DOI:
10.1039/D0RA08308A
(Paper)
RSC Adv., 2020,
10, 44312-44322
Degradation of pesticides using amine-functionalized cellulose nanocrystals†
Received
28th September 2020
, Accepted 1st December 2020
First published on 15th December 2020
Abstract
A series of amine-functionalized cellulose nanocrystal materials were successfully synthesized, characterized, and evaluated for the remediation of pesticide contaminants from organic and aqueous media. Their ability to degrade malathion in organic systems has been examined, resulting in up to 100% degradation of the compound into detectable lower molecular weight by-products. A poly(ethylenimine) cellulose nanocrystal (CNC-PEI) material was also capable of degrading aqueous solutions of malathion, deltamethrin, and permethrin with 100%, 95%, and 78% degradation, respectively. Thus, these materials can potentially serve as a new and viable remediation technique based on their ability to effectively degrade various pesticides. The reusability of the CNC-PEI was also explored. The CNC-PEI material maintained its ability to degrade malathion throughout two wash and re-use cycles.
Introduction
The use of pesticides in agriculture has undeniably played a significant role in improving crop yields as well as protecting against aggressive pests and invasive weeds. It is documented that approximately 2.4 million metric tons of pesticide active ingredients are applied annually worldwide.1,2 Furthermore, the rapidly increasing world population and resultant food demands have considerably increased the global use of pesticides. The pervasive application of pesticides has led to universal contamination, detectable in all facets of the ecosystem.1,2 This contamination poses several health hazards toward the public and non-target ecological species due to the sometimes toxic, relatively stable, and less soluble active ingredients of pesticide formulations.3,4 These active ingredients have aptly been classified as extremely hazardous class 1A, highly hazardous class 1B, moderately hazardous class 2 and slightly hazardous class 3 active ingredients by the World Health Organization.5
Due to the increased use of pesticides and the subsequent resistance developed by insects and invasive plants, the required dosage to counteract the target organism has increased over time. Unsurprisingly, this increased pesticide application along with the sometimes slow environmental degradation of residual pesticides has resulted in an accumulation of active ingredients and their degradants in the environment, resulting in water source contamination, soil quality degradation, biomagnification, and reduced biodiversity. Many reports concerning the negative effects on humans and ecosystems underscore the necessity to remediate pesticides and their residues, especially from aqueous sources.6,7
It is particularly difficult to develop a single, universal method for pesticide remediation.8–10 This is highlighted by the plethora of physical, chemical, and biological methods that have been explored to mitigate pesticide pollution such as coagulation/flocculation, photocatalytic degradation, electrochemical or aerobic degradation, oxidation, membrane filtration, nanofiltration, and adsorption.1,2,8–13 Microbial, photochemical, and/or chemical treatments are the primary degradative decontamination processes for the removal of pesticides in surface waters. There are numerous examples of microorganism-assisted methodologies for pesticide degradation. While effective, these approaches are time consuming, often requiring days to months for complete metabolism to occur, leading to increased expenses that are incompatible with industrial scale applications.14 Photochemical methods are effective although they also come with obstacles. For example, it is difficult to identify an appropriate light source to facilitate practical remediation efforts. Often, a compatible light source that was identified in the lab becomes unsuitable when applied in the field, due to either undesired photon absorption by the pesticide formulating agents or the increased opaqueness of environmentally relevant solutions.15–17
In aqueous systems the most common pathways applied to promote chemical degradation are hydrolysis and oxidation. Hydrolysis is an attractive method because it has proven to be economical and effective. Hydrolysis occurs via a water substitution or elimination reaction pathway. Whether the degradation occurs more rapidly at a high pH (alkaline hydrolysis) or a low pH (acid hydrolysis) varies among pesticides. The rate of hydrolysis also depends on the temperature, where increased temperatures (ca. 100 °C) are required for degradation to occur within 24 h.18 So called advanced oxidation processes (AOPs), require the addition of oxidants such as hydrogen peroxide/ferrous salts, TiO2 photocatalysts, or ozone, as well as formulating agents and surfactants to quench and remove the oxidative species.19–21 Currently, the limiting factor surrounding AOPs is the high cost of many of the necessary oxidants.22
A variety of nanomaterials have also been established as useful technologies for water pollutant remediation. Many examples can be found in the literature detailing the adsorption of pollutants, mitigation of pathogens, and degradation of toxins into less toxic derivatives.12,23–28 Cellulose-based materials, owing to their natural abundance, renewable sourcing, biodegradability, and biocompatibility, have been leveraged extensively for pollution remediation.29–32 More closely relevant to the work described herein, several groups have exploited the modification of cellulose fibers or particles with amine functionality to arrive at cellulose-poly(amine) materials that are capable of removing heavy metal ions, cationic and anionic dyes, and pharmaceuticals from contaminated water.33–42 Recently, our group has explored poly(ethylenimine) (i.e. PEI) functionalized nanoparticles, microparticles, cellulose nanocrystals, and kaolinite clay materials for VOC remediation.43–46 We have also explored the use of PEI-modified cellulose microcrystals for the removal of poly- and perfluorinated surfactants from water.47 In this study, we evaluated the use of a series of amine-functionalized cellulose nanocrystals (CNCs) to serve as a new material for the remediation of pesticide residues from water or organic solvent.
Herein, we highlight the ability of amine-functionalized CNCs to serve as effective tools for the degradation of various pesticides into their respective lower molecular weight by-products in both organic and aqueous media. Namely, ethylenediamine, tris(2-aminoethyl)amine, or poly(ethylenimine) grafted CNC materials (CNC-EDA, CNC-TRIS, and CNC-PEI, respectively) were evaluated for their ability to remediate pesticide solutions. These formulations were successfully synthesized and characterized by infrared spectroscopy and thermogravimetric analysis. An analytical protocol for analyzing pesticides of interest and assessing whether or not these new materials are capable of degrading pesticides was also developed and employed.
Materials and methods
General materials and methods
CNC slurry (12.2 wt% in H2O) was purchased from Cellulose Lab. Ethylenediamine (EDA), tris(2-aminoethyl)amine (Tris), poly(ethylenimine) solution (PEI) (1200–1300 Da, 50 wt% in H2O), 10–15% sodium hypochlorite solution (NaClO), sodium hydroxide, 2,2,6,6-tetramethylpiperidine 1-oxyl free radical (TEMPO), N-(3-dimethylaminopropyl)-N′-ethylcarbodiimide hydrochloride (EDC), and sodium bromide were purchased from Sigma-Aldrich and used without any further purification. Gas chromatography (GC) analyses were conducted using an Agilent 7890A gas chromatograph equipped with an Agilent G4513A autoinjector and a Flame Ionization Detector (FID). A Zebron ZB-MultiResidue-1 capillary GC column (30 m × 0.25 mm × 0.25 μm) was installed for pesticide detection. Agilent Technologies gas chromatography 1.5 mL volume vials with septum screw-caps were used in the analysis assays. Thermogravimetric analysis (TGA) was performed on a TA Instrument Hi-Res TGA 2950 analyzer. Analysis was conducted under nitrogen from 25 to 1000 °C using a 10 °C min−1 gradient. Fourier Transform Infrared (FTIR) analysis was performed with a Nicolet Magna 500 with NicPlan FT-IR Microscope and Mapping Stage.
Synthesis and characterization of polyamine-grafted cellulose nanocrystals45
Oxidation. A 20 g sample of a CNC slurry (12.2 wt% in H2O) was suspended in a water solution containing 0.20 g of TEMPO and 0.025 g of sodium bromide. A 4.77 g portion of a 12% aq. NaClO solution was added to the cellulose suspension in order to initiate the TEMPO-mediated oxidation. The reaction was performed at room temperature and stirred for 5 h. Additionally, a pH of 10 was maintained by addition of a 0.5 M NaOH solution. After 5 h, the solution was thoroughly washed with water by means of dialysis to remove any unreacted reagents. The pH of this purified suspension was then adjusted to a pH of 2.5 in order to neutralize the C-6 sodium carboxylates that resulted from the oxidation. The aqueous suspension was then washed thoroughly via dialysis, freeze-dried, and stored at −20 °C.
PEI-grafting. In order to achieve successful amine-functionalization, a 100 mg sample of the oxidized CNC was re-suspended in water (1% cellulose/water) in a 50 mL beaker. Next, 110 mg of EDC and the prescribed amine was added to the suspension. Specifically, ethylenediamine (40 μL, 0.590 mmol), tris(2-aminoethyl)amine (89 μL, 0.590 mmol), or poly(ethylenimine) (40 μL, 0.590 mmol) was added and allowed to stir for 6 h at room temperature. The modified cellulose nanocrystals were thoroughly washed with water by dialysis followed by freeze-drying and storage at −20 °C prior to full characterization and testing for pesticide remediation. The materials were characterized by TGA and FTIR45 and SEM (Fig. S1†). The surface area of the CNC-PEI material was measured by BET analysis (Fig. S16†).
Gas chromatographic pesticide remediation assay methods
GC analyses were carried out within the following parameters: inlet temperature: 170.0 °C; splitless injection at 15 mL min−1; column flow: 2.0143 mL min−1, constant pressure; carrier gas: helium; FID temperature: 340 °C; oven temperature program: 100 °C for 0.5 min, ramp to 180 °C at 20 °C min−1, hold for 4.5 min, final ramp to 240 °C at 6 °C min−1, hold for 14.5 min.
In order to assess the effectiveness of the amine-modified CNC materials we determined the standard pesticide peak area followed by the percent reduction of that area upon interaction with the amine-modified CNC material. In other words, the area of an untreated pesticide sample was compared to the area of treated pesticide samples. Control experiments were also necessary in order to ensure that pesticide remediation was not a result of adventitious degradation due to solvent, agitation, or time. In order to determine the standard pesticide area, before treatment with CNC-material, a 165 ppm solution of malathion pesticide in DCM was prepared. This solution was then added in 1 mL aliquots to 1.5 mL glass screw top GC vials. The vials were immediately subjected to GC analysis to determine the untreated pesticide peak area at t = 0 h. An additional set of vials were prepared, this time with 50 mg of the prescribed amine-functionalized CNC material and the 165 ppm malathion pesticide solution. The vials were then capped and sealed with Teflon tape followed by parafilm to avoid evaporation of the DCM solvent. These vials were then equipped with a stir bar and placed on a stir plate for 24 h prior to GC analysis. A final set of control vials were also analyzed that contained only a 165 ppm pesticide solution in DCM aged for 24 h. All experiments were conducted in triplicate. Pesticide remediation percentages could then be calculated by comparing the untreated pesticide peak area to the treated pesticide peak area. A calibration curve for malathion over the concentration range of 0.1 mM (33 ppm) to 1.5 mM (496 ppm) was generated (Fig. S17†).
CNC-PEI material loading experiment
To determine the optimal material loading for effective pesticide remediation, a series of GC vials were prepared containing a 165 ppm malathion solution in DCM as well as increasing amounts of CNC-PEI material. Thus, 1.5 mL glass screw-top GC vials were prepped with 10, 20, 30, 40, 50, 60, and 70 mg of the CNC-PEI material followed by 1 mL of a 165 ppm malathion solution in DCM. The vials were then capped and sealed with Teflon tape followed by parafilm to avoid evaporation of the DCM solvent. Control vials were also prepared consisting only of 1 mL of the 165 ppm solution of pesticide in DCM and analyzed at time 0 h as well as 24 h. Vials were then placed on an agitator plate for 24 h. Upon completion of the 24 h reaction time, the vial was subjected to GC analysis. All experiments were conducted in triplicate and pesticide degradation percentages were calculated. These results appear in Fig. S2.†
Agitation experiment
In order to determine whether or not agitation was required for pesticide remediation to occur, two experiments were conducted under otherwise identical conditions where one sample cohort was stirred using a stir bar and stir plate for the treatment period while the other sample cohort was allowed to stand on the benchtop. Untreated control samples were also prepared for analysis at time 0 h and 24 h. All trials were completed in triplicate. Upon GC analysis, it was concluded that agitation was necessary for optimal pesticide degradation to occur. These results appear in Fig. S3.†
Evaluation of CNC-PEI in aqueous systems
All commercially available pesticides were purchased from a local hardware store or over the internet. Pesticide solutions were prepared following the directions indicated on the respective packaging or used directly from the bottle as directed. More specifically, the Southern AG® malathion commercial pesticide was purchased over the internet in its 50% emulsifiable concentrate formulation. Therefore, this pesticide was prepared as a 2 tsp gal−1 aqueous solution as described on the label. While, the deltamethrin (Eliminator® Ant, Flea, and Tick Killer) and permethrin (Eliminator® Home Insect Killer) samples were purchased from a local hardware store as their ready-to-use formulations, with 2.5% and 0.02% active pesticide ingredient, respectively.
Pure samples of each pesticide sample were also prepared by making a solution of 10 mg of pesticide per 1 mL of water. Due to the poor aqueous solubility of most pesticides, these solutions were allowed to sonicate for approximately 20 min and then analyzed by means of GC. These analytical pesticide standards were prepared to facilitate comparison to the commercial pesticide formulation that also contained a number of additives along with the active pesticide ingredient. Once the retention times of each pesticide was determined, the remediation experiments could be completed. GC vials were prepared following the previously described method: control untreated vials (t = 0 h and t = 24 h) contained only 1 mL of the pesticide solution while treated samples contained 1 mL of the pesticide solution and 50 mg CNC-PEI. All experiments were conducted in triplicate.
GC-MS methods for pesticide degradation by-product detection
Pesticide samples containing degradation by-products were evaluated using a Shimadzu GC-2010 Plus, equipped with a AOC-20i auto injector, coupled to a QP2010 SE mass spectrometer. A Shimadzu GC SH-Rxi-5ms capillary column (15 m × 0.25 mm × 0.25 μm) and a Shimadzu SH-RXI-5SIL MS column (30 m × 0.25 mm × 0.25 μm) were installed for product detection. GC analyses were carried out within the following parameters: inlet temperature: 50.0 °C; split injection (4 μL) at 14.1 mL min−1; column flow: 1.0 mL min−1, constant pressure; carrier gas: helium; temperature program: 40 °C for 3 min; ramp to 320 °C at 20 °C min−1, hold for 10 min. MS analyses were conducted as follows: ion source temperature: 200 °C; interface temperature: 100 °C; solvent cut time: 3 min. Chromatograms and mass spectra from these analyses, along with a descriptive narrative, appear in the ESI.†
CNC-PEI re-use experiments
To demonstrate the reusability of the amine-modified CNC material, previously used CNC-PEI was filtered from the pesticide solution and washed five times with 10 mL aliquots of deionized H2O in order to remove any pesticide contaminants and by-products. After each wash, the filtrate was injected into the GC to monitor the presence of any remaining pesticide or pesticide by-products. The washed material was then allowed to air dry followed by further drying in vacuo. The dried CNC-PEI was then subjected to FTIR and TGA analysis to monitor any potential changes to the used material. No signs of complete PEI defunctionalization were detected (Fig. S12 and S13†). The CNC-PEI was then evaluated for malathion degradation via GC following the above described protocol. After GC evaluation, the used CNC-PEI was again recovered via filtration, wash, and drying cycles. The morphology of the CNC-PEI material after washing was compared to pristine CNC-PEI material by SEM analysis, revealing no apparent morphological differences before and after washing (Fig. S15†).
Results and discussion
Synthesis and characterization of amine-modified CNC materials
We prepared CNC-PEI, CNC-EDA, and CNC-TRIS samples following our previously described amine-functionalization protocol.45 Briefly, commercially available CNC was oxidized via an aqueous TEMPO-mediated process wherein the primary, C6-alcohols of the CNC material were selectively oxidized to their corresponding sodium carboxylates. Following successful oxidation, the material was treated with an ion-exchange solution in order to convert the C6 sodium carboxylates to their neutral counterparts. Finally, CNC-EDA, CNC-TRIS, and CNC-PEI were obtained after carbodiimide coupling with EDA, TRIS, or PEI, respectively (Fig. 1).
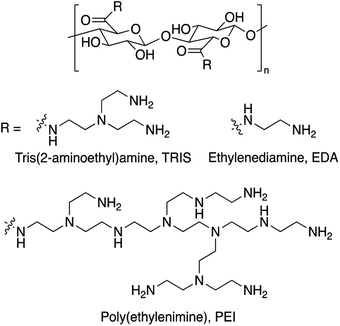 |
| Fig. 1 Amine-modified cellulose nanocrystal structures. | |
The amine-modified CNC materials were characterized by Fourier-transform infrared spectroscopy (FTIR) and thermogravimetric analysis (TGA). Emergence of N–H bending modes were easily followed throughout the synthetic sequence by FTIR (Fig. 2A). Following TEMPO oxidation, the new C-6 carbonyl produces an identifiable C
O stretch at 1712 cm−1 that is not present in the unmodified CNC starting material. Furthermore, upon amine (i.e. EDA, TRIS, or PEI) functionalization this carbonyl stretch is mostly obscured by the emergence of N–H bending bands around 1585 cm−1, suggesting successful amide bond formation following EDC coupling of the C6 carboxylate of the oxidized CNC and the prescribed amine. It is important to note the absence of characteristic amine N–H sp3 stretches in the amine-modified CNC materials which are coincident with the OH stretches from the CNC core of the material.
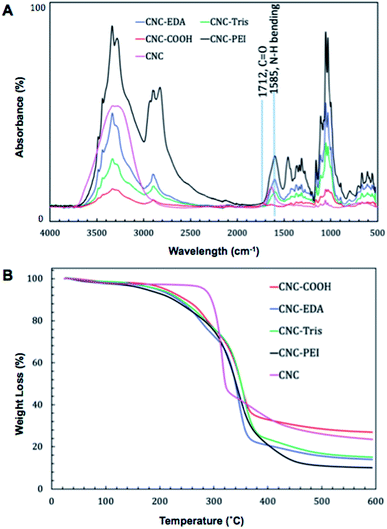 |
| Fig. 2 (A) Infrared spectra of amine-modified CNC. CNC-COOH (red line), CNC-TRIS (green line), CNC-EDA (blue line) and CNC-PEI (black line). Successful oxidation is indicated by C O carbonyl stretching at 1712 cm−1 as seen in CNC-COOH, and amine modification is indicated by emergence of N–H bending modes at 1585 cm−1. (B) TGA analysis of CNC (pink line), CNC-COOH (red line), CNC-TRIS (green line), CNC-EDA (blue line) and CNC-PEI (black line). | |
Thermogravimetric analysis of the amine-modified materials resulted in similar degradation profiles that each differ in comparison to the oxidized CNC precursor prior to amine coupling, lending further support of successful amine-modification (Fig. 2B). The morphology of the material before and after modification was evaluated by SEM and TEM (Fig. S1†). The surface area of the CNC-PEI material was also evaluated using BET analysis (Fig. S16†).
Evaluation of CNC-PEI in organic systems for pesticide remediation
Currently, the most commonly utilized pesticides belong to the organophosphorus (OP) class of agrochemicals. Malathion (i.e. O,O-dimethyl-(S)-(1,2-dicarbethoxy)ethyl phophorodithioate) is one example that is primarily used as an insecticide to control mosquito and fruit fly populations. Malathion also serves as a viable alternative to the traditionally used organochlorine (OC) compounds, such as DDT, lindane, and endosulfan.48 These OC pesticides are notorious for their persistent and bioaccumulative nature, which resulted in the release of persistent pesticide residues into natural water supplies. While OP pesticides do serve as a less harmful alternative to chlorinated pesticides they still represent a major environmental concern, especially in the context of water contamination.7,49–51
We sought to implement amine-modified CNCs as a material to facilitate pesticide degradation. In order to easily assess and compare the remediation efficiency of each amine-CNC material, a GC analysis protocol was first optimized using solutions of DCM spiked with malathion. OP pesticides tend to be poorly water soluble, therefore, these initial analyses allowed for a higher degree of OP pesticide solubility as well as clean chromatograms for analysis. According to the United States Environmental Protection Agency (US EPA) and Food and Drug Administration (FDA), food crops are allowed to have residual amounts of malathion at a maximum concentration of 8 ppm.
Additionally, the minimal risk level (MRL) for inhalation (0.2 ppm per <14 days) and oral exposure (0.02 ppm per day) of malathion are set at much smaller concentrations. Thus, a solution of 165 ppm malathion in DCM was used in the remediation experiments to ensure the concentration was above these levels and able to provide reliable GC chromatogram results. The loading of CNC-PEI was first optimized. Briefly, 1.5 mL glass screw-top GC vials were charged with 10, 20, 30, 40, 50, 60, and 70 mg of the CNC-PEI material followed by a 1 mL aliquot of a solution of 165 ppm malathion in DCM. The vials were then capped and sealed with Teflon tape followed by paraffin wax film to avoid evaporation of the DCM solvent. Control vials were also prepared containing 1 mL of the 165 ppm solution of pesticide in DCM and analyzed at time 0 h as well as 24 h. The vials were then placed on an orbital shaker for 24 h. Following treatment with CNC-PEI for 24 h, the vials were subjected to GC analysis. All experiments were conducted in triplicate and pesticide degradation percentages were calculated. Following the CNC-PEI loading experiments, it was determined that 50 mg of the material was sufficient to completely degrade a 165 ppm solution of malathion in DCM. An increase in remediation is noticed when increasing the material loading from 10 mg to 50 mg of CNC-PEI, achieving 75.6 ± 0.8% and 100% remediation of malathion, respectively (Fig. S1 in ESI†). Using the optimized 50 mg CNC-PEI loading, it was also concluded that agitation was necessary for complete degradation to occur since only 74% degradation was observed without agitation as compared to 100% degradation with agitation of the CNC-PEI/malathion solution (see ESI† for details). Agitation was required for optimal results (100% remediation) due to the CNC-PEI particles slowly settling to the bottom of the vial over time.
All three amine-modified materials were assessed for their ability to degrade malathion. A 50 mg loading of CNC-EDA, CNC-TRIS, and CNC-PEI resulted in 59 ± 2%, 50 ± 5%, and 100% remediation of malathion, respectively (Fig. 3). The superior performance of the CNC-PEI material is likely due to the presence of additional reactive sites on the surface of the CNC as compared to the mono- and di-functional CNC-EDA and CNC-TRIS materials, respectively. It is suspected that these amine derivatizing agents have the capability to degrade malathion to some degree on their own based on the confirmed degradation pathway and by-products. However, grafting the amine compounds on the solid CNC provides several advantages. These include permitting the use of smaller amounts of the amine compounds, the ability to re-use the sample, and the capability of using it as a solid or in solution. A sample of unmodified CNC was tested as a control in order to demonstrate the necessity of the amine functionalization step for effective remediation. In this case, treatment of malathion with unmodified CNCs resulted in quantitative recovery of malathion after 24 h. Finally, we generated a calibration curve for malathion over the concentration range of 0.1 mM (33 ppm) to 1.5 mM (496 ppm), and we found that CNC-PEI was capable of greater than 98% remediation at both the high and low ends of the curve (Fig. S17†).
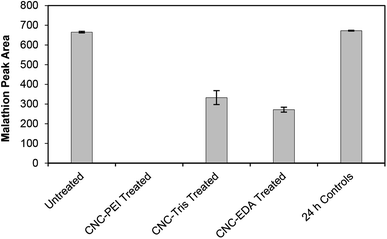 |
| Fig. 3 Pesticide remediation assays with CNC-PEI, CNC-TRIS, and CNC-EDA. Untreated samples contain only pesticide solution and serve as controls at t = 0 h and 24 h. | |
Evaluation of CNC-PEI in aqueous systems
Next, we sought to investigate the efficiency of the CNC-PEI material in aqueous medium (Fig. 4). Many insecticides are typically utilized as a liquid or spray that employ water as the carrier.52 Therefore, various commercially available pesticide formulations of malathion, permethrin, and deltamethrin were employed. These commercial formulations are widely available at local hardware stores or from internet vendors. It is important to note that many commercial pesticide solutions are formulated as a mixture of the active ingredient and other components. Since the commercially available formulations contain a number of other additives in addition to the pesticide of interest, calibration curves for the aqueous formulations could not be generated. Pure standards of each pesticide were used to identify the peaks of interest in the rather complex commercially available solutions. Each commercially available pesticide solution was prepared as instructed on the packaging prior to treatment with the CNC-PEI materials and analysis. As previously described, control GC vials containing only pesticide solution as well as treated vials containing the pesticide formulation and 50 mg CNC-PEI were prepared. All experiments were conducted in triplicate with agitation for 24 h. Treatment of the commercially available malathion solution with 50 mg CNC-PEI resulted in 100% degradation of the OP pesticide at ambient temperature. Similarly, treatment of a commercially available deltamethrin solution with CNC-PEI resulted in a 95 ± 0.9% reduction at ambient temperature. The analysis of the commercial permethrin preparation was complicated by the fact that the active ingredient is present as a pair of detectable cis/trans stereoisomers about the central cyclopropane ring. Nevertheless, upon treatment with CNC-PEI samples at a slightly elevated temperature of 35 °C, the two peaks exhibited an average of 84.3 ± 0.7% and 78 ± 2% reduction, respectively (Fig. 4C). One of the advantages of using CNC-PEI for degradation in aqueous environments is that it does not require the use of acidic or basic solutions unlike common hydrolysis pathways.
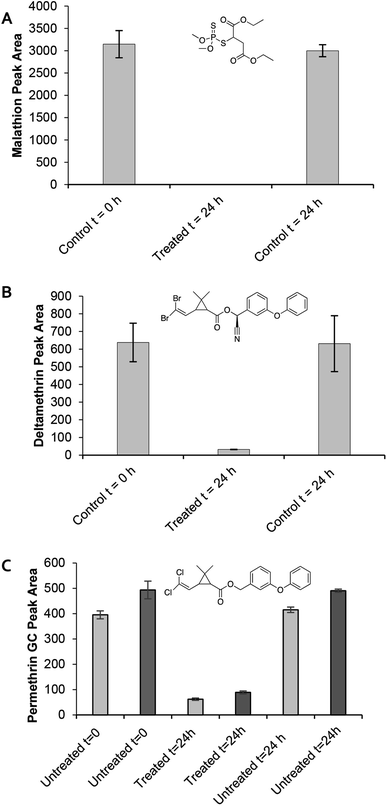 |
| Fig. 4 (A) Degradation of malathion, (B) deltamethrin, and (C) permethrin with CNC-PEI. Untreated samples contain only pesticide solution and serve as controls at t = 0 h and t = 24 h. Malathion and deltamethrin were conducted at RT while permethrin was conducted at 35 °C. | |
Exploring the mechanism of malathion degradation
Next, in order to probe the mechanism of degradation, we sought to identify the by-products resulting from the interaction of malathion and CNC-PEI. Thus, a 165 ppm solution of malathion was prepared in deionized water, and treated with 50 mg of CNC-PEI followed by agitation for 24 h. The CNC-PEI material was then removed from the reaction mixture by filtration, and the filtrate was then analyzed via GC-MS in order to identify the degradation products resulting from treatment. Two main degradation products, O,O-dimethyl phosphorodithioic acid (1, DMTP) and diethyl fumarate (2), were identified by GC-MS analysis. These compounds have previously been reported as commonly observed hydrolysis degradation by-products of malathion.53–55 We posit that, in our system, these products arise from a facile elimination of one of the ester α-protons from malathion – induced by CNC-PEI – to generate DMTP and diethyl fumarate by means of either an E2 or E1CB mechanism (Scheme 1). Although we did not evaluate the effects of pH on the ability of CNC-PEI to degrade malathion, based on the proposed mechanism outlined in Scheme 1, one would expect that increasing the pH would accelerate the rate of degradation by reducing the level of protonation of the CNC-PEI material. Conversely, lowering the pH would likely result in increased levels of protonation of the CNC-PEI material, retarding the rate of the β-elimination. The diethyl fumarate degradation product exhibited a mass spectrum that was consistent with data published in the National Institute of Standards and Technology (NIST) library. The DMTP mass spectrum exhibits a fragmentation pattern (i.e. loss of S–H, m/z = 125) that is easily assigned to by-product 1.56 In a previous study, Bavcon et al. monitored the degradation of malathion in untreated water, where only 30% of an initial 10.3 ppm malathion concentration was degraded over 14 days.57 Consistent with this previous observation of slow degradation of malathion in untreated water, control GC-MS experiments with untreated aqueous malathion samples revealed no detectable degradation over 24 h. Additionally, maloxon, a commonly observed toxic oxidation by-product (which is the P
O congener of malathion, was not detected via GC-MS) (see ESI† for full GC-MS details).57,58
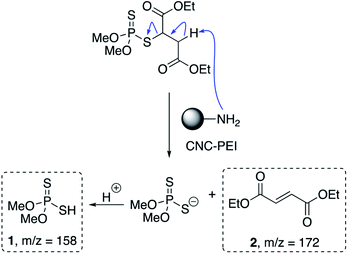 |
| Scheme 1 Proposed degradation pathway of malathion upon treatment with CNC-PEI. | |
Deltamethrin degradation
Next, we also investigated the degradation products of deltamethrin after treatment with CNC-PEI. Upon GC-MS analysis, three lower molecular weight by-products were identified (Scheme 2). Deltamethrin by-product 3 (m/z = 429) is presumably the result of cleavage of the diaryl ether moiety. Compounds 4B (m/z = 281) and 5B (m/z = 209) are likely by-products of ester cleavage. More specifically, compound 4B is the acylium ion of deltamethrinic acid (4A) while 5B is the counterpart to α-hydroxy-3-phenoxy-benzeneacetonitrile (5A). Deltamethrinic acid, 4A, and α-hydroxy-3-phenoxy-benzeneacetonitrile, 5A, have previously been reported in the literature as major hydrolysis degradation by-products of deltamethrin in aquatic environments.59–61 Further, the mass spectrum of 5A aligns well with that of the neutral 3-phenoxyphenylacetonitrile on the NIST database.62 An additional by-product was detected via GC-MS; however, its identity is unclear. Further investigations are being conducted in order to elucidate the structure and clarify the degradation pathway of deltamethrin in the presence of CNC-PEI (see ESI† for full details).
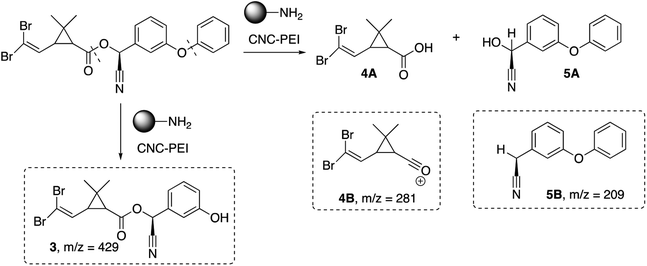 |
| Scheme 2 Proposed degradation pathway of deltamethrin upon treatment with CNC-PEI. | |
CNC-PEI reuse experiment
Next, we explored the reusability of the material for pesticide remediation by resubjecting used CNC-PEI samples to further treatment with additional aliquots of pesticide-contaminated water (Fig. 5). Thus, spent CNC-PEI samples were filtered from the pesticide solution and washed with 10 mL aliquots of deionized water until no pesticide contaminants or degradation products were detected upon GC analysis. The washed material was then allowed to air dry for 24 h. The dried CNC-PEI was then subjected to FTIR and TGA analyses to monitor any potential changes to the used material. No signs of drastic PEI loss were detected following the first wash cycle (Fig. S13 and S14†). The morphology of the washed material was also monitored by SEM analysis, revealing no appreciable difference in the materials before and after washing (Fig. S15†). The CNC-PEI was then resubjected to fresh samples of malathion contaminated water and the degree of malathion degradation was analyzed by GC. A previously used and washed sample of CNC-PEI resulted in 98 ± 4% degradation following the first reuse cycle (Fig. 5). Following a subsequent reuse cycle, however, only 50 ± 13% malathion degradation was achieved. It is clear that the effectiveness of the CNC-PEI material decreases as the number of wash and reuse cycles increases. For example, the 3rd reuse experiment resulted in no detectable malathion degradation.
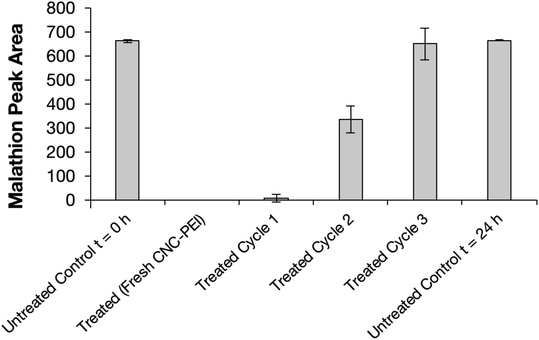 |
| Fig. 5 CNC-PEI reuse experiments. Complete loss of function is observed at reuse cycle 3. | |
CNC-PEI malathion degradation time study
Finally, we sought to evaluate the time course of the degradation of malathion to understand how rapidly the degradation event occurs. Thus the degradation efficiency of CNC-PEI was monitored after 10, 20, and 30 min of treatment time (Fig. 6). We found that malathion was degraded by ∼63% after 10 min, 94% after 20 min, and greater the 99% after 30 min of treatment (Fig. 6A). A plot of ln[malathion] versus time returned a line with slope = −kobs, indicating that the degradation event follows pseudo-1st order kinetics (kobs = 0.17 min−1). This observation is consistent with the proposed elimination described above (see Scheme 1). From the expression, t1/2 = ln
2/k, the half-life of the degradation of malathion by CNC-PEI was calculated to be 4.1 min. These results confirm that CNC-PEI is capable of rapidly degrading relevant concentrations of malathion in water, with near complete degradation in a matter of minutes.
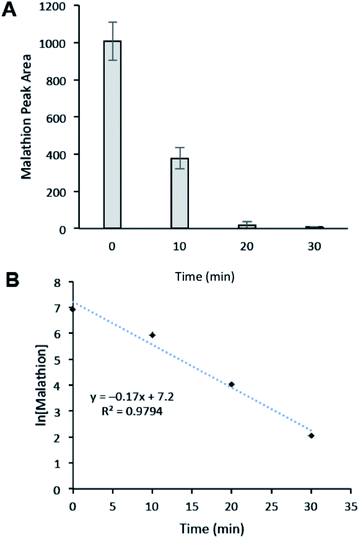 |
| Fig. 6 (A) Time course study of malathion degradation by CNC-PEI. (B) First order kinetic analysis: plot of ln[malathion] vs. time. | |
Conclusions
We have described the successful degradation of various pesticides in aqueous media using amine modified cellulose nanocrystals. CNC-PEI was able to effect 100%, 95%, and 78% degradation of malathion, deltamethrin, and permethrin, respectively. The transformation occurs, in the case of malathion, on the order of minutes. These amine-modified CNC materials take advantage of a renewable, biodegradable, and biocompatible polymer as well as an amine moiety that has potential for chemical degradation of various pesticides. We explored the mechanism by which the degradation process occurs by evaluating the degradation products by GC-MS after treatment. This study demonstrates that amine-modified cellulose nanocrystals may be a cheap and useful material for the remediation of pesticide contaminants in water. Future studies with these materials will focus on exploring the lower concentration limits of pesticide-contaminated water as well as expanding our study of target pesticides to include other important compound classes such as the neonicotinoids.
Conflicts of interest
The authors declare no competing financial interests.
Acknowledgements
The authors gratefully acknowledge the Fats & Proteins Research Foundation, Inc., the Poultry Protein and Fat Council, the Clemson University Animal Co-Products Research and Education Center, and the Clemson University Research Foundation for financial support. The authors would also like to thank Ms Kim Ivey for technical assistance throughout the CNC material characterization. The authors thank Dr Kristi Whitehead for assistance with formatting figures. The authors thank Dr Fernanda Guerra for collecting BET data.
References
- S. Malato, J. Blanco, M. I. Maldonado, I. Oller, W. Gernjak and L. Perez-Estrada, Coupling solar photo-Fenton and biotreatment at industrial scale: main results of a demonstration plant, J. Hazard. Mater., 2007, 146, 440–446 Search PubMed.
- A. Zapata, S. Malato, J. A. Sanchez-Perez, I. Oller and M. I. Maldonado, Scale-up strategy for a combined solar photo-Fenton/biological system for remediation of pesticide-contaminated water, Catal. Today, 2010, 151, 100–106 Search PubMed.
- S. M. Rhind, Endocrine disrupting compounds and farm animals: their properties, actions and routes of exposure, Domest. Anim. Endocrinol., 2002, 23, 179–187 Search PubMed.
- I. Cerillo, M. F. Olea-Serrano, J. Ibarluzea, J. Exposito, P. Torne, J. Laguna, V. Pedraza and N. Olea, Environmental and lifestyle factors for organochlorine exposure among women living in Southern Spain, Chemosphere, 2006, 62, 1917–1924 Search PubMed.
- The WHO Recommended Classification of Pesticides by Hazard and Guidelines to Classification Citation: International Program on Chemical Safety. World Health Organization, WHO Press, World Health Organization, Geneva, Switzerland, 2009 Search PubMed.
- D. W. Kolpin, E. M. Thurman and D. A. Goolsby, Occurrence of selected pesticides and their metabolites in near-surface aquifers of the mid-Western United States, Environ. Sci. Technol., 1996, 30, 335–340 Search PubMed.
- W. G. Aker, X. Hu, P. Wang and H. M. Hwang, Comparing the relative toxicity of malathion and malaoxon in blue catfish Ictalurus furcatus, Environ. Toxicol., 2008, 23, 548–554 Search PubMed.
- J. M. Herrmann and C. Guillard, Photocatalytic degradation of pesticides in agricultural used waters, C. R. Acad. Sci., 2000, 3, 417–422 Search PubMed.
- F. J. Benitez, J. L. Acero, T. Gonzalez and J. Garcia, Organic matter removal from wastewaters with manure, household waste or sewage sludge, Process Biochem., 2001, 37, 257–265 Search PubMed.
- R. J. Miltner, D. B. Baker, T. F. Speth and C. A. Fronk, Treatment of Seasonal Pesticides in Surface Waters, J. - Am. Water Works Assoc., 1989, 81, 43–52 Search PubMed.
- S. A. A. Firozjaei, A. M. Latifi, S. Khodi, S. Abolmaali and A. Choopani, A Review on Biodegradation of Toxic Organophosphate Compounds, J. Appl. Biotechnol. Rep., 2015, 2, 215–224 Search PubMed.
- D. Rawtani, N. Khatri, S. Tyagi and G. Pandey, Nanotechnology-based recent approaches for sensing and remediation of pesticides, J. Environ. Manage., 2018, 206, 749–762 Search PubMed.
- E. M. John and J. M. Shaike, Chlorpyriphos: pollution and remediation, Environ. Chem. Lett., 2015, 13, 269–291 Search PubMed.
- M. Rani, U. Shanker and V. Jassal, Recent strategies for removal and degradation of persistent & toxic organochlorine pesticides using nanoparticles: a review, J. Environ. Manage., 2017, 190, 208–222 Search PubMed.
- G. Durand, J. L. Abad, F. Sanchez-Baeza, A. Messeguer and D. Barcelo, Unequivocal identification of compounds formed in the photodegradation of fenitrothion in water/methanol and proposal of selected transformation pathways, J. Agric. Food Chem., 1994, 42, 814–821 Search PubMed.
- Z. Mengyue, C. Shifu and T. Yaowu, Photocatalytic degradation of organophosphorus pesticides using thin film of TiO2, J. Chem. Technol. Biotechnol., 1995, 64, 339–344 Search PubMed.
- R. Doong and W. Chang, Photoassisted titanium dioxide mediated degradation of organophosphorus pesticides by hydrogen peroxide, J. Photochem. Photobiol., A, 1997, 107, 239–244 Search PubMed.
- G. L. Beyke, Thermally enhanced hydrolysis for treatment of pesticides and explosives, Remediation, 2018, 28, 17–22 Search PubMed.
- S. Chiron, A. Fernandez-Alba, A. Rodriguez and E. Garcia-Calvo, Pesticide chemical oxidation: state of the art, Water Res., 2000, 34, 366–377 Search PubMed.
- L. A. Pérez-Estrada, S. Malato, W. Gernjak, A. Aguëra, E. M. Thurman, I. Ferrer and A. R. Fernández-Alba, Photo-Fenton degradation of diclofenac: identification of main intermediates and degradation pathway, Environ. Sci. Technol., 2005, 39, 8300–8306 Search PubMed.
- M. S. Lucas and J. A. Peres, Decolorization of the azo dye Reactive Black 5 by Fenton and photo-Fenton oxidation, Dyes Pigm., 2006, 71, 236–244 Search PubMed.
- C. Comninellis, A. Kapalka, S. Malato, S. A. Parsons, I. Poulios and D. Mantzavinos, Advanced oxidation processes for water treatment: advances and trends for R&D, J. Chem. Technol. Biotechnol., 2008, 83, 769–776 Search PubMed.
- C. L. Mangun, Z. Yue, J. Economy, S. Maloney, P. Kemme and D. Cropek, Adsorption of organic contaminants from water using tailored ACFs, Chem. Mater., 2001, 13, 2356–2360 Search PubMed.
- G. V. Lowry and K. M. Johnson, Congener-specific dechlorination of dissolved PCBs by microscale and nanoscale zerovalent iron in a water/methanol solution, Environ. Sci. Technol., 2004, 38, 5208–5216 Search PubMed.
- A. Acevedo, E. A. Carpio, J. Rodriguez and M. A. Manzano, Disinfection of natural water by solar photocatalysis using immobilized TiO2 devices: efficiency in eliminating indicator bacteria and operating life of the system, J. Sol. Energy Eng., 2012, 134, 011008 Search PubMed.
- G. Ghasemzadeh, M. Momenpour, F. Omidi, M. R. Hosseini, M. Ahani and A. Barzegari, Applications of nanomaterials in water treatment and environmental remediation, Front. Environ. Sci. Eng., 2014, 8, 471–482 Search PubMed.
- H. A. Wayland, S. N. Boury, B. P. Chhetri, A. Brandt, M. A. Proskurnin, V. A. Filichkina, V. P. Zharov, A. S. Biris and A. Ghosh, Advanced Cellulosic Materials for Treatment and Detection of Industrial Contaminants in Wastewater, ChemistrySelect, 2016, 1, 4472–4488 Search PubMed.
- F. D. Guerra, M. F. Attia, D. C. Whitehead and F. Alexia, Nanotechnology for environmental remediation: materials and applications, Molecules, 2018, 23, 1760 Search PubMed.
- A. G. Varghese, S. A. Paul and M. S. Latha, Remediation of heavy metals and dyes from wastewater using cellulose-based adsorbents, Environ. Chem. Lett., 2019, 17, 867–877 Search PubMed.
- D. Wang, A critical review of cellulose-based nanomaterials for water purification in industrial processes, Cellulose, 2019, 26, 687–701 Search PubMed.
- H. Voisin, L. Bergström and A. P. Mathew, Nanocellulose-based materials for water purification, Nanomaterials, 2017, 7, 57 Search PubMed.
- N. Mahfoudhi and S. Boufi, Nanocellulose as a novel nanostructured adsorbent for environmental remediation: a review, Cellulose, 2017, 24, 1171–1197 Search PubMed.
- L. Melone, B. Rossi, N. Pastori, W. Panzeri, A. Mele and C. Punta, TEMPO-oxidized cellulose cross-linked with branched polyethyleneimine: nanostructured adsorbent sponges for water remediation, ChemPlusChem, 2015, 80, 1408–1415 Search PubMed.
- L. Fan, Y. Lu, L.-Y. Yang, F. Huang and X.-K. Ouyang, Fabrication of polyethylenimine-functionalized sodium alginate/cellulose nanocrystal/polyvinyl alcohol core-shell microspheres ((PVA/SA/CNC)@PEI) for diclofenac sodium adsorption, J. Colloid Interface Sci., 2019, 554, 48–58 Search PubMed.
- L. Jin, W. Li, Q. Xu and Q. Sun, Amino-functionalized nanocrystalline cellulose as an adsorbent for anionic dyes, Cellulose, 2015, 22, 2443–2456 Search PubMed.
- C. Tang, P. Brodie, Y. Li, N. J. Grishewich, M. Brunsting and K. C. Tam, Shape recoverable and mechanically robust cellulose aerogel beads for efficient removal of copper ions, Chem. Eng. J., 2020, 392, 124821 Search PubMed.
- J. Li, W. Zuo, W. Wu, Y. Yi, Y. Jing, H. Dai and G. Fang, Shape memory aerogels from nanocellulose and polyethyleneimine as a novel adsorbent for removal of Cu(II) and Pb(II), Carbohydr. Polym., 2018, 196, 376–384 Search PubMed.
- A. Pei, N. Butchosa, L. A. Berglund and Q. Zhou, Surface quaternized cellulose nanofibrils with high water absorbency and adsorption capacity for anionic dyes, Soft Matter, 2013, 9, 2047–2055 Search PubMed.
- L. Jin, Q. Sun, Q. Xu and Y. Xu, Adsorptive removal of anionic dyes from aqueous solutions using microgel based on nanocellulose and polyvinylamine, Bioresour. Technol., 2015, 197, 348–355 Search PubMed.
- W. Zhu, L. Liu, Q. Liao, X. Chen, Z. Qian, J. Shen, J. Liang and J. Yao, Functionalization of cellulose with hyperbranched polyethylenimine for selective dye adsorption and separation, Cellulose, 2016, 23, 3785–3797 Search PubMed.
- C. Xi, R. Wang, P. Rao, W. Zhang, G. Li, F. Chai, Y. Cai, T. Luo and X. Zhou, The fabrication and arsenic removal performance of cellulose-nanocrystal-containing adsorbents based on the “bridge joint” effect of iron ions, Carbohydr. Polym., 2020, 237, 116129 Search PubMed.
- N. Zhang, G.-L. Zang, C. Shi, H.-Q. Yu and G.-P. Sheng, A novel adsorbent TEMPO-mediated oxidized cellulose nanofibrils modified with PEI: preparation, characterization, and application for Cu(II) removal, J. Hazard. Mater., 2016, 316, 11–18 Search PubMed.
- M. L. Campbell, F. D. Guerra, J. Dhulekar, F. Alexis and D. C. Whitehead, Target-Specific Capture of Environmentally Relevant Gaseous Aldehydes and Carboxylic Acids with Functional Nanoparticles, Chem.–Eur. J., 2015, 21, 14834–14842 Search PubMed.
- F. D. Guerra, M. L. Campbell, D. C. Whitehead and F. Alexis, Tunable properties of functional nanoparticles for efficient capture of VOCs, ChemistrySelect, 2017, 2, 9889–9894 Search PubMed.
- F. D. Guerra, M. L. Campbell, M. F. Attia, D. C. Whitehead and F. Alexis, Capture of aldehyde VOCs using a series of amine-functionalized cellulose nanocrystals, ChemistrySelect, 2018, 3, 5485–5501 Search PubMed.
- M. I. Swasy, M. L. Campbell, B. R. Brummel, F. D. Guerra, M. F. Attia, G. D. Smith, F. Alexis and D. C. Whitehead, Poly(amine) modified kaolinite clay for VOC capture, Chemosphere, 2018, 213, 19–24 Search PubMed.
- M. Ateia, M. F. Attia, A. Maroli, N. Tharayil, F. Alexis, D. C. Whitehead and T. Karanfil, Rapid Removal of Poly- and Perfluorinated Alkyl Substances by Polyethylenimine-functionalized Cellulose Microcrystals at Environmentally Relevant Conditions, Environ. Sci. Technol. Lett., 2018, 5, 764–769 Search PubMed.
- W. H. Chambers, Organophosphorous compounds: an overview, in Oranophosphates, Chemistry, Fate, and Effects, ed. J. E. Chambers and P. E. Levi, Academic Press, San Diego, 1992, pp. 3–17 Search PubMed.
- L. Karalliedde and N. Senanayake, Organophosphorus insecticide poisoning, J. Int. Fed. Clin. Chem., 1999, 11, 4–9 Search PubMed.
- M. D. LaGrega, Hazardous waste management. Buckingham PL, Evans JC Hazardous waste management, McGraw-Hill, New York, 2nd edn, 2001 Search PubMed.
- F. M. Raushel, Chemical biology: Catalytic detoxification, Nature, 2011, 469, 310–311 Search PubMed.
- M. Sarwar, Commonly Available Commercial Insecticide Formulations and Their Applications in the Field, Inter. J. Mater. Chem. Phys., 2015, 1, 116–123 Search PubMed.
- K. D. Racke, Degradation of organophosphorus insecticides in environmental matrices. in Organophosphates, Chemistry, Fate, and Effects, ed. J. E. Chambers and P. E. Levi, Academic Press, San Diego, 1992, pp. 47–73 Search PubMed.
- S. O. Pehkonen and Q. Zhang, The degradation of organophosphorus pesticides in natural waters: a critical review, Crit. Rev. Environ. Sci. Technol., 2002, 32, 17–72 Search PubMed.
- X. Zhao and H. M. Hwang, A study of the degradation of organophosphorus pesticides in
river waters and the identification of their degradation products by chromatography coupled with mass spectrometry, Arch. Environ. Contam. Toxicol., 2009, 56, 646–653 Search PubMed.
- NIST Chemistry Web Book, https://webbook.nist.gov/cgi/cbook.cgi?ID=C623916%26Mask=200#Mass-Spec, accessed Jan. 10, 2019 Search PubMed.
- M. Bavcon, P. Trebše and L. Zupančič-Kralj, Investigations of the determination and transformations of diazinon and malathion under environmental conditions using gas chromatography coupled with a flame ionisation detector, Chemosphere, 2003, 50, 595–601 Search PubMed.
- M. A. Borwn, M. X. Petreas, H. S. Okamoto, T. Mischeke and R. D. Stephens, Monitoring of malathion and its impurities and environmental transformation products on surfaces and in air following an aerial application, Environ. Sci. Technol., 1993, 27, 388–397 Search PubMed.
- D. C. G. Muir, G. P. Rawn and N. P. Grift, Fate of the pyrethroid insecticide deltamethrin in small ponds: a mass balance study, J. Agric. Food Chem., 1985, 33, 603–609 Search PubMed.
- K. M. Erstfeld, Environmental fate of synthetic pyrethroids during spray drift and field runoff treatments in aquatic microcosms, Chemosphere, 1999, 39, 1737–1769 Search PubMed.
- S. Chen, K. Lai, Y. Li, M. Hu, Y. Zhang and Y. Zeng, Biodegradation of deltamethrin and its hydrolysis product 3-phenoxybenzaldehyde by a newly isolated Streptomyces aureus strain HP-S-01, Appl. Microbiol. Biotechnol., 2011, 90, 1471–1483 Search PubMed.
- NIST Chemistry Web Book, https://webbook.nist.gov/cgi/cbook.cgi?Name=3-Phenoxyphenylacetonitrile+%26Units=SI%26cMS=on#Mass-Spec, accessed March. 15, 2019 Search PubMed.
Footnote |
† Electronic supplementary information (ESI) available: Accompanying data for experimental details for preparation of the amine-CNC materials, assay against pesticides, GC-MS analysis, and recycling experiments. See DOI: 10.1039/d0ra08308a |
|
This journal is © The Royal Society of Chemistry 2020 |
Click here to see how this site uses Cookies. View our privacy policy here.