression equation was E0′ (V) = −0.054 pH + 0.65 (n = 7, γ = 0.998). The slope of 54 mV pH−1 was close to 59 mV pH−1, which suggested that the number of electrons transferred was equal to the protons in the electrode reaction process.
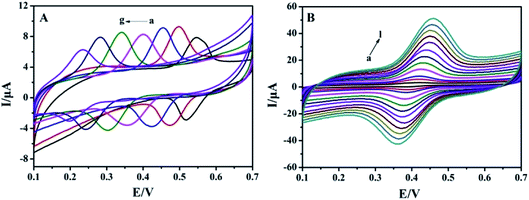 |
| Fig. 3 Cyclic voltammograms of 1.0 × 10−5 mol L−1 rutin on MnO2@FBPC/CILE with (A) different pH PBS (a–g: 2.0, 3.0, 4.0, 5.0, 6.0, 7.0, 8.0) at the scan rate of 100 mV s; (B) different scan rates in pH 4.0 PBS (a–l: as 20, 60, 100, 200, 300, 400, 500, 600, 700, 800, 900, 1000 mV s−1). | |
The effect of the scan rate on the redox response of 1.0 × 10−5 mol L−1 rutin was recorded, and the results are shown in Fig. 3B. The redox peak currents increased gradually with the increase in the scan rate in the range from 20 mV s−1 to 1000 mV s−1, and the linear relationship of peak currents and scan rate were Ipa (μA) = 32.64υ (V s−1) + 1.13 (n = 13, γ = 0.997) and Ipc (μA) = −28.81υ (V s−1) − 1.11 (n = 13, γ = 0.998), which indicated that the electrode reaction was an adsorption-controlled process due to the porous structure of FBPC. The redox peak potential and ln
υ also exhibited good linear relationships with the linear regression equations, as Epa (V) = 0.023
ln
υ + 0.458 (n = 8, γ = 0.990) and Epc (V) = −0.025
ln
υ + 0.358 (n = 8, γ = 0.994). According to the Laviron's equation,41 the values of the electron transfer coefficient (α), electron transfer number (n), and electrode reaction standard rate constant (ks) were calculated as 0.48, 2.14, and 4.65 s−1, respectively.
3.5 Effects of accumulation conditions
To improve the detection sensitivity, the effects of accumulation time and potential on the oxidation peak current were investigated, as shown in Fig. 4. Ipa increased rapidly with the accumulation time from 0 s to 40 s and then decreased by more than 40 s, indicating the saturated adsorption of rutin (Fig. 4A). Simultaneously, Ipa increased with the increase in the accumulation potential from 0 V to 0.4 V and gradually decreased when the accumulation potential was more than 0.4 V (Fig. 4B). Therefore, 40 s and 0.4 V were chosen as the optimal accumulation conditions in the subsequent experiments.
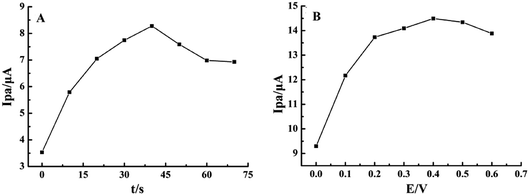 |
| Fig. 4 Effects of (A) accumulation time and (B) potential on Ipa of 1.0 × 10−5 mol L−1 rutin on MnO2@FBPC/CILE. | |
3.6 Calibration curve
Differential pulse voltammetric (DPV) curves of different rutin concentrations on MnO2@FBPC/CILE are shown in Fig. 5A. The oxidation peak currents increased with rutin in two concentration ranges, i.e., from 0.008 to 100.0 μmol L−1 and 100.0 to 700.0 μmol L−1. The linear regression equations were calculated as Ipa (μA) = 0.643C (μmol L−1) + 4.733 (n = 14, γ = 0.996) and Ipa (μA) = 0.047C (μmol L−1) + 61.095 (n = 4, γ = 0.992) with the detection limit calculated as 2.67 nmol L−1 (3σ). Two different slopes of the calibration curves could be attributed to the difference in the activity of the modified electrode surface at low and high rutin concentrations. At the low concentration range, a large slope was due to the relatively high ratio of active sites to the total number of rutin molecules. At the high concentration range, the active sites decreased in comparison to the total number of rutin molecules at the electrode surface, leading to a smaller slope value. The analytical parameters for the electrochemical detection of rutin on different modified electrodes are summarized in Table 1. The low detection limit was due to the presence of MnO2 on the surface of FBPC with a porous structure, larger surface area, high conductivity, and excellent electrocatalytic activity.
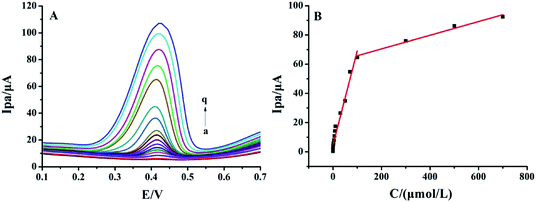 |
| Fig. 5 DPV curves of rutin with various concentrations (A) a–q: 0.008, 0.01, 0.1, 0.6, 1.0, 2.0, 4.0, 6.0, 8.0, 10.0, 30.0, 50.0, 70.0, 100.0, 300.0, 500.0, 700.0 μmol L−1. (B) The linear relationship between Ipa and rutin concentration. | |
Table 1 Comparison of various modified electrodes for rutin detection
Electrodes |
Linear range (μmol L−1) |
LOD (μmol L−1) |
Ref. |
Poly(p-aminobenzene sulfonic acid). Glassy carbon electrode. Graphene. Nickel nanoparticle–graphene oxide. Cuprous-oxide–Au nanoparticles. N-doped graphene. Au–Ag nanoparticles. Palladium phthalocyanine–multiwalled carbon nanotube. Multi-wall carbon nanotubes. β-Cyclodextrin. |
PABSAa/GCEb |
0.25–10.0 |
0.0012 |
42 |
GRc–MnO2/CILE |
0.01–500.0 |
0.0027 |
43 |
Ni–GOd/GCE |
0.011–15.0 |
0.0032 |
44 |
Cu2O–Aue/NGf/GCE |
0.06–512.9 |
0.03 |
45 |
Au–AgNTg/NG |
0.79–61.0 |
0.16 |
46 |
PdPc–MWCNTh–Nafion/GCE |
0.1–51.0 |
75.0 |
47 |
MWNTsi/β-CDj/GCE |
0.4–1000.0 |
0.2 |
48 |
MnO2@FBPC/CILE |
0.008–700.0 |
0.00267 |
This work |
3.7 Analytical applications
In order to verify the practicality and reliability of the proposed sensor, MnO2@FBPC/CILE was applied for the determination of rutin content in compound rutin tablets (20 mg per tablet) and human urine by the calibration curve method and standard addition method. Drug tablets were purchased from Shanxi Yunpeng Pharmaceutical Ltd. Co. (B080302) and Shanghai Zhaohui Pharmaceutical Ltd. Co. (090904). A piece of drug tablet was ground and dissolved in 5.0 mL ethanol to obtain a 6.02 mmol L−1 stock solution. Then, a 100 μL stock solution was diluted with pH 4.0 PBS in a 10.0 mL calibration tube for further detection. Drug samples were also detected by the HPLC method based on the ref. 49 with the average value as 62.29 μmol L−1, which was close to the electrochemical data (shown in Table 2). The human urine sample (from health volunteer) was collected and the supernatant from the static solution was removed. Then, 0.1 mL sample was further diluted to 10.0 mL with pH 4.0 PBS, detected by the proposed procedure with the addition of a standard rutin solution to test the recovery, which was in the range of 92.45–105.03%. All the above results confirmed that MnO2@FBPC/CILE could be efficiently applied for rutin detection with good accuracy.
Table 2 Detection of rutin in different samples with MnO2@FBPC/CILE (n = 3)
Sample |
Labeled (μmol L−1) |
Found (μmol L−1) |
Added (μmol L−1) |
Measured (μmol L−1) |
RSD (%) |
Recovery (%) |
B080302 |
60.20 |
59.18 |
20.00 |
80.67 |
2.18 |
107.46 |
090904 |
60.20 |
60.03 |
60.00 |
121.96 |
3.23 |
103.22 |
Normal human urine |
— |
0.00 |
20.00 |
18.49 |
4.79 |
92.45 |
40.00 |
42.01 |
5.95 |
105.03 |
60.00 |
59.05 |
1.69 |
98.42 |
3.8 Interferences study
The influence in a number of inorganic ions, amino acids, glucose, and some flavonoid drugs on the detection of 1.0 × 10−5 mol L−1 rutin was also investigated. As shown in Table 3, it can be seen that higher concentrations (by 50 times) of inorganic ions, amino acids, glucose, uric acid, and dopamine led to the relative error (RE) less than 7.50%. Similar concentrations of bisphenol A, ascorbic acid, catechol, hydroquinone, baicalin, and luteolin had a relative error of less than 8.0%. Therefore, this MnO2@FBPC/CILE may be used for the analysis of the rutin content in drug or biological samples.
Table 3 Influence of co-existing substances on 1.0 × 10−5 mol L−1 rutin analysis
Co-exiting species |
Concentration (mol L−1) |
RE (%) |
Co-exiting species |
Concentration (mol L−1) |
RE (%) |
KCl |
5.0 × 10−4 |
5.18 |
BaCl2 |
5.0 × 10−4 |
2.82 |
NaCl |
5.0 × 10−4 |
2.87 |
CoCl2 |
5.0 × 10−4 |
7.16 |
CaCl2 |
5.0 × 10−4 |
2.16 |
Bisphenol A |
1.0 × 10−5 |
6.65 |
Glucose |
5.0 × 10−4 |
2.87 |
Ascorbic acid |
1.0 × 10−5 |
1.63 |
Alanine |
5.0 × 10−4 |
−0.62 |
Catechol |
1.0 × 10−5 |
2.86 |
Glutamine |
5.0 × 10−4 |
2.99 |
Hydroquinone |
1.0 × 10−5 |
8.03 |
Uric acid |
5.0 × 10−4 |
3.36 |
Baicalin |
1.0 × 10−5 |
6.73 |
Dopamine |
5.0 × 10−4 |
−3.74 |
Luteolin |
1.0 × 10−5 |
3.07 |
3.9 Reproducibility, repeatability, and stability
The reproducibility of MnO2@FBPC/CILE was performed by detecting the 1.0 × 10−5 mol L−1 rutin solution. The RSD value of six parallel measurements with a single electrode was calculated as 6.98%, indicating the good reproducibility of this method. In a series of five electrodes prepared in the same way, an RSD of 2.40% was obtained for the 1.0 × 10−5 mol L−1 rutin solution, indicating excellent repeatability. The long-term stability of MnO2@FBPC/CILE was tested after being stored at room temperature for a week. The current responses were retained at 90% of its original signal strengths for the same rutin solution, which showed long-term stability.
4 Conclusions
The fungus-based biomass porous carbon (FBPC) was prepared by activation and high temperature carbonization, and the MnO2@FBPC composite was directly synthesised by a hydrothermal method. The morphology, structure, and composition of the materials were characterized by SEM, Raman, XRD, and XPS. The results showed that the MnO2@FBPC composite had a porous structure, large surface area, good conductivity, and high electrocatalytic activity. The electrochemical response of rutin on MnO2@FBPC/CILE was investigated with the electrochemical parameters calculated, which exhibited a wider linear range (0.008–700.0 μmol L−1) and lower detection limit for the rutin detection, and the method was successfully applied to the real sample detection. This work extended the usage of biomass porous carbon and the related composite in the field of electroanalysis.
Conflicts of interest
There are no conflicts to declare.
Acknowledgements
This work was financially supported by the Hainan Provincial Natural Science Foundation of China (2018CXTD336), the National Natural Science Foundation of China (61864002), the Open Foundation of Key Laboratory of Laser Technology and Optoelectronic Functional Materials of Hainan Province (2020LTOM01), and Open Project of Chemistry Department of Qingdao University of Science and Technology (QUSTHX201935).
References
- J. B. He, Y. Wang, N. Deng, Z. G. Zha and X. Q. Lin, Cyclic voltammograms obtained from the optical signals: study of the successive electro-oxidations of rutin, Electrochim. Acta, 2007, 52, 6665–6672 CrossRef CAS.
- R. M. Gené, C. Cartaña, T. Adzet, E. Marín, T. Parella and S. Cañigueral, Anti-inflammatory and analgesic activity of baccharis trimera: identification of its active constituents, Planta Med., 1996, 62, 232–235 CrossRef.
- R. Ramanathan, N. Das and C. Tan, Inhibitory effects of 2-hydroxy chalcone and other flavonoids on human cancer cell-proliferation, Int. J. Oncol., 1993, 3, 115 CAS.
- S. Yang, Q. U. Ling, L. I. Gang, Y. Ran and C. Liu, Gold nanoparticles/ethylenediamine/carbon nanotube modified glassy carbon electrode as the voltammetric sensor for selective determination of rutin in the presence of ascorbic acid, J. Electroanal. Chem., 2010, 645, 115–122 CrossRef CAS.
- G. Chen, J. Zhang and J. H. Ye, Determination of puerarin, daidzein and rutin in Pueraria lobata (Wild.) Ohwi by capillary electrophoresis with electrochemical detection, J. Chromatogr. A, 2001, 923, 255–262 CrossRef CAS.
- G. J. Volikakis and C. E. Efstathiou, Determination of rutin and other flavonoids by flow-injection/adsorptive stripping voltammetry using nujol-graphite and diphenylether-graphite paste electrodes, Talanta, 2000, 51, 775–785 CrossRef CAS.
- S. Hartwell, Sensitive determination of sub-nanogram amounts of rutin by its inhibition on chemiluminescence with immobilized reagents, Talanta, 2002, 57, 59–67 CrossRef.
- K. Ishii, T. Furuta and Y. Kasuya, High-performance liquid chromatographic determination of quercetin in human plasma and urine utilizing solid-phase extraction and ultraviolet detection, J. Chromatogr. B: Anal. Technol. Biomed. Life Sci., 2003, 794, 49–56 CrossRef CAS.
- Z. Legnerová, Using on-line solid phase extraction for simultaneous determination of ascorbic acid and rutin trihydrate by sequential injection analysis, Anal. Chim. Acta, 2003, 497, 165–174 CrossRef.
- H. N. Hassan, B. N. Barsoum and I. H. Habib, Simultaneous spectrophotometric determination of rutin, quercetin and ascorbic acid in drugs using a kalman filter approach, J. Pharm. Biomed. Anal., 1999, 20, 315–320 CrossRef CAS.
- S. Hu, H. Zhu, S. Liu, J. Xiang, W. Sun and L. Zhang, Electrochemical detection of rutin with a carbon ionic liquid electrode modified by Nafion, graphene oxide and ionic liquid composite, Microchim. Acta, 2012, 178, 211–219 CrossRef CAS.
- Q. He, J. Liu, X. Liu, G. Li, D. Chen, P. Deng and L. Jing, Fabrication of amine-modified magnetite-electrochemically reduced graphene oxide nanocomposite modified glassy carbon electrode for sensitive dopamine determination, Nanomaterials, 2018, 8, 194 CrossRef.
- I. M. Apetrei and C. Apetrei, A modified nanostructured graphene-gold nanoparticle carbon screen-printed electrode for the sensitive voltammetric detection of rutin, Measurement, 2018, 114, 37–43 CrossRef.
- R. Xing, X. Zhao, Y. Liu, J. Liu, B. Liu, Y. Ren, S. Liu and L. Mao, Low cost and reliable electrochemical sensor for rutin detection based on Au nanoparticles-loaded ZnS nanocomposites, J. Nanosci. Nanotechnol., 2018, 18, 4651–4657 CrossRef CAS.
- X. Niu, Z. Wen, X. Li, W. Zhao, X. Li and Y. Huang, Fabrication of graphene and gold nanoparticle modified acupuncture needle electrode and its application in rutin analysis, Sens. Actuators, B, 2018, 255, 471–477 CrossRef CAS.
- S. Wei, X. Wang, H. Zhu, X. Sun, S. Fan, G. Li and Z. Sun, Graphene-MnO2 nanocomposite modified carbon ionic liquid electrode for the sensitive electrochemical detection of rutin, Sens. Actuators, B, 2013, 178, 443–449 CrossRef.
- W. J. Liu, H. Jiang and H. Q. Yu, Development of biochar-based functional materials: toward a sustainable platform carbon material, Chem. Rev., 2015, 115, 12251–12285 CrossRef CAS.
- S. Mubeen, T. Zhang, B. Yoo, M. A. Deshusses and N. V. Myung, Palladium nanoparticles decorated single-walled carbon nanotube hydrogen sensor, J. Phys. Chem. C, 2007, 111, 6321–6327 CrossRef CAS.
- J. Wang and L. Qian, Fungi-derived hierarchically porous carbons for high-performance supercapacitors, RSC Adv., 2014, 5, 4396–4403 RSC.
- W. M. Qiao, S. H. Yoon and I. Mochida, KOH activation of needle coke to develop activated carbons for high-performance EDLC, Energy Fuels, 2006, 20, 1680–1684 CrossRef CAS.
- M. Sevilla, R. Mokaya and A. B. Fuertes, Ultrahigh surface area polypyrrole-based carbons with superior performance for hydrogen storage, Energy Environ. Sci., 2011, 4, 2930 RSC.
- B. Chang, Y. Guo, Y. Li and B. Yang, Hierarchical porous carbon derived from recycled waste filter paper as high-performance supercapacitor electrodes, RSC Adv., 2015, 5, 72019–72027 RSC.
- C. Dai, J. Wan, J. Yang, S. Qu, T. Jin, F. Ma and J. Shao, H3PO4 solution hydrothermal carbonization combined with KOH activation to prepare argy wormwood-based porous carbon for high-performance supercapacitors, Appl. Surf. Sci., 2018, 444, 105–117 CrossRef CAS.
- W. Sun, Y. Guo, X. Ju, Y. Zhang, X. Wang and Z. Sun, Direct electrochemistry of hemoglobin on graphene and titanium dioxide nanorods composite modified electrode and its electrocatalysis, Biosens. Bioelectron., 2013, 42, 207–213 CrossRef CAS.
- W. Sun, X. Wang, Y. Wang, X. Ju, L. Xu, G. Li and Z. Sun, Application of graphene–SnO2 nanocomposite modified electrode for the sensitive electrochemical detection of dopamine, Electrochim. Acta, 2013, 87, 317–322 CrossRef CAS.
- X. Qi, H. Gao, Y. Zhang, X. Wang, Y. Chen and W. Sun, Electrochemical DNA biosensor with chitosan-Co3O4 nanorod-graphene composite for the sensitive detection of staphylococcus aureus nuc gene sequence, Bioelectrochemistry, 2012, 88, 42–47 CrossRef CAS.
- Y. Niu, H. Xie, G. Luo, W. Weng, C. Ruan, G. Li and W. Sun, Electrochemical performance of myoglobin based on TiO2-doped carbon nanofiber decorated electrode and its applications in biosensing, RSC Adv., 2019, 9, 4480–4487 RSC.
- Y. Zhao, W. Ran, J. He, Y. Huang, Z. Liu, W. Liu, Y. Tang, L. Zhang, D. Gao and F. Gao, High-performance asymmetric supercapacitors based on multilayer MnO2/graphene oxide nanoflakes and hierarchical porous carbon with enhanced cycling stability, Small, 2015, 11, 1310–1319 CrossRef CAS.
- L. Li, Z. Du, S. Liu, Q. Hao, Y. Wang, Q. Li and T. Wang, A novel nonenzymatic hydrogen peroxide sensor based on MnO2/graphene oxide nanocomposite, Talanta, 2010, 82, 1637–1641 CrossRef CAS.
- Y. R. Chen, K. F. Chiu, H. C. Lin, C. L. Chen and C. Y. Hsieh, Graphene/activated carbon supercapacitors with sulfonated-polyetheretherketone as solid-state electrolyte and multifunctional binder, Solid State Sci., 2014, 37, 80–85 CrossRef CAS.
- C. J. Jafta, F. Nkosi, L. L. Roux, M. K. Mathe, M. Kebede, K. Makgopa, S. Yang, D. Tong, M. Oyama and N. Manyala, Manganese oxide/graphene oxide composites for high-energy aqueous asymmetric electrochemical capacitors, Electrochim. Acta, 2013, 110, 228–233 CrossRef CAS.
- C. Yuan, H. Lin, H. Lu, E. Xing, Y. Zhang and B. Xie, Synthesis of hierarchically porous MnO2/rice husks derived carbon composite as high-performance electrode material for supercapacitors, Appl. Energy, 2016, 178, 260–268 CrossRef CAS.
- H. Xie, X. Li, G. Luo, Y. Niu and G. Li, Nano-diamond modified electrode for the investigation on direct electrochemistry and electrocatalytic behavior of myoglobin, Diamond Relat. Mater., 2019, 97, 107453 CrossRef CAS.
- J. Liu, H. Cheng, H. Xie, G. Luo and W. Sun, Platinum nanoparticles decorating a biomass porous carbon nanocomposite-modified electrode for the electrocatalytic sensing of luteolin and application, RSC Adv., 2019, 9, 33607–33616 RSC.
- H. Xie, G. Luo, Y. Niu, W. Weng and W. Sun, Synthesis and utilization of Co3O4 doped carbon nanofiber for fabrication of hemoglobin-based electrochemical sensor, Mater. Sci. Eng., C, 2020, 107, 110209 CrossRef CAS.
- R. Zou, X. Li, G. Luo, Y. Niu, W. Weng, W. Sun, J. Xi, Y. Chen and G. Li, Boron nitride nanosheet modified electrode: preparation and application to direct electrochemistry of myoglobin, Electroanalysis, 2019, 31, 575–581 CAS.
- W. Sun, Y. Wang, Y. Zhang, X. Ju and Z. Sun, Poly(methylene blue) functionalized graphene modified carbon ionic liquid electrode for the electrochemical detection of dopamine, Anal. Chim. Acta, 2012, 751, 59–65 CrossRef CAS.
- S. Xiong, X. Zhang, J. Chu, X. Wang, R. Zhang, M. Gong and B. Wu, Hydrothermal synthesis of porous sugarcane bagasse carbon/MnO2 nanocomposite for supercapacitor application, J. Electron. Mater., 2018, 47, 6575–6582 CrossRef CAS.
- W. Chen, W. Weng, X. Niu, X. Li, Y. Men, W. Sun, G. Li and L. Dong, Boron-doped graphene quantum dots modified electrode for electrochemistry and electrocatalysis of hemoglobin, J. Electroanal. Chem., 2018, 823, 137–145 CrossRef CAS.
- K. B. Oldham, Analytical expressions for the reversible Randles-Sevcik function, J. Electroanal. Chem., 1979, 105, 373–375 CrossRef CAS.
- E. Laviron, General expression of the linear potential sweep voltammogram in the case of diffusionless electrochemical systems, J. Electroanal. Chem., 1979, 101, 19–28 CrossRef CAS.
- X. Chen, Z. Wang, F. Zhang, L. Zhu, Y. Li and Y. Xia, Determination of rutin on the poly(p-aminobenzene sulfonic acid) modified glassy carbon electrode, Chem. Pharm. Bull., 2010, 58, 475–478 CrossRef CAS.
- S. Wei, X. Wang, H. Zhu, X. Sun, S. Fan, G. Li and Z. Sun, Graphene-MnO2 nanocomposite modified carbon ionic liquid electrode for the sensitive electrochemical detection of rutin, Sens. Actuators, B, 2013, 178, 443–449 CrossRef.
- Ş. U. Karabiberoğlu and Z. Dursun, Fabrication of nickel coated graphene oxide composite electrode for sensitive determination of Rutin, J. Electroanal. Chem., 2018, 76–85 CrossRef.
- S. Li, B. Yang, C. Wang, W. Jin, F. Yue, Y. Bo, Z. Xiong and Y. Du, A facile and green fabrication of Cu2O-Au/NG nanocomposites for sensitive electrochemical determination of rutin, J. Electroanal. Chem., 2017, 786, 20–27 CrossRef CAS.
- B. B. Yang, D. Bin, K. Zhang, Y. Du and T. Majima, A seed-mediated method to design N-doped graphene supported gold-silver nanothorns sensor for rutin detection, J. Colloid Interface Sci., 2018, 512, 446–454 CrossRef CAS.
- R. Xing, H. Yang, S. Li, J. Yang, X. Zhao, Q. Wang, S. Liu and X. Liu, A sensitive and reliable rutin electrochemical sensor based on palladium phthalocyanine-MWCNTs-Nafion nanocomposite, J. Solid State Electrochem., 2016, 21, 1–10 Search PubMed.
- J. L. He, Y. Yang, X. Yang, Y. L. Liu, G. L. Shen and R. Q. Yu, β-Cyclodextrin incorporated carbon nanotube-modified electrode as an electrochemical sensor for rutin, Sens. Actuators, B, 2006, 114, 94–100 CrossRef CAS.
- S. H. Nile, A. S. Nile, Y. S. Keum and K. Sharmab, Utilization of quercetin and quercetin glycosides from onion (Allium cepa L.) solid waste as an antioxidant, urease and xanthine oxidase inhibitors, Food Chem., 2017, 235, 119–126 CrossRef CAS.
|
This journal is © The Royal Society of Chemistry 2020 |
Click here to see how this site uses Cookies. View our privacy policy here.