DOI:
10.1039/D0RA07330J
(Paper)
RSC Adv., 2020,
10, 37735-37742
An ICT-based fluorescence enhancement probe for detection of Sn2+ in cancer cells†
Received
26th August 2020
, Accepted 7th October 2020
First published on 13th October 2020
Abstract
Development of a novel fluorescence enhancement probe for detection of Sn2+ in organisms, with high selectivity and sensitivity, is of great interest but remains a great challenge. Herein, an ICT-based fluorescence probe TPPB was rationally developed to act as an ‘enhancement’ luminescent and “naked-eye” indicator for Sn2+ detection. Importantly, spectroscopic studies indicated that TPPB was a fluorescence enhancement sensor for Sn2+ with rapid response, low detection limit (0.116 μM) and excellent binding constant (1.6 × 104 M−1). The mechanism of TPPB response to Sn2+ was further proved by 1H NMR titration, and enhancement calculations. Furthermore, TPPB is applied as a fluorescence probe for imaging in Hela cells, indicated that it can be potentially applied for Sn2+ sensing in biological fields.
Introduction
The study of the methods used to detect metal ions has attracted the interest of analysts and has been developed rapidly, due to the metal ions not being biodegradable.1 Stannous chloride (SnCl2) is a mild Lewis acid that is widely used as a reducing agent and catalyst in various synthetic organic operations and in the production of biodegradable polylactic acid.2–4 In inorganic synthesis, SnCl2, which acts as an effective reducing agent, could reduce metal salts, such as silver and gold salts to their metals, Cu2+ to Cu+, and Fe3+ to Fe2+.5 Particularly, Sn2+ species have been used in articles of daily use, such as tin-plating of steel to make tin cans,6 added to some canned and bottled foods,7 and mouth wash.8 In additional, tin is an essential trace micronutrient for living organisms.9,10 For example, a deficiency of tin can enlarge the risks correlated with growth factors and cancer prevention, while an excess concentration of tin has a negative effect on the breathing, digestive, and nervous systems.11 Therefore, it is significant to develop an accurate, simple, and efficient way to detect Sn2+.12
Sn2+ ions have routinely been determined by flame atomic absorption spectrometry,13 potentiometry,14 voltammetry,15 and UV-vis spectrophotometry.16 Compare with these analytical approaches, fluorescent sensors have been developed for the determination of various important chemical species, as they offer significant advantages of excellent sensitivity, simplicity, instantaneous response and low detection limit up to nanomolar scale by contrast with routine analysis.17–19 Therefore, the study of fluorescence probe as the high selectivity and sensitivity detection of Sn2+ is urgently desirable. Hitherto many of fluorescence probes for the detection of Sn2+, which sensing mechanisms are mainly based on chemical reaction, complexation reaction, quantum dots, and nanoparticles, have been established.20–22 However, Sn2+-selective sensors base on chemical reaction and complexation reaction have rarely been reported.23 Fluorescence probes, which based on complexation reaction between the probe and analyte, have become more and more precise, sensitive and popular in research. While the fluorescence quenching probe is more common in research when metal ions are bound to fluorophore receptor system, but other interference factors could cause the quenching of the fluorescence so that its sensitivity and selectivity will be reduced and inferior to the fluorescence enhancement probe.24,25 Therefore, the fluorescence enhancement probe is highly preferable than the fluorescence quenching probe for practical applications about detecting heavy metal ions like Sn2+, due to the higher sensitivity, selectivity, and reliability. The fluorescence enhancement probe is also quenched in heavy metal ions detection via enhancement spin–orbit coupling energy or electron transfer. For overcoming this challenge, sensors structures are often designed to contain specific moieties,26–36 such as triphenylamine.37 Recently, we have reported a probe of 2-imidazole-pyridine derivatives with emission at 446 nm by utilizing the nitrogen of imidazole and pyridine to form complex with metal ion, but it shown fluorescence quenched behavior after coordinating with metal ion (Fig. 1).38 In this work, Schiff base structural unit was designed to replace the imidazole to form weak electron-withdrawing center. Furthermore, diethylamino and triphenylamine moiety are strong electron-donating groups and thus are suitable as donor in an intramolecular charge transfer (ICT) system. The ICT process of free probe is weak due to the weak electron-withdrawing center. However, there is a strong ICT process after the N of Schiff base and pyridine coordinating with Sn2+ due to the weak electron-withdrawing center changing to strong electron-withdrawing center. The drastic change in ICT efficiency should elicit a fluorescence enhancement response.
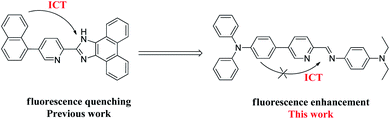 |
| Fig. 1 Molecular structure of probe TPPB. | |
Based on the above considerations, herein, we report a new ICT-based sensor TPPB (Fig. 1). Characterizations including 1H and 13C NMR spectra, and MS spectrum are utilized to confirm the chemical structure of sensor TPPB. The probe TPPB shows a fluorescence enhancement response to Sn2+. This enables the probe to perform the detection of Sn2+ with good sensitivity, and prominent selectivity. Furthermore, the probe was successfully imaged for Sn2+ in living cells with low cytotoxicity. It is a suitable research tool to study the role of Sn2+ in a biological environment.
Experimental section
Materials and methods
All reagents for synthesis were analytically pure. All the solvents for spectroscopic measurement were chromatographically pure. 1H NMR spectra were recorded at 400 MHz, in CDCl3 solution on a Bruker AV400 MHz spectrometer and chemical shifts were recorded in parts per million (ppm) with TMS as the internal reference. Mass spectra (MS) were obtained on a QTRAP LC/MS/MS system (API2000; Applied Biosystems, Foster City, CA, USA), and signals were given in m/z. Fluorescence spectra were determined with a Hitachi F-4600 fluorescence spectrophotometer. Photoluminescence (PL) quantum yields were determined using a Hamamatsu system for absolute PL quantum yield measurements (type C11347).
Synthesis of compound TPPB
The synthetic method of compound TPPB was shown in Scheme 1. Compound 5-(4-(diphenylamino)phenyl)picolinaldehyde (M-1) was prepared according to the literature method.39 Ethanol (10 mL), N,N-diethylbenzene-1,4-diamine (0.173 g, 1.05 mmoL) were stirred at room temperature, then, M-1 (0.35 g, 1.00 mmoL) was added to the mixed solution under reflux for 2 h. After that the mixed solution was poured into 100 mL ice water, extracted with dichloromethane (3 × 30 mL), and evaporated under reduced pressure to remove the organic solvent. The product was isolated using silica gel column chromatography with petroleum ether/ethyl acetate = 5 as the solvent to yield faint yellow solid (0.39 g, 78.6% yield). 1H NMR (400 MHz, CDCl3) δ (ppm): 8.89 (s, 1H, Py-H), 8.70 (s, 1H, CH
N), 8.21 (d, J = 8.3 Hz, 1H, Py-H), 7.94 (d, J = 8.3 Hz, 1H, Py-H), 7.52 (d, J = 8.2 Hz, 2H, Ar-H), 7.38 (d, J = 8.5 Hz, 2H, Ar-H), 7.29 (t, J = 7.6 Hz, 4H, Ar-H), 7.16 (t, J = 7.0 Hz, 6H, Ar-H), 7.07 (t, J = 7.3 Hz, 2H, Ar-H), 6.72 (d, J = 8.6 Hz, 2H, Ar-H), 3.41 (q, J = 7.0 Hz, 4H, CH2), 1.20 (t, J = 7.0 Hz, 6H, CH3); 13C NMR (100 MHz, CDCl3) δ (ppm):154.57, 154.25, 148.70, 147.94, 147.82, 139.15, 136.82, 134.37, 131.18, 129.79, 128.15, 125.56, 125.23, 123.84, 123.64, 121.64, 112.44, 44.96, 13.08; EI-MS m/z: [M + 1] 497.3.
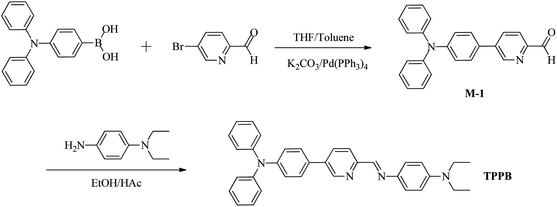 |
| Scheme 1 The synthetic pathways of probe TPPB. | |
Results and discussion
Synthesis and characterization
The sensor TPPB was prepared according to Scheme 1. Initially the intermediates M-1 was synthesized by the straightforward cross-coupling reaction under standard Suzuki coupling condition.40 The Schiff base sensor TPPB was prepared in 78.6% yield as yellow solid from the condensation of 5-(4-(diphenylamino)phenyl)picolinaldehyde with N,N-diethylbenzene-1,4-diamine in ethyl alcohol under refluxing condition. 1H NMR, 13C NMR, and MS spectra were used to confirm the chemical structure of probe TPPB. All the protons and carbon atoms (Fig. S1–S3†) were unambiguous assigned.
Optical analysis of probe TPPB sensing Sn2+
The selectivity of the TPPB probe for Sn2+ ion was researched by optical studies of the probe with various metal ions, and the sensitivity of the probe TPPB was also monitored by titration analysis. We firstly proceeded to examine the selectivity of the sensor TPPB to the various metal ions due to the selectivity is an important characteristic feature of an ion-selective sensor. We tested the selectivity of probe TPPB (20 μM) for Sn2+ (4.5 equiv.) with possible interferences including metal ion salts of K+, Ag+, Zn2+, Pb2+, Sr2+, Mg2+, Mn2+, Hg2+, Cd2+, Ca2+, Ba2+, Cr2+, Al3+, Fe3+ (4.5 equiv.) in THF solution, respectively (Fig. 2A). Remarkably, only Sn2+ elicited a large fluorescence enhancement at 561 nm with excitation at 420 nm, due to the strong ICT process, which was resulted by the complexation of Sn2+ with N of pyridine and Schiff base, the electron-withdrawing ability of the recognition site was enhanced. By contrast, almost all the other interfering metal ions have no observable fluorescence response. Fig. 2B shown the color of the probe TPPB changed from very pale yellow to deep yellow with Sn2+ added under UV irradiation, while the color intensity of the probe TPPB did not change with other metal ions added, except that Al3+, Cr2+ and Fe3+ brought about with slight enhancement. This may be due to the different electrical properties and inappropriate diameters of different metal ions. In Fig. 2C, the free TPPB can be seen as yellow solution. The color of the solution underwent change from yellow to colorless and transparent in the presence of Sn2+, however other metal ions did not bring about any obvious change in the color of the solution, except that Zn2+, Cd2+, and Hg2+ brought about with yellow enhancement. So, TPPB can be used as visual indictor for Sn2+. This technique is therefore superior to other analytical techniques because it has ability to detect Sn2+ by naked-eye.
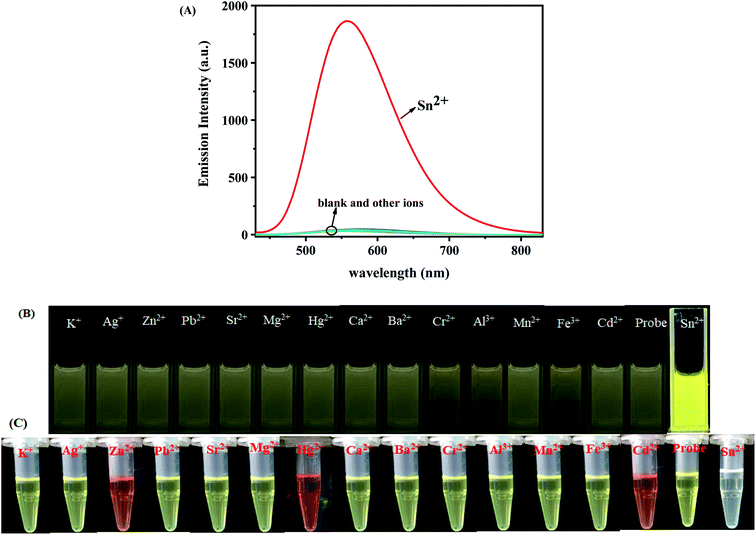 |
| Fig. 2 (A) Fluorescence spectral of probe TPPB (20 μM) in the presence of Sn2+ (4.5 equiv.) and various of other metal ions (4.5 equiv.) in THF solution at room temperature, λex = 420 nm; (B) the color changes of TPPB solution (20 μM) when a various metal ions (4.5 equiv.) were added under UV-light; (C) naked eyes color changes of the probe with different metal ions. | |
Moreover, in order to explore the utility of TPPB as an ion-selective sensor, the competition experiments were carried out by adding other competitive metal ions to TPPB solution in presence of Sn2+ (Fig. 3). The black bar corresponds to the fluorescence probe TPPB with all of metal ions respectively, and red bar corresponds to the fluorescence probe TPPB with all of metal ions in presence of Sn2+ respectively. Interestingly, Sn2+ induced fluorescence responses were hardly influenced by these common coexistent metal ions. The evidence revealed that the probe TPPB shown a high selectivity and good stability toward to Sn2+ even in the presence of other relevant metal ions.
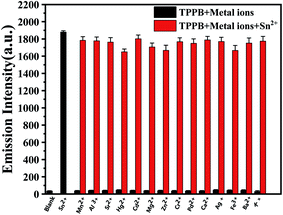 |
| Fig. 3 Fluorescence response of TPPB with Sn2+ ion in coexistence of other metal ions at λex = 420 nm, λem = 561 nm. | |
The sensitivity of the probe TPPB was monitored by titration analysis. The fluorescence titrations of TPPB with Sn2+ were performed. As shown in Fig. 4A, probe TPPB exhibited extremely feeble fluorescence. However, with the addition of 0–4.5 equiv. of Sn2+, the fluorescence intensity showed an excellent enhancement (up to 63-fold) at 561 nm. The fluorescence enhancement could be attributed to the strong ICT process.
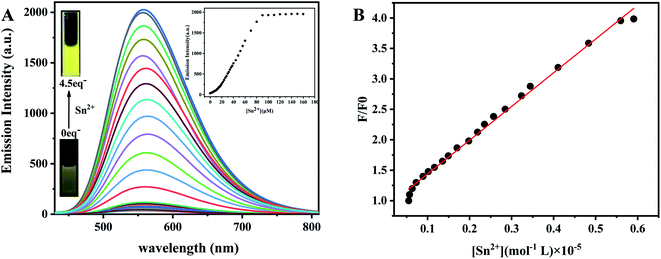 |
| Fig. 4 (A) Fluorescence spectra of TPPB upon incremental addition of Sn2+ (0–4.5 equiv.). Insert: the fluorescence intensity of TPPB in the presence of increasing Sn2+ concentration; (B) the linearity between F/F0 and Sn2+ concentration. | |
The large fluorescence enhancement was corroborated by the observation that the fluorescence color of the sensor solution turned from very pale yellow to deep yellow (Fig. 4A, inset), which indicated that the probe TPPB showing an excellent fluorescence enhancement behavior for selective detection of Sn2+ ion. Subsequently, an evident increasing observed in the quantum yield (Φ) of [TPPB + Sn2+] (68.21%) as compared to that of TPPB (1.43%) by using fluorescein (Φref = 90%) as a standard fluorescence reference (the calculation formula see ESI S2.1†). It supports the fluorescence enhancement of TPPB observed in presence of Sn2+, and suggesting a sensitive and selective detection of Sn2+ by TPPB compared to other metal ions. Importantly, the sensor shown a good linear relationship between the fluorescence intensity at 561 nm and the concentrations of Sn2+ from 1 to 6 μM (Fig. 4B), suggesting that sensor TPPB is potentially useful for quantitative determination of Sn2+ with a large dynamic range.
In order to confirm the binding stoichiometry between TPPB and Sn2+, the Job's plots analysis was carried out. The plot of fluorescence intensity against the molecular fraction of [TPPB]/[ Sn2+ + TPPB] was provided in Fig. S4A,† it showed the minimum at mole fraction of 0.5 indicating 1
:
1 stoichiometry between TPPB and Sn2+ in the complexes. Based on a 1
:
1 binding mode, apparent association constant (Ka) of the TPPB-Sn2+ interaction was calculated from the fluorescence titration spectra using the formula shown in calculation S2.3 (see ESI S2.3†) and Ka value was found to be 1.6 × 104 M−1. What's more, a good linear relationship of the fluorescence intensity as a function of [Sn2+] concentration from 0–4.5 equiv. (R = 0.99367) was obtained (Fig. S4B†). From the slope of the linear fit, the limit of detection (LOD) of the probe TPPB for Sn2+ ion was determined by using the formula shown in calculation S2.4 (see ESI S2.4†) and was found to be 0.116 μM (Fig. S4C†). Shortly, the probe TPPB could be a sensitive fluorescence probe for the quantitative detection of Sn2+ at micromole levels.
To further confirm the binding mechanism of Sn2+ with sensor TPPB, 1H NMR titration experiments were performed in CDCl3 and shown in Fig. 5. The changes in 1H NMR signals of TPPB are more apparent with the appearance of the new peak around 10.10 and 8.99 ppm when the analyte concentration is 0.2 equiv., and subsequently, it becomes gradually intensified with further additions. Owing to the precipitation, we were unable to continue the experiment beyond 1.5 equiv. of Sn2+ ions. The new signals are assigned to the proton of Ha and Hc, which are located in the vicinity of Sn2+ binding site. The high field shift of 0.09 ppm for the arene proton (Hb) shows that the electron density has decreased around Hb after Sn2+ binding, due to the π-electron density shifted to the carbon, which link with N. On the other hand, the chemical shifts of Hd and He, shifted from 7.93(d) and 8.21(d) ppm to 8.01(s) ppm. These results indicated that N acted as electron donors for coordination to Sn2+. The data further proved that the TPPB coordinating with Sn2+ with a stoichiometric ratio of 1
:
1.
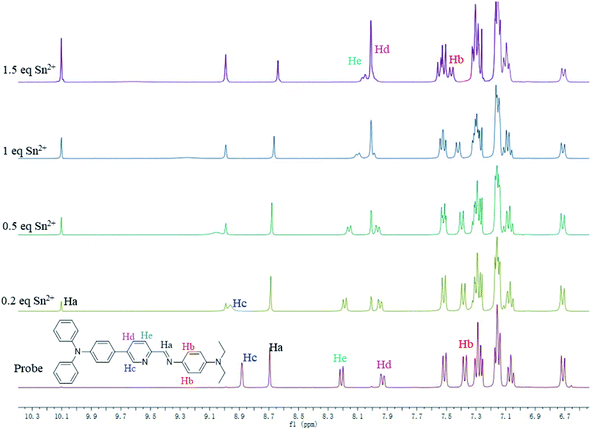 |
| Fig. 5 1H NMR titration spectra of TPPB with Sn2+ ions in CDCl3. | |
DFT calculations
DFT studies further support the Sn2+-assisted ICT process in TPPB. The optimized geometries of TPPB and TPPB-Sn2+ adduct, which were identified with the Job's plots analysis and 1H NMR titration experiments, have been generated using B3LYP/6-311G(d, p) level (Lanl2dz for Sn) with Gaussian 09 software.41 As shown in Fig. 6, the N1 and N2 were found to be arranged in an almost in-plane orientation in TPPB and TPPB-Sn2+ adduct, with a dihedral angle of −179.3° and 1.9°, respectively. The planar conformation provides efficient π-conjugation. After optimizing structure of TPPB, the N1 and N2 were trans-configuration, while they convert to cis-configuration in TPPB-Sn. The above phenomenon means that the ground-state geometry undergoes a significant twist upon the addition of Sn2+.
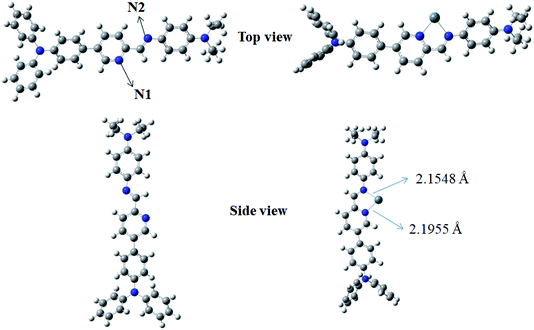 |
| Fig. 6 The optimized geometric structures based on B3LYP for TPPB and TPPB-Sn2+. Here, both top views and side views are shown. | |
Examination of the frontier molecular orbitals given in Fig. 7 suggests that the electron density in the HOMO and LUMO for TPPB is entirely localized on the pyridine, Schiff base and one benzene ring of triphenylamine moiety. The above phenomenon clearly demonstrates an obstruction of the ICT process in TPPB, resulting in a weak fluorescence emission. While after Sn2+ ion binding with TPPB, the electron density localized on the Schiff base and triphenylamine moiety in the HOMO then shifted to Sn2+ binding site during transition to LUMO, which indicates an ICT from the Schiff base and triphenylamine moiety to the Sn2+ binding site, resulting in a strong fluorescence emission. Moreover, the calculated energy difference between HOMO and LUMO (0.53 eV) of TPPB –Sn2+ is lower than that of the free TPPB (the HOMO to LUMO energy gap is 3.18 eV). Hence, the ICT process of TPPB-Sn is also energetically favorable.
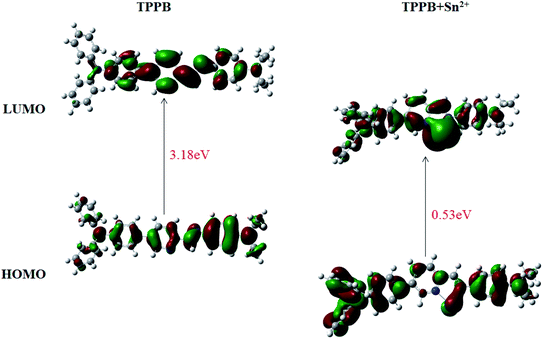 |
| Fig. 7 HOMO–LUMO energy diagrams of TPPB with Sn2+ ions. | |
Imaging of cancer cell
In order to demonstrate the potential application of TPPB for the detection of Sn2+ in biological media, fluorescence microscopy studies were carried out by using Hela cells. The cytotoxicity of TPPB against Hela cells and normal cells was measured on MTT assay. The appropriate result was achieved based on Hela cells and normal cells, which showed high livability with more than 80% survival after 24 h (Fig. S5†). It means probe TPPB shows low cytotoxicity against cells.
Furthermore, TPPB as the fluorescence probe imaged in Hela cells for detection Sn2+ was studied. As shown in Fig. 8, incubation of Hela cells with 10 μM of the probe TPPB for 30 min gave very low dim fluorescence in the intracellular region (Fig. 8B). After treatment with 100 μM Sn2+ for 30 min, the fluorescence intensity obvious enhancement in Hela cells (Fig. 8E). These results inferred that the fluorescence realized enhancement due to the intracellular uptake of Sn2+ result in form of complex TPPB-Sn2+. The above results providing direct evidence that the probe shows good biocompatibility with low cytotoxicity.
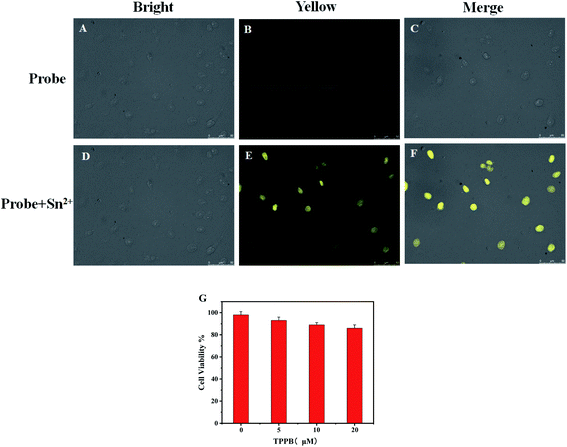 |
| Fig. 8 Fluorescence imaging of Hela cells incubated with TPPB (20 μM) for 30 min (A–C), and then with Sn2+ (200 μM) for another 30 min (D–F), (Left) bright filed image, (Middle) yellow channel, (Right) merge image; (G) the cytotoxicity of TPPB against Hela cells incubated with different concentration of TPPB for 24 h. | |
Conclusions
In conclusion, we have synthesized and characterized probe TPPB for detection Sn2+. Interaction of Sn2+ with TPPB induces the fluorescence enhancement response with emission at 561 nm due to ICT process. Probe TPPB, which shows low detection limit of 0.116 μM and strong association constant of 1.6 × 104 M−1, is a highly sensitive and selective sensor toward Sn2+, as demonstrated by selective, competitive and titration experiment. It could form complexes with Sn2+ with a stoichiometric ratio of 1
:
1. Importantly, the color of the probe TPPB changed from yellow to colorless and transparent with Sn2+ added under visible light, which meant that the probe can be used as visual indictor for Sn2+ by naked-eye. The possible recognition pattern was derived from DFT calculations, and 1H NMR titration. Impressively, the probe TPPB with low toxicity has practical application in cell imaging, which indicated that the probe is suitable for tracking intracellular Sn2+. In conclusion, TPPB can be used as a great promise candidate sensor for detection of Sn2+ in complex living samples.
Conflicts of interest
There are no conflicts to declare.
Acknowledgements
The authors are grateful for the financial support from the National Natural Science Foundation of China (21867011); Natural Science Foundation of Jiangxi Province (20192BAB213008); University (no. QD201907), the Outstanding Young and Middle-aged Scientific Innovation Team of Colleges and Universities of Hubei Province: “Biomass Chemical Technologies and Materials” (Grant No. T201908).
References
- L. M. Zhu, J. Yang, Q. S. Wang and L. T. Zeng, J. Lumin., 2014, 148, 161–164 CrossRef CAS.
- O. M. Singh and L. R. Devi, Mini-Rev. Org. Chem., 2013, 10, 84–96 CrossRef CAS.
- R. G. Clevenger, B. Kumar, E. M. Menuey, G. H. Lee, D. Patterson and K. V. Kilway, Chem.–Eur. J., 2018, 24, 243–250 CrossRef CAS.
- Z. Q. Lei, Y. B. Bai and S. F. Wang, Chin. Sci. Bull., 2005, 50, 2390–2392 CrossRef CAS.
- X. Y. Yue, X. G. Zhang and F. L. Qing, Org. Lett., 2009, 11, 73–76 CrossRef CAS.
- L. J. Curtman and J. K. Marcus, J. Am. Chem. Soc., 1914, 36, 1093–1103 CrossRef CAS.
- F. M. El-Demerdash, M. I. Yousef and M. A. Zoheir, Food Chem. Toxicol., 2005, 43, 1743–1752 CrossRef CAS.
- A. Wieg and T. Attin, J. Dent., 2014, 42, 1210–1215 CrossRef.
- H. Rüdel, Ecotoxicol. Environ. Saf., 2003, 56, 180–189 CrossRef.
- Y. Arakawa and K. Tomiyama, Nihon. Rinsho., 2016, 74, 1199–1206 Search PubMed.
- N. Cardarelli, Thymus, 1990, 15, 223–231 CAS.
- A. K. Mahapatra, S. K. Manna, K. Maiti, R. Maji, C. D. Mukhopadhyay, D. Sarkar and T. K. Mondal, RSC Adv., 2014, 4, 36615–36622 RSC.
- S. Ulusoy, H. İ. Ulusoy, M. Akçay and R. Gürkan, Food Chem., 2012, 134, 419–426 CrossRef CAS.
- I. A. Ismail and A. M. El-Kot, Microchem. J., 1991, 44, 49–53 CrossRef CAS.
- A. S. Dadda, A. C. Teixeira, P. K. Feltes, M. M. Campos, C. E. Leite and C. M. MoriguchiJeckel, J. Braz. Chem. Soc., 2014, 25, 1621–1629 CAS.
- M. Arvand, A. M. Moghimi, A. Afshari and N. Mahmoodi, Anal. Chim. Acta, 2006, 579, 102–108 CrossRef CAS.
- S. K. Kailasa, J. R. Koduru, M. L. Desai, T. J. Park, R. K. Singhal and H. Basu, Trac-Trend. Anal. Chem., 2018, 105, 106–120 CrossRef CAS.
- Z. Zhou, Y. Li, W. Su, B. Gu, H. Xu, C. Wu, P. Yin, H. Li and Y. Zhang, Sens. Actuators, B, 2019, 280, 120–128 CrossRef CAS.
- H. Xu, B. Gu, Y. Li, Z. Huang, W. Su, X. Duan, P. Yin, H. Li and S. Yao, Talanta, 2018, 180, 199–205 CrossRef CAS.
- J. Du, M. Zhao, W. Huang, Y. Deng and Y. He, Anal. Bioanal. Chem., 2018, 410, 4519–4526 CrossRef CAS.
- K. S. Patil, P. G. Mahajan and S. R. Patil, Spectrochim. Acta, Part A, 2017, 170, 131–137 CrossRef CAS.
- F. Firdaus, A. Farhi, M. Faraz and M. Shakir, J. Lumin., 2018, 199, 475–482 CrossRef CAS.
- J. H. Baek, M. G. Choi, N. Y. Kim and S. K. Chang, Sens. Actuators, B, 2019, 284, 562–567 CrossRef CAS.
- A. J. Moro, P. J. Cywinski, S. Körsten and G. J. Mohr, Chem. Commun., 2010, 46, 1085–1087 RSC.
- Z. Xu, S. J. Han, C. Lee, J. Yoon and D. R. Spring, Chem. Commun., 2010, 46, 1679 RSC.
- K. C. Song, M. H. Kim, H. J. Kim and S. K. Chang, Tetrahedron Lett., 2007, 48, 7464–7468 CrossRef CAS.
- J. Zhang, K. Zhang, X. Huang, W. Cai, C. Zhou, S. Liu, F. Huang and Y. Cao, J. Mater. Chem., 2012, 22, 12759–12766 RSC.
- H. Wang and W. H. Chan, Tetrahedron, 2007, 63, 8825–8830 CrossRef CAS.
- H. Mu, R. Gong, Q. Ma, Y. Sun and E. Fu, Tetrahedron Lett., 2007, 48, 5525–5529 CrossRef CAS.
- H. Yang, Z. G. Zhou, J. Xu, F. Y. Li, T. Yi and C. H. Huang, Tetrahedron, 2007, 63, 6732–6736 CrossRef CAS.
- R. Dwivedi, D. P. Singh, S. Singh, A. K. Singh, B. S. Chauhan, S. Srikrishna and V. P. Singh, Org. Biomol. Chem., 2019, 17, 7497–7506 RSC.
- L. N. Neupane, P. K. Mehta, J. U. Kwon, S. H. Park and K. H. Lee, Org. Biomol. Chem., 2019, 17, 3590–3598 RSC.
- X. M. Meng, L. Liu, H. Y. Hu, M. Z. Zhu, M. X. Wang, J. Shi and Q. X. Guo, Tetrahedron Lett., 2006, 47, 7961–7964 CrossRef CAS.
- E. M. Nolan, M. E. Racine and S. J. Lippard, Inorg. Chem., 2006, 45, 2742–2749 CrossRef CAS.
- J. Wang and X. Qian, Chem. Commun., 2006, 109–111 RSC.
- Y. F. Cheng, D. T. Zhao, M. Zhang, Z. Q. Liu, Y. F. Zhou, T. M. Shu, F. Y. Li, T. Yi and C. H. Huang, Tetrahedron Lett., 2006, 47, 6413–6416 CrossRef CAS.
- Y. Y. Zhu, H. Y. Xia, L. F. Yao, D. P. Huang, J. Y. Song, H. F. He, L. Shen and F. Zhao, RSC Adv., 2019, 9, 7176–7180 RSC.
- Y. Y. Zhu, Q. Sun, J. W. Shi, H. Y. Xia, J. L. Wang, H. Y. Chen, H. F. He, L. Shen, F. Zhao and J. Zhong, J. Photochem. Photobiol., A, 2020, 389, 112244 CrossRef CAS.
- J. L. Wang, C. Y. Chai, S. X. Xu, F. Zhao, H. Y. Xia and Y. B. Wang, Inorg. Chim. Acta, 2019, 484, 237 CrossRef CAS.
- N. Miyaura and A. Suzuki, Chem. Rev., 1995, 95, 2457 CrossRef CAS.
- M. J. Frisch, G. W. Trucks, H. B. Schlegel, G. E. Scuseria, M. A. Robb, J. R. Cheeseman, et al., Gaussian 09, revision A.01, Gaussian, Inc., Wallingford, CT, 2009 Search PubMed.
Footnote |
† Electronic supplementary information (ESI) available. See DOI: 10.1039/d0ra07330j |
|
This journal is © The Royal Society of Chemistry 2020 |
Click here to see how this site uses Cookies. View our privacy policy here.