DOI:
10.1039/D0RA06197B
(Paper)
RSC Adv., 2020,
10, 37989-37994
Thiolated poly(aspartic acid)-functionalized two-dimensional MoS2, chitosan and bismuth film as a sensor platform for cadmium ion detection†
Received
16th July 2020
, Accepted 6th October 2020
First published on 14th October 2020
Abstract
In this work, a sensitive electrochemical platform for determination of cadmium ions (Cd2+) is obtained using thiolated poly(aspartic acid) (TPA)-functionalized MoS2 as a sensor platform by differential pulse anodic stripping voltammetry (DPASV). The performance of the TPA–MoS2-modified sensor is systemically studied. It demonstrates that the TPA–MoS2 nanocomposite modified sensor exhibits superior analytical performance for Cd2+ over a linear range from 0.5 μg L−1 to 50 μg L−1, with a detection limit of 0.17 μg L−1. Chitosan is able to form a continuous coating film on the surface of the GC electrode. The good sensing performance of the TPA–MoS2-modified sensor may be attributed to the following factors: the large surface area of MoS2 (603 m2 g−1), and the abundant thiol groups of TPA. Thus, the TPA–MoS2-modified sensor proves to be a reliable and environmentally friendly tool for the effective monitoring of Cd2+ existing in aquacultural environments.
1. Introduction
Cadmium (Cd) is one of the priority pollutants due to its high toxicity.1–3 Cd2+ not only causes renal tubular dysfunction and bone degeneration (itai-itai disease),4–6 but is also classified as a carcinogenic chemical by the International Agency for Research on Cancer (IARC).7–9 Traditionally, inductively coupled plasma mass spectrometry (ICP-MS),10,11 atomic absorption spectrometry (ABS)12–14 and ion chromatography15,16 are used for detecting Cd2+. Although these instruments have good precision and high resolution, they are too expensive and labor-intensive.17,18 Therefore, it is urgent to develop a sensitive method to protect our food, medicine and drinking water from Cd2+ pollution.
The electrochemical sensor is one of the most promising tools for detecting Cd2+ due to its low cost, rapid speed, portability and reliability.19–21 Mercury modified electrode has been intensively used for detecting trace Cd2+ by differential pulse anodic stripping voltammetry (DPASV).22,23 However, the toxicity of mercury severely limits its applications in constructing sensors.24,25 Therefore, bismuth, the less toxicity heavy metal,26,27 is used for constructing sensors in this work. Except electrode materials, the sensing material also plays an important role in constructing sensors.28
As is well known, 2D materials have been established for the sensitive detection methods of environmental pollutants.29–32 Molybdenum disulfide (MoS2) is one of transition-metal dichalcogenides (TMDs), which has a typical layered structure formed by a stack of planes.33–35 Each layer of MoS2 consists of three atom layers (S–Mo–S), a layer of molybdenum atoms sandwiched between two layers of sulfur atoms.36–38 These triple layers are weakly held together by van der Waals forces.39–41 MoS2 has unique properties including good flexibility, a tunable bandgap energy controlled by the number of layers and planar electric transportation property.42–45 Due to its fascinating characteristics, MoS2 has received much attention for construction of promising electronics devices, for example, field-effect transistors, lithium-ion batteries, etc.46–49 However, exploring more binding sites on MoS2 for Cd2+ is still challenging, and this is the key to achieve excellent low detection limit and sensitivity. The poor surface modification of MoS2 limits its application as electrode material for constructing electrochemical sensors.50
To address this critical challenge, it is predicted that controllable surface modification of MoS2 could significantly improve the sensitivity of MoS2 based sensor.51,52 Thus, in this work, thiolated polymers are chemically adsorbed on electron-rich MoS2 through covalent bonding. The sulfur on the MoS2 surface plays as a soft Lewis base showing a high affinity for heavy metal ions (e.g., Bi3+, Cd2+). MoS2 demonstrates both a high adsorption capacity due to abundant sulfur adsorption sites and fast kinetics due to easy access to these sites. Therefore, thiolated polymer modified MoS2 plays as one of the most effective platforms for detecting Cd2+. It shows that this sensor possesses superior stability, sensitivity and LOD. The proposed sensor can be used as an environmental-friendly “pre-alarm” tool for rapid determining Cd2+ in aquaculture water, Chinese drug, food and beverage.
2. Experimental section
2.1. Materials and solutions
Cadmium chloride, bismuth chloride, and other chemicals are from Tianjin Kermel (Tianjin, China). Thiolated poly(aspartic acid) is obtained from Covestro company (Shanghai, China). 100 mmol L−1 pH 6.5 acetate buffer is used as the electrolyte in electrochemical experiments.
2.2. Apparatus
A JEM-2100 instrument (Japan) is used for obtaining transmission electron microscopy (TEM) images. Scan electron microscopy (SEM) images are obtained by Zeiss Sigma 300 (Germany). A CHI 660B Electrochemical Workstation (Shanghai, China) is used for testing Cd2+ through DPASV. A functionalized MoS2 and chitosan film modified glassy carbon (GC) electrode is the working electrode, the Ag/AgCl electrode is the reference electrode, and the platinum wire is the auxiliary electrode. The concentration of Cd2+ is also confirmed through atomic absorption spectrometry (AAS, Jena, Germany).
2.3. Preparation of MoS2 and modification of GC electrode
MoS2 nanosheets are prepared by an ultrasonic exfoliation method as literature reported.53 1.0 g MoS2 powder is added into 10 mL N-methyl-pyrrolidone solution under ultrasonic wave for 2 h at 0 °C. After centrifugation at 7000 rpm for 30 min, the prepared MoS2 nanosheets is dispersed in the supernatant. The 1 mL of 1 mg mL−1 prepared MoS2 is mixed with 1 mL 2 mg mL−1 thiolated poly(aspartic acid) (TPA), and vibrated by vortex for 12 h at room temperature.
The GC electrode is polished by alumina powder (0.05 μm) and washed by ethanol for three times, respectively. Then, 5 μL 0.6 mg mL−1 functionalized MoS2 solution, 5 μL chitosan (8 wt%) and 10 μL Milli-Q water are mixed. Finally, 5 μL mixture is immobilized onto the GC electrode to obtain a TPA–MoS2/GC electrode.
2.4. Cd2+ detection by TPA–MoS2/GC electrode
Bismuth film is plated on the TPA–MoS2/GC electrode through the electrodeposition of 10 mg L−1 Bi3+ for 210 s with stirring. After coating bismuth film, Cd2+ is monitored by the TPA–MoS2/GC electrode in 8 mL 100 mmol L−1 acetate buffer (pH 6.5).
3. Results and discussion
3.1. TEM characterization of MoS2
MoS2 was obtained through ultrasonic exfoliation method. Fig. 1 showed representative TEM images of the MoS2 nanosheets (A), the functionalized MoS2 (B) and the functionalized MoS2 deposited with bismuth and cadmium (C). It suggested that the prepared MoS2 nanosheets were very thin and clear. After TPA non-covalent modified on the MoS2 nanosheets, the original morphology of the MoS2 nanosheets was retained in the composites. Fig. 1C is a TEM image of the TPA–MoS2–Bi–Cd nanocomposites and clearly showed the modification of the MoS2 by Bi and Cd nanocluster. As shown in Fig. S1,† TPA–MoS2 surface becomes rough and there are lots of nanoparticles on the surface of TPA–MoS2 after detection. It is consistent with TEM images. FTIR was also used to confirm the formation of TPA on the MoS2 nanosheets. As shown in Fig. 2, the peak of 2550 cm−1 was the SH group of TPA. After TPA modified on the MoS2 nanosheets, the absorption bands at 1390 (symmetric stretching of –COOH), 1600 (asymmetric stretching of –COOH), and 3000–3500 cm−1 (NH3+ stretch) became weak. The change in their dipole moment was due to TPA modification on the MoS2 nanosheets. These results showed the successful modification of TPA on the MoS2 nanosheets. X-ray photoelectron spectroscopy (XPS) was used to determine the chemical composition and states of the TPA–MoS2 with cadmium. As shown in Fig. S2A,† two peaks at 229 and 232 eV energy values ascribed to Mo core peaks, and the peak at 226 eV ascribed to S core peak. Fig. S2B† showed two peaks at 163 and 161.7 eV. These binding energy values are consistent with the expected charge states of Cd2+, Mo4+ and S2−. Furthermore, the specific surface area of the MoS2 nanosheets was estimated by the Brunauer–Emmett–Teller (BET) method. Its specific surface area achieved to be 603 m2 g−1. The high effective surface area and high-quality of MoS2 could provide good performances for GC electrode.
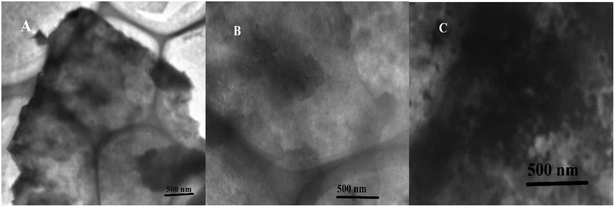 |
| Fig. 1 TEM image of MoS2 nanosheets (A), the TPA functionalized MoS2 (B), and the TPA–MoS2 with bismuth and cadmium (C). | |
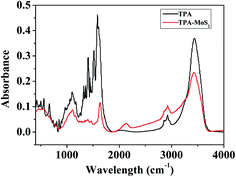 |
| Fig. 2 FTIR image of TPA and TPA–MoS2. | |
3.2. Electrochemical characterization of the TPA–MoS2-modified GC electrode
Cyclic voltammetry (CV) was an electrochemical measurement method which could be used for monitoring the preparation procedure of GC electrode. As shown in Fig. 3, the immobilization process of the TPA–MoS2/GC electrode was performed. Fig. 3 displayed the CVs of the bare GC, MoS2/GC and TPA–MoS2/GC electrodes in 2 mmol L−1 K3[Fe(CN)6]/K4[Fe(CN)6] solution. The CV of the bare GC electrode was highest among these electrodes. It demonstrated that the electron transfer rate between [Fe(CN)6]3−/4− and the bare electrode was very fast. The CV of the TPA–MoS2/GC electrode was the lowest, which demonstrated that a chitosan film and TPA–MoS2 had covered on the surface of the bare GC electrode. Without modification of TPA, the MoS2 nanosheets possessed good conductivity, and the CV of the chitosan–MoS2/GC electrode was higher than the TPA–MoS2/GC electrode. The results might be due to the introduction of TPA, which played a key role in improving the binding sites of Cd2+. It also showed that the TPA–MoS2 and chitosan film had covered on the GC electrode.
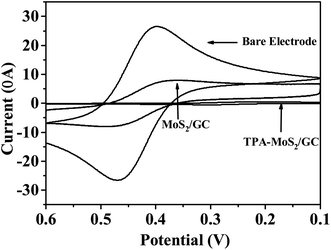 |
| Fig. 3 Cyclic voltammograms of bare GC, MoS2/GC and TPA–MoS2/GC electrodes in 2 mmol L−1 [Fe(CN)6]3−/4− at a scan rate of 100 mV s−1. | |
3.3. The TPA–MoS2-modified GC electrode for Cd2+ analysis
In this study, DPASV was used for detecting Cd2+. Fig. 4A showed that the DPASVs of the MoS2/GC and the TPA–MoS2/GC electrodes increased with the concentrations of Cd2+ from 0.5 to 100 μg L−1 in 100 mmol L−1 acetate buffer (pH 6.5) with 10 mg L−1 Bi3+ electrodepositing for 210 s. After the concentrations of Cd2+ achieved 150, 200 and 250 μg L−1, the DPASVs of these electrodes increased unobvious. The DPASVs response signals increased linear from 0.5 to 100 μg L−1 then become flat. As shown in Fig. 4B, the current peaks increased linearly with Cd2+ concentration, and the correlation coefficients was approximate 0.99. The sensitivity of the MoS2/GC and the TPA–MoS2/GC electrodes were 101.8 and 178.2 mA cm−2 m−1, respectively. Furthermore, the detection limit (DL) was an important parameter as sensors. The DL of the TPA–MoS2/GC and the MoS2/GC electrodes achieved 0.2 μg L−1 and 0.5 μg L−1 at a signal-to-noise ratio of 3, respectively. It demonstrated that the DL (0.2 μg L−1) of the TPA–MoS2/GC electrode was much lower than the standard for detecting tap water quality in China (GB 5749-2006, Cd 0.005 mg L−1). The DL of the TPA–MoS2/GC electrode was superior to that of the chitosan-multiwalled carbon nanotubes/GC electrode (0.4 μg L−1, electrodepositing Bi3+ for 300 s). Reproducibility was another important parameter of sensors. As shown in Fig. 5, 10 repeated measurements for 50 μg L−1 Cd2+ had excellent reproducibility with relative standard deviation (RSD) of 0.41%. Therefore, this prepared sensor could be potentially applied to rapid detection of Cd2+ in the aquaculture water.
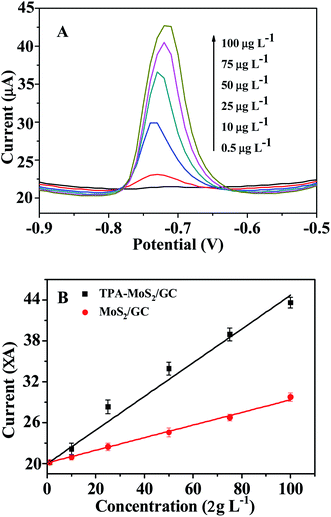 |
| Fig. 4 (A) DPASVs obtained for different concentrations of Cd2+ using a bismuth-coated TPA–MoS2/GC electrode; from bottom to top, 0.5, 10, 25, 50, 75, 100 μg L−1 with 10 mg L−1 Bi3+ electrodeposited for 210 s under stirring in 100 mmol L−1 acetate buffer (pH 5.0). (B) Calibration curves of MoS2/GC (red color) and TPA–MoS2/GC (black color) electrodes for Cd2+. | |
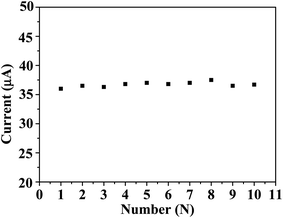 |
| Fig. 5 The stability of 10 repetitive measurements of 50 μg L−1 Cd2+ in 0.1 mol L−1 acetate buffer (pH 5.0) containing 3 mg L−1 Bi3+. | |
3.4. The TPA–MoS2/GC electrode testing aquaculture water samples
This sensor was used for detecting Cd2+ in the aquaculture water samples. The test samples were directly added into the bottle without enrichment, and then detected by the TPA–MoS2/GC electrode according to previous procedure. The Cd2+ standard solution (50 μg L−1) was added into the bottle to calculate and correct the results of the test samples. A 0.22 μm filter membrane was used for extracting 6 aquaculture water samples. As shown in Table 1, the Cd2+ concentration in aquaculture water A and aquaculture water D were 1.9 and 2.1 μg L−1, respectively. For other samples (sample B, sample C, sample E and sample F), there was no Cd2+. The concentration of aquaculture water samples was validated by AAS. It indicated that the sensor was a reliable and sensitive tool for the rapid detection of Cd2+ in real samples.
Table 1 Comparison of Cd2+ concentration in different aquaculture water samples by the sensor and AAS method
Sample |
Concentration (μg L−1) (sensor method) |
Concentration (μg L−1) (AAS method) |
Differencea (%) |
Difference = (sensor(value) − AAS(value))/AAS(value) × 100%. |
A |
1.9 |
1.7 |
11.76 |
B |
— |
— |
— |
C |
— |
— |
— |
D |
2.1 |
2.4 |
−12.5 |
E |
— |
— |
— |
F |
— |
— |
— |
The good sensing performance of the TPA–MoS2/GC electrode might be attributed to the following factors: the large surface area of MoS2 greatly increased the active surface area of the GC electrode for Cd2+ adsorption, and the abundant thiol group of TPA significantly increased the binding sites for Cd2+ (Scheme 1); the good electric conductivity of MoS2 could improve the electron transfer rate between the surface of the GC electrode and Cd2+ in the bulk solution; and the 2D nanostructure provided a very broad space for the easy transfer of Cd2+. Based on these reasons, the TPA–MoS2/GC electrode exhibited low DL and good reproducibility (Table 2). Thus, the TPA–MoS2/GC electrode showed greater potential for use as a “pre-alarm” tool.
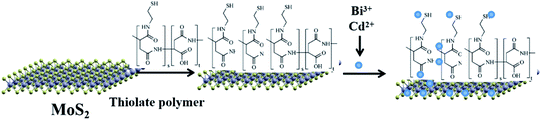 |
| Scheme 1 Schematic diagram of Cd2+ absorption on TPA–MoS2 nanocomposites. | |
Table 2 Analysis of Cd2+ by different reported methods
Electrode |
Detection limit (μg L−1) |
Deposition time (s) |
Ref. |
TPA–MoS2 |
0.17 |
210 |
Present work |
Bi-coated GC |
50 |
120 |
54 |
Bi-GP-CNT/GC |
0.6 |
150 |
55 |
Bi-Nafion/GC |
1.39 |
120 |
56 |
4. Conclusion
In summary, the TPA–MoS2/GC electrode was prepared for Cd2+ detection in the aquaculture water samples. It showed that the TPA–MoS2 nanocomposites offered significant advantages, such as low DL and good reproducibility. The good results were attributed to the large specific surface area, abundant thiol group from TPA and high electrical conductivity of the TPA–MoS2 nanocomposites. Furthermore, the bismuth electrode provided more environmental-friendly electrode for researcher health. The TPA–MoS2/GC electrode possessed greatly potential for its application in the on-site rapid analysis of Cd2+ in the water or food.
Conflicts of interest
There is no conflicts of interest.
Acknowledgements
This work was supported by the Central Public-interest Scientific Institution Basal Research Fund, CAFS (No. 2020GH09 and 2020TD75).
References
- Q. Zhao, H. Wang, Y. Du, H. J. Rogers, Z. Wu, S. Jia, X. Yao, F. Xie and W. Liu, J. Agric. Food Chem., 2020, 68, 1974–1985 CrossRef CAS.
- C. O. Ogunkunle, D. A. Odulaja, F. O. Akande, M. Varun, V. Vishwakarma and P. O. Fatoba, J. Biotechnol., 2020, 310, 54–61 CrossRef CAS.
- Y. S. Kim, M. Y. Song, J. S. Kim, D. S. Rha, Y. J. Jeon, J. E. Kim, H. Y. Ryu, I. J. Yu and K. S. Song, Toxicol. Res., 2009, 25, 140–146 CrossRef CAS.
- A. Al-Ghafari, E. Elmorsy, E. Fikry, M. Alrowaili and W. G. Carter, PLoS One, 2019, 14, e0225341 CrossRef CAS.
- L. Knani, M. Venditti, S. Kechiche, M. Banni, I. Messaoudi and S. Minucci, Toxicol. Mech. Methods, 2019, 1–9 Search PubMed.
- X. Li, R. Li, J. Yan, Y. Song, J. Huo, Z. Lan, J. Chen and L. Zhang, J. Appl. Toxicol., 2020, 40, 352–362 CrossRef CAS.
- P. Chen, X. Duan, M. Li, C. Huang, J. Li, R. Chu, H. Ying, H. Song, X. Jia, Q. Ba and H. Wang, Toxicol. Appl. Pharmacol., 2016, 310, 150–158 CrossRef CAS.
- IARC Monogr. Eval. Carcinog. Risk Chem. Man, 1976, 11, 1–293 Search PubMed.
- N. Degraeve, Mutat. Res., 1981, 86, 115–135 CAS.
- B. Yan, M. P. Isaure, S. Mounicou, H. Castillo-Michel, W. De Nolf, C. Nguyen and J. Y. Cornu, Environ. Pollut., 2020, 260, 113987 CrossRef CAS.
- N. Zhang, K. Shen, X. Yang, Z. Li, T. Zhou, Y. Zhang, Q. Sheng and J. Zheng, Food Chem., 2018, 264, 462–470 CrossRef CAS.
- N. A. Kasa, E. Akkaya, B. T. Zaman, G. Cetin and S. Bakirdere, Environ. Monit. Assess., 2018, 190, 589 CrossRef.
- M. Shirani, S. Habibollahi and A. Akbari, Food Chem., 2019, 281, 304–311 CrossRef CAS.
- O. Zverina, J. Kuta, P. Coufalik, P. Koseckova and J. Komarek, Food Chem., 2019, 298, 125084 CrossRef CAS.
- B. Paull, E. Twohill and W. Bashir, J. Chromatogr. A, 2000, 877, 123–132 CrossRef CAS.
- S. Tanikkul, J. Jakmunee, S. Lapanantnoppakhun, M. Rayanakorn, P. Sooksamiti, R. E. Synovec, G. D. Christian and K. Grudpan, Talanta, 2004, 64, 1241–1246 CrossRef CAS.
- M. Xu, R. Ma, C. Huang, G. Shi, T. Zhou and J. Deng, Anal. Chim. Acta, 2020, 1096, 174–183 CrossRef CAS.
- M. Zhou, Y. Wu, J. Zhang, Y. Zhang, X. Chen, J. Ye and S. Wang, Anal. Sci., 2019, 35, 283–287 CrossRef CAS.
- K. M. Hassan, G. M. Elhaddad and M. AbdelAzzem, Mikrochim. Acta, 2019, 186, 440 CrossRef.
- Y. Chen, D. Zhang, D. Wang, L. Lu, X. Wang and G. Guo, Talanta, 2019, 202, 27–33 CrossRef CAS.
- L. Wu, X. Fu, H. Liu, J. Li and Y. Song, Anal. Chim. Acta, 2014, 851, 43–48 CrossRef CAS.
- M. F. De Oliveira, A. A. Saczk, L. L. Okumura, A. P. Fernandes, M. De Moraes and N. R. Stradiotto, Anal. Bioanal. Chem., 2004, 380, 135–140 CrossRef.
- E. Nagles, V. Arancibia, C. Rojas and R. Segura, Talanta, 2012, 99, 119–124 CrossRef CAS.
- Z. J. Suturovic, S. Z. Kravic, Z. S. Stojanovic, A. D. Durovic and T. Z. Brezo-Borjan, J. Anal. Methods Chem., 2019, 2019, 3579176 Search PubMed.
- M. Mladenov, V. Mirceski, I. Gjorgoski and B. Jordanoski, Bioelectrochemistry, 2004, 65, 69–76 CrossRef CAS.
- N. B. Li, W. W. Zhu, J. H. Luo and H. Q. Luo, Analyst, 2012, 137, 614–617 RSC.
- H. Li, J. Li, Z. Yang, Q. Xu, C. Hou, J. Peng and X. Hu, J. Hazard. Mater., 2011, 191, 26–31 CrossRef CAS.
- S. Cerovac, V. Guzsvany, Z. Konya, A. M. Ashrafi, I. Svancara, S. Roncevic, A. Kukovecz, B. Dalmacija and K. Vytras, Talanta, 2015, 134, 640–649 CrossRef CAS.
- J. Tu, Y. Gan, T. Liang, Q. Hu, Q. Wang, T. Ren, Q. Sun, H. Wan and P. Wang, Front. Chem., 2018, 6, 333 CrossRef.
- Q. Wang, Z. Zhou, Y. Zhai, L. Zhang, W. Hong, Z. Zhang and S. Dong, Talanta, 2015, 141, 247–252 CrossRef CAS.
- J. Liu, L. Han, T. Wang, W. Hong, Y. Liu and E. Wang, Chem.–Asian J., 2012, 7, 2824–2829 CrossRef CAS.
- J. M. Lim, D. Kim, Y. G. Lim, M. S. Park, Y. J. Kim, M. Cho and K. Cho, J. Mater. Chem. A, 2015, 3, 7066–7076 RSC.
- L. O. Jones, M. A. Mosquera, M. A. Ratner and G. C. Schatz, ACS Appl. Mater. Interfaces, 2020, 12, 4607–4615 CrossRef CAS.
- Z. Han, Z. Tang, K. Jiang, Q. Huang, J. Meng, D. Nie and Z. Zhao, Biosens. Bioelectron., 2020, 150, 111894 CrossRef CAS.
- Z. Chen, C. Liu, J. Liu, J. Li, S. Xi, X. Chi, H. Xu, I. H. Park, X. Peng, X. Li, W. Yu, X. Liu, L. Zhong, K. Leng, W. Huang, M. J. Koh and K. P. Loh, Adv. Mater., 2020, 32, e1906437 CrossRef.
- F. I. Alzakia, W. Sun, S. J. Pennycook and S. C. Tan, ACS Appl. Mater. Interfaces, 2020, 12, 3096–3103 CrossRef CAS.
- W. Lu, P. Yu, M. Jian, H. Wang, H. Wang, X. Liang and Y. Zhang, ACS Appl. Mater. Interfaces, 2020, 12, 16822 CrossRef.
- C. L. C. Rodriguez, P. A. R. Munoz, K. Z. Donato, L. Seixas, R. K. Donato and G. J. M. Fechine, Phys. Chem. Chem. Phys., 2020, 22, 1457–1465 RSC.
- E. M. Alexeev, D. A. Ruiz-Tijerina, M. Danovich, M. J. Hamer, D. J. Terry, P. K. Nayak, S. Ahn, S. Pak, J. Lee, J. I. Sohn, M. R. Molas, M. Koperski, K. Watanabe, T. Taniguchi, K. S. Novoselov, R. V. Gorbachev, H. S. Shin, V. I. Fal'ko and A. I. Tartakovskii, Nature, 2019, 567, 81–86 CrossRef CAS.
- M. M. Furchi, A. Pospischil, F. Libisch, J. Burgdorfer and T. Mueller, Nano Lett., 2014, 14, 4785–4791 CrossRef CAS.
- S. Tsoi, P. Dev, A. L. Friedman, R. Stine, J. T. Robinson, T. L. Reinecke and P. E. Sheehan, ACS Nano, 2014, 8, 12410–12417 CrossRef CAS.
- R. Zhang, Y. Qin, P. Liu, C. Jia, Y. Tang and H. Wang, ChemSusChem, 2020, 13, 1354–1365 CrossRef CAS.
- Z. Wang, Q. Tu, S. Zheng, J. J. Urban, S. Li and B. Mi, Nano Lett., 2017, 17, 7289–7298 CrossRef CAS.
- Y. Yuan, H. Lv, Q. Xu, H. Liu and Y. Wang, Nanoscale, 2019, 11, 4318–4327 RSC.
- Y. Gao, K. Huang, X. Wu, Z. Hou and Y. Liu, J. Alloys Compd., 2018, 741, 174–181 CrossRef CAS.
- A. Dankert, L. Langouche, M. V. Kamalakar and S. P. Dash, ACS Nano, 2014, 8, 476–482 CrossRef CAS.
- H. Liu, M. Si, S. Najmaei, A. T. Neal, Y. Du, P. M. Ajayan, J. Lou and P. D. Ye, Nano Lett., 2013, 13, 2640–2646 CrossRef CAS.
- W. Zhang, Y. Song, S. J. He, L. Shang, R. N. Ma, L. P. Jia and H. S. Wang, Nanoscale, 2019, 11, 20910–20916 RSC.
- P. Bi and W. Hong, Mater. Chem. Phys., 2020, 255, 123577–123583 CrossRef CAS.
- D. Ma, J. Yu, W. Yin, X. Zhang, L. Mei, Y. Zu, L. An and Z. Gu, Chemistry, 2018, 24, 15868–15878 CrossRef CAS.
- Y. Chen, X. Wu and K. Huang, Sens. Actuators, B, 2018, 270, 179–186 CrossRef CAS.
- H. L. Shuai, X. Wu and K. J. Huang, J. Mater. Chem. B, 2017, 5, 5362–5372 RSC.
- A. Gupta, V. Arunachalam and S. Vasudevan, J. Phys. Chem. Lett., 2016, 7, 4884–4890 CrossRef CAS.
- J. Wang, J. Lu, S. B. Hocevar, P. A. Farias and B. Ogorevc, Anal. Chem., 2000, 72, 3218–3222 CrossRef CAS.
- X. Xuan and J. Y. Park, Sens. Actuators, B, 2018, 255, 1220–1227 CrossRef CAS.
- J. Li, J. Zhang, H. Wei and E. Wang, Analyst, 2009, 134, 273–277 RSC.
Footnotes |
† Electronic supplementary information (ESI) available. See DOI: 10.1039/d0ra06197b |
‡ Authors equally contribution to this work. |
|
This journal is © The Royal Society of Chemistry 2020 |
Click here to see how this site uses Cookies. View our privacy policy here.