DOI:
10.1039/D0SC01172J
(Edge Article)
Chem. Sci., 2020,
11, 5182-5190
Structure elucidation of the redox cofactor mycofactocin reveals oligo-glycosylation by MftF†
Received
26th February 2020
, Accepted 18th April 2020
First published on 23rd April 2020
Abstract
Mycofactocin (MFT) is a redox cofactor belonging to the family of ribosomally synthesized and post-translationally modified peptides (RiPPs) and is involved in alcohol metabolism of mycobacteria including Mycobacterium tuberculosis. A preliminary biosynthetic model had been established by bioinformatics and in vitro studies, while the structure of natural MFT and key biosynthetic steps remained elusive. Here, we report the discovery of glycosylated MFT by 13C-labeling metabolomics and establish a model of its biosynthesis in Mycolicibacterium smegmatis. Extensive structure elucidation including NMR revealed that MFT is decorated with up to nine β-1,4-linked glucose residues including 2-O-methylglucose. Dissection of biosynthetic genes demonstrated that the oligoglycosylation is catalyzed by the glycosyltransferase MftF. Furthermore, we confirm the redox cofactor function of glycosylated MFTs by activity-based metabolic profiling using the carveol dehydrogenase LimC and show that the MFT pool expands during cultivation on ethanol. Our results will guide future studies into the biochemical functions and physiological roles of MFT in bacteria.
Introduction
Coenzymes are small molecules indispensable for the catalytic activity of many enzymes. While coenzymes like NAD+ or FAD are ubiquitous in nature and are essential for the core metabolism of all forms of life, specialized cofactors like pyrroloquinoline quinone (PQQ)1 and coenzyme F420
2 are restricted to certain microbial phyla, but typically involved in extraordinary metabolic processes like methylotrophy, methanogenesis, or detoxification processes. Moreover, specialized cofactors serve as model systems for the evolution of cofactors and their co-evolution with their associated enzyme families. They can be regarded as examples of low-molecular weight natural products that modify, extend or enhance microbial metabolism. Mycobacteria are particularly rich in unusual redox cofactors and antioxidants that contribute to redox balance and metabolic plasticity. For instance, mycothiol3,4 or ergothioneine5 protect Mycobacterium tuberculosis from oxidative stress and support detoxification pathways. Coenzyme F420 is involved, e.g., in cell wall biosynthesis6 or defense against nitrosative stress in mycobacteria.7 Moreover, some antimycobacterial drugs like pretomanid8 are administered as prodrugs and will only develop bioactivity after biotransformation by a coenzyme F420-dependent reductase.9 Mycofactocin (MFT) is a putative redox-cofactor whose existence has been postulated on the basis of comparative genomics and bioinformatics.10,11 Its molecular identity and structure, however, have remained elusive to date. The MFT biosynthetic gene cluster is highly conserved and widespread among mycobacteria. The inactivation of the MFT gene locus in the model species Mycolicibacterium smegmatis (synonym: Mycobacterium smegmatis) as well as M. tuberculosis resulted in the inability of the mutants to utilize ethanol as a sole source of carbon and further disturbances of mycobacterial redox homeostasis were revealed.12 Involvement of MFT in methanol metabolism was reported as well.13 These recent results strongly support the hypothesis that MFT is a redox cofactor and might represent a fitness factor of mycobacteria during some stages of infection.
The architecture of the MFT gene cluster (Fig. 1A) suggested that the resulting natural product is a ribosomally synthesized and post-translationally modified peptide (RiPP).14 Several in vitro studies have contributed to a preliminary biosynthetic model of MFT (Fig. 1B): the precursor peptide MftA of M. smegmatis consisting of 31 amino acids is produced by the ribosome and bound by its chaperone MftB. Subsequently, the terminal core peptide consisting of Val and Tyr is oxidatively decarboxylated and cyclized by the radical SAM enzyme MftC.15–17 The resulting cyclic core structure is released by the peptidase MftE18 forming 3-amino-5-[(p-hydroxyphenyl)methyl]-4,4-dimethyl-2-pyrrolidinone (AHDP).19 Just recently, it was shown that MftD, an enzyme homologous to the L-lactate dehydrogenase LldD2
20 catalyzes the oxidative deamination of AHDP to yield pre-mycofactocin (PMFT).21 The same study demonstrated by voltammetry that the α-keto amide moiety of PMFT is redox-active and can be reduced to PMFTH2 (midpoint potential: −255 mV). Efficient reduction was also achieved by the action of carveol dehydrogenase using carveol as an electron donor in vitro.21 Therefore, PMFT likely represents the redox-center of MFT, as riboflavin is the redox-active core of FMN and FAD.
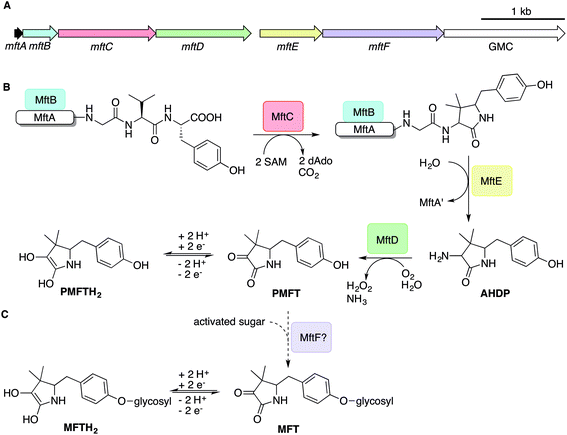 |
| Fig. 1 Biosynthesis of mycofactocin. (A) Schematic representation of the MFT biosynthetic gene cluster of M. smegmatis. Arrows present genes mftA-F. The scale bar indicates 1000 base pairs. (B) Current biosynthesis model of MFT revealed by in vitro studies. The precursor peptide MftA (WP_029104568.1) is bound by its chaperone MftB. The rSAM enzyme MftC catalyzes oxidative decarboxylation and cyclization of the core peptide consisting of a C-terminal Val–Tyr dipeptide. The peptidase MftE releases the cyclized core to form AHDP. MftD performs oxidative deamination of AHDP yielding pre-mycofactocin (PMFT), the presumed redox-active core. (C) The putative glycosyltransferase MftF (WP_011727662.1) was hypothesized to glycosylate premycofactocins. (P)MFT is reduced to (P)MFTH2 (mycofactocinol) by oxidoreductases. dAdo: 5′-deoxyadenosine, GMC: glucose-methanol-choline oxidoreductase, SAM: S-adenosyl methionine. | |
Although these current hypotheses are plausible, all these known metabolic intermediates have only been observed in vitro and could therefore represent artifacts, making the verification of their relevance in vivo urgently desired. Furthermore, additional steps of MFT biosynthesis, the function of the mftF gene as well as the chemical structure of natural MFT awaited experimental clarification. In this study, we confirm the current biosynthetic model of MFT in vivo, detected several novel oligoglycosylated MFT congeners and elucidated their structure. We show that MFTs are decorated with a β-1,4-glucan chain and provide genetic evidence that glycosylation is performed by the glycosyltransferase MftF. Finally, we show dependence of MFT formation on ethanol and corroborate its cofactor function by activity-based metabolic profiling.
Results and discussion
Discovery of mycofactocins by metabolomics
In order to identify potential mycofactocin congeners in mycobacteria, we used the fast-growing and weakly pathogenic species M. smegmatis MC2 155 as a model organism and developed a metabolomics approach combining metabolic induction and labeling to specifically trace MFT congeners. Assuming that MFT production would be stimulated by alcohols, we cultivated bacteria in media containing 10 g L−1 ethanol. Furthermore, we used stable isotope labeling to obtain candidate molecules compatible with the proposed biosynthetic pathway: since the C-terminal core peptide of MftA is composed of Val and Tyr, we reasoned that MFT congeners could be specifically labeled by feeding L-Val-13C5 and L-Tyr-13C9. Intracellular contents were extracted and analyzed by liquid chromatography coupled with high-resolution mass spectrometry (LC-MS). Compounds were detected by in silico grouping of co-eluting isotopic peaks and adducts (feature finding). Afterwards, 13C-labeled compounds were deduced computationally (Data Set 1†). According to the established biosynthetic pathway we expected 13 carbons to remain 13C-labeled after oxidative decarboxylation of the Val–Tyr core peptide. We therefore searched for compounds that displayed an exchange of 13 carbons, resulting in a mass shift of +13.04362 Da (ESI Fig. S1†). This approach revealed a list of only twelve candidate compounds. Strikingly, the exact mass and proposed sum formula of three of these compounds corresponded to known intermediates of MFT, recently described in vitro, namely AHDP, PMFT as well as PMFTH2. In addition to these compounds, several labeled molecules with increasing molecular weight were detected. Some co-eluting candidates with a mass difference of +17.02654 could be explained as NH4+ adducts of each other. The remaining nine candidate compounds (Table 1) were grouped based on their chromatographic retention times, eluting closely to either PMFT or PMFTH2 (approx. 7.2 min and 6.9 min, respectively). Intriguingly, members of the two groups could be arranged in pairs with a mass difference of two hydrogen atoms leading us to assume that each group represented derivatives of either PMFT or PMFTH2. Thus, we termed these two clusters of molecules mycofactocinones (MFT) and mycofactocinols (MFTH2), respectively. Notably, some mycofactocinols eluted as two chromatographically separated isomers. For instance, the dominant PMFTH2 eluted at 6.8 min, while the minor isomer eluted at 6.5 min. These two compounds displayed highly similar MS/MS spectra (ESI Fig. S2†) and most likely represent tautomeric forms. For reasons of simplicity, only the more prevalent isomer was considered during metabolomics studies. We then performed MS/MS networking (Fig. 2), an approach that clusters compounds based on similarity of their MS/MS fragmentation pattern and therefore potentially related chemical scaffolds.22
Table 1 MFT candidate molecules obtained by stable isotope labeling of M. smegmatis with L-Val-13C9 and L-Tyr-13C9a
Name |
Sum formula |
Exact mass (measured) |
RT [min] |
Area (mean) |
Mycofactocinols (MFT-nH2) and mycofactocinones (MFT-n) represent glycosylated forms of PMFTH2 and PMFT, respectively. MMFT-n: methylmycofactocinones, MMFT-nH2: methylmycofactocinols (n: number of saccharide moieties). Area values represent the mean of 4 biological replicates. All labeled compounds are shown in Data Set 1, all MFT congeners revealed in this study are shown in Data Set 2.
|
Aglycons
|
AHDP |
C13H18N2O2 |
234.13683 |
6.53 |
44 862 |
PMFTH2 |
C13H17NO3 |
235.12084 |
6.88 |
49 905 |
PMFT |
C13H15NO3 |
233.10519 |
7.22 |
100 832 |
![[thin space (1/6-em)]](https://www.rsc.org/images/entities/char_2009.gif) |
Mycofactocinols (MFT-nH
2
)
|
MFT-1H2 |
C19H27NO8 |
397.17367 |
6.88 |
53 413 |
![[thin space (1/6-em)]](https://www.rsc.org/images/entities/char_2009.gif) |
Methylmycofactocinols (MMFT-nH
2
)
|
MMFT-2H2 |
C26H39NO13 |
573.24214 |
6.89 |
5722 |
MMFT-7H2 |
C56H89NO38 |
1383.50626 |
6.89 |
340 064 |
MMFT-8H2 |
C62H99NO43 |
1545.55908 |
6.86 |
550 390 |
![[thin space (1/6-em)]](https://www.rsc.org/images/entities/char_2009.gif) |
Methylmycofactocinones (MMFT-n)
|
MMFT-7 |
C56H87NO38 |
1381.49061 |
7.25 |
185 407 |
MMFT-8 |
C62H97NO43 |
1543.54343 |
7.21 |
335 701 |
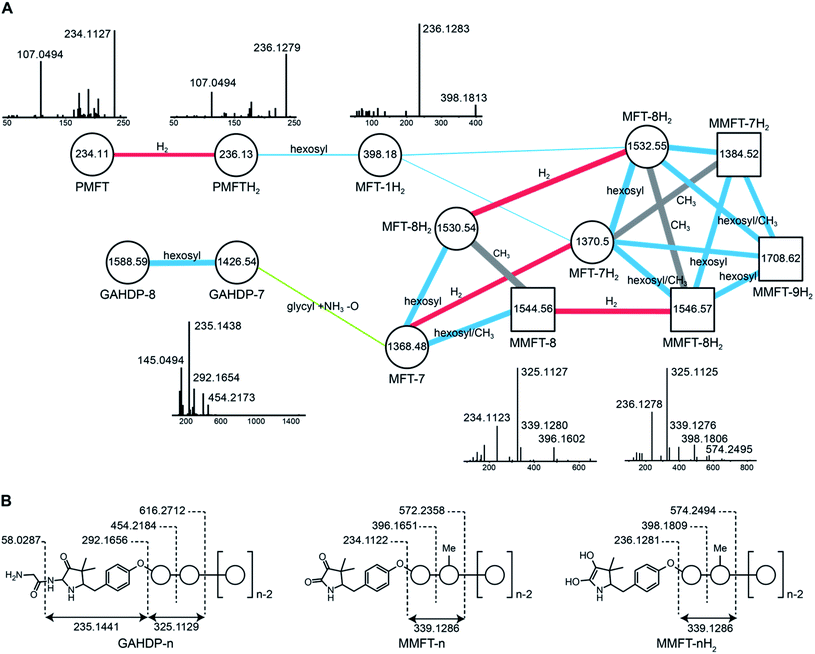 |
| Fig. 2 Discovery and tandem mass spectrometry of MFT congeners. (A) Molecular network of MFT congeners. Nodes (circles) represent chemical compounds. Internal node labels display the precursor mass of compounds (m/z [M + H+]). External node labels show proposed compound annotations. Edges represent relationships in terms of shared MS/MS fragments. Edge labels show proposed modifications based on precursor mass shifts (blue: hexosylation, red: oxidation/reduction, grey: methylation). Line widths of edges mirror cosine distances. Representative MS/MS spectra of corresponding precursor ions are shown above or below nodes. (B) Schematic representation of mass fragmentation patterns of GAHDP-n, MMFT-nH2 and MMFT-n. Numbers indicate mass-to-charge ratios (m/z) of fragments observed. Circles represent hexose moieties. Me: methyl group. | |
Interestingly, candidates retrieved from 13C-labeling experiments clustered with further putative MFT congeners. The mass difference between the first candidate mycofactocinol (MFT-1H2) with an exact mass of 397.17395 Da and PMFTH2 was +162.05303 Da, which corresponded to a hexose sugar.
Furthermore, the MS/MS spectrum of MFT-1H2 (Fig. 2) showed a fragment ion that corresponded to the mass of the putative aglycon (m/z 236.13 [M + H]+), thus supporting the assumption that MFT-1H2 was a glycosylated derivative of PMFTH2. MS/MS networking also revealed a recurrent mass difference of 14.01565 between compounds, indicating that methylation might occur as well. We thus assumed that the MFT candidate molecules could be explained as glycosylated or glycosylated and monomethylated species of PMFT(H2). In analogy to coenzyme F420-n, where n indicates the number of glutamyl residues in the side chain,2 we named the glycosylated molecules MFT-n(H2) with n representing the number of sugar moieties. Monomethylated species were termed methylmycofactocinones (MMFT-n) and methylmycofactocinols (MMFT-nH2), respectively. A targeted search for theoretical mass traces revealed additional members of the MFT-n(H2) and MMFT-n(H2) series (Data Set 2†). As expected, the mycofactocinones exhibited MS/MS fragments with a systematic shift by −2.0016 (e.g., m/z 234.11, 396.16, 572.24) (Fig. 2A) demonstrating that the reduction/oxidation indeed takes place in the PMFT moiety. Oligoglycosylation with up to n = 9 saccharide units was detected, while seven and eight units appeared to be the most dominant forms. We observed both methylated (MMFT) and unmethylated (MFT) sugar chains, with the methylated series being more prominent. Only monomethylated species were found. Mass fragmentation of MMFT-n(H2) species was well in agreement with the assumption that the second sugar was the hotspot for methylation. For instance, MS/MS fragmentation of MMFT-8H2 yielded peaks corresponding to ions of MFT-1H2 (398.18011) and MMFT-2H2 (574.24640) suggesting that the methyl group is present in the second sugar moiety (Fig. 2A).
Structure elucidation of the oligosaccharide moiety
To determine the structure of elongated mycofactocins, we conducted large-scale cultivations. The dominant mycofactocin exhibited the same mass and fragmentation pattern as MMFT-2H2, but eluted at a slightly shifted retention time (Fig. S3†). We therefore named this species MMFT-2bH2. Due to the low yields and co-elution of contaminants, structural analysis by nuclear magnetic resonance (NMR) was not possible at this stage. However, cellulase (β-1,4-glucanase) treatment degraded the sugar chain of mycofactocin species (n > 2), while amylase (α-1,4-glucanase) did not exhibit any effect (Fig. 3A). This finding strongly suggested that the oligosaccharide chain represents a β-1,4-glucan. Intriguingly, isomer MMFT-2bH2, but not MMFT-2H2, accumulated after the enzymatic digest, suggesting that MMFT-2b(H2) represents the product of cellulase digestion of MMFT-7/8(H2) and shares the identical disaccharide anchor. To further elucidate the structure of MMFT-nH2, we analyzed enriched fractions of MMFT-2bH2 and MMFT-nH2 by chemical derivatization and gas chromatography coupled with mass spectrometry (GC-MS). Monosaccharides were released by acid hydrolysis and derivatized by trimethylsilylation (TMS). Comparative analysis of peaks arising from the MMFT-nH2 and MMFT-2bH2 fractions and carbohydrate standards confirmed the presence of D-glucose (Fig. S4†) and revealed that the methylated sugar present in MMFT-n(H2) is 2-O-methyl-D-glucose (Fig. S5†). To confirm the glycosidic linkage positions, the oligosaccharide was permethylated before hydrolysis so that only hydroxyl groups involved in glycosidic bond formation would be free for silylation.23 This experiment (Fig. S6†) lead to the formation of glucose with 2,3,6-O-methyl-1,4-O-TMS modification confirming the 1,4-glycosidic linkage. Additional modification experiments (methanolysis and permethylation) supported the assignments (Fig. S7–S19†). After repeated cultivation we finally obtained MMFT-7/8H2 in sufficient amounts to record 1D and 2D-NMR spectra (ESI results and discussion, Fig. S20–S27, Tables S1 and S2†). The 1H NMR spectrum of MMFT-7/8H2 exhibited a similar five-membered lactam moiety as present in AHDP, but an isolated methine group was shifted to low-field (δH-3 4.28 ppm/δC-3 76.16 ppm) compared to AHDP (δH-3 3.30 ppm/δC-3 61.64 ppm). This indicated the amine group connected to C-3 was replaced by a hydroxyl group. The HMBC correlation between H-1′ to C-11 suggested the sugar chain to be attached to the hydroxyl group of the tyrosine moiety (Fig. 3B). The β-1,4-glycosidic linkage was confirmed by the HMBC correlations of H-1′′ to C-4′ and H-1′′′ to C-4′′ and the configuration of the glucose moiety was assigned as β-form by the large coupling constant of anomeric protons (JH-1′–H-2′ = 8.0 Hz; JH-1′′–H-2′′ = 8.0 Hz; JH-1′′′–H-2′′′ = 8.0 Hz). The position of the methylated glucose was determined by the observation of a methoxy moiety (δH-7′′ 3.64 ppm/δC-7′′ 60.55 ppm) and HMBC correlation of H-7′′ to C-2′′. The planar structure of MMFT-7/8H2 is presented in Fig. 3C.
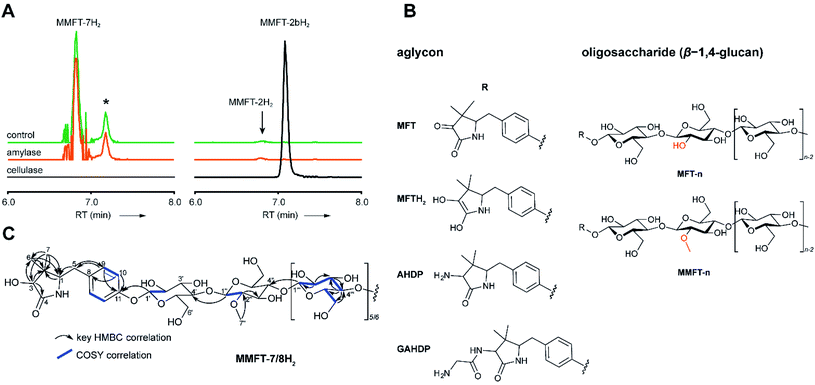 |
| Fig. 3 Structure of mycofactocins. (A) Enzymatic degradation of MMFT-n by cellulase. Extracted ion chromatograms (XIC, [M + H]+) of extract of M. smegmatis corresponding to MMFT-7H2 (m/z 1383.50626, left stack) and MMFT-2H2 or MMFT-2bH2 (m/z 574.24640, right stack) after treatment with cellulase, amylase, or buffer (control) are shown. Asterisk designates a peak corresponding to the M + 2 isotope of MMFT-7. Digestion by cellulase (β-1,4-glucanase) consumes MMFT-nH2 and produces MMFT-2bH2 suggesting that the oligosaccharide consists of β-1,4-linked glucose. (B) Key COSY and HMBC correlations of MMFT-7/8H2. (C) Proposed chemical structures of key mycofactocins and biosynthetic congeners. Mycofactocins are glycosylated by sugar chains consisting of up to nine β-1,4-linked glucose units (n ≤ 9). In methylated mycofactocins (MMFT) the second hexose is methylated (2-O-methyl-D-glucose). The aglycon is PMFT or PMFTH2 in mycofactocinones or mycofactocinols, respectively. The aglycon is AHDP or GAHDP in biosynthetic precursors AHDP-n and GAHDP-n, respectively. | |
In summary, we propose that the oligosaccharide moiety of MFT is a β-1,4-glucane (cellulose). The methylated hexose present in MMFT-n(H2) and MMFT-2b(H2) was shown to be 2-O-methyl-glucose. The fact that MMFT-2 and MMFT-2b (digested MMFT-n) are distinct in retention times points to some degree of structural diversity within MMFTs. Notably, cellulose was shown to be produced by M. tuberculosis as a constituent of biofilms after exposure to reductive stress.24 The production of methylated glucans, like 6-O-methylglucose lipopolysaccharides (MGPL), albeit with α-1,4 linkage, is well described in Mycobacteria, 2-O-methylglucose appears to be less common.25 Glycosylation is a relatively uncommon modification of cofactors. The most important examples are mycothiol4 and bacillithiol.26
Glycine-derived intermediates of MFT biosynthesis
Surprisingly, the MS/MS network (Fig. 2A) revealed two additional compounds (m/z 1426.54 and 1588.59) with an unusual mass shift compared to the MFT-n(H2) candidates. Their mass differences and MS/MS spectra indicated that they represented hepta- and octaglycosylated species sharing a head moiety closely related to PMFT and PMFT(H2). The molecular masses and MS/MS spectra of the compounds could be explained by the assumption that the aglycon corresponded to glycyl-AHDP (GAHDP) and these compounds represented the oligoglycosylated forms GAHDP-7 and GAHDP-8. Since the VY core peptide of MftA is preceded by a glycine residue at its N-terminal side it appeared highly likely that the GAHDP-n species corresponded to premature cleavage products of the MftC-processed precursor peptide. To corroborate this hypothesis, we fed M. smegmatis cultures with a combination of fully 13C-labeled Gly-13C2, Val-13C5, and Tyr-13C9. Indeed, GAHDP-derived molecules underwent a mass shift of +15.05033 Da, indicating the incorporation of Gly-13C2 (+2.00671 Da) in addition to the decarboxylated Val–Tyr moiety (+13.04362 Da) (Fig. S28†). Targeted searches for GAHDP-n as well as AHDP-n (lacking the glycyl residue) and MAHDP-n candidates (AHDP decorated with monomethylated oligosaccharide) revealed three series of oligoglycosylated compounds with similar retention times within each series (Fig. 4, Data Set 2†).
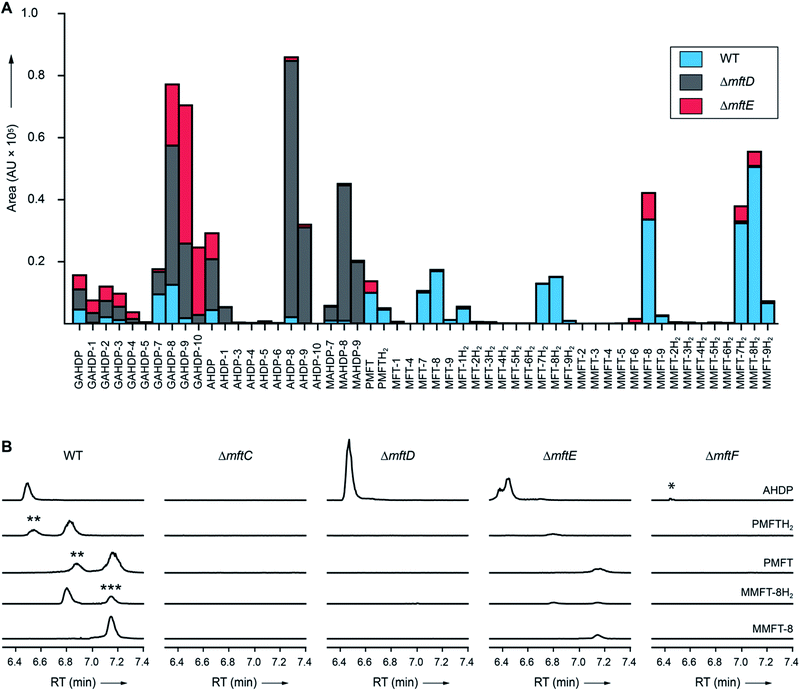 |
| Fig. 4 Metabolic profile of MFT congeners present in M. smegmatis. (A) Distribution of proposed MFT congeners as determined by LC-MS (Data Set 2†). Bars indicate area under the curve of designated species (average of three biological replicates, n = 3). Blue: WT, red: ΔmftE, gray: ΔmftD. The ΔmftE mutant produces significantly reduced amounts of MFT congeners compared to WT, but accumulates incorrectly cleaved products (GAHDP-n series). ΔmftD is unable to produce PMFT(H2) and glycosylated (M)MFT-n(H2), thus accumulating AHDP-n congeners. (B) Extracted ion chromatograms (XIC, [M + H]+) of WT and mutants (ΔmftC, ΔmftD, ΔmftE, ΔmftF) corresponding to AHDP (m/z 235.14411), PMFT (m/z 234.11247), PMFTH2 (m/z 236.12812), MMFT-8 (m/z 1544.55072) and MMFT-8H2 (m/z 1546.56637). **marks minor isomeric forms (Fig. S2†). ***marks a peak corresponding to the M + 2 isotope of MMFT-8. ΔmftC is blocked in biosynthesis of all MFT intermediates, ΔmftF abolishes most of the MFT products, but forms trace amounts of AHDP (*). ΔmftE produces most MFT species in lower amounts, while intermediates like AHDP are increasing. ΔmftD strongly accumulates AHDP, while MFT congeners are abolished. | |
Dissection of MFT biosynthesis
In order to test if all MFT candidate compounds were related to MFT biosynthesis, we investigated mutants (ΔmftC, ΔmftD, ΔmftE, ΔmftF) created previously12 of the MFT biosynthesis pathway for the production of candidate molecules (Fig. 4A, Data Set 2†). Indeed, none of the aglycons, nor any of the glycosylated candidates were detected in the ΔmftC strain (Fig. 4B). This finding, together with the fact that the genetically complemented strain ΔmftC-Comp restored production of MFT congeners (Data Set 2†) represented strong evidence that we indeed identified bona-fide MFT-derivatives.
The ΔmftE mutant was able to produce mycofactocins, albeit in significantly lower amounts, explaining the previously unexpected phenotypic observation that the ΔmftE mutant was able to grow on ethanol, but slower than WT.12 Intriguingly, the pool of GAHDP-n was strongly increased in the ΔmftE strain (Fig. 4A). We thus conclude that MftE can be complemented by an unknown peptidase present in the metabolic background of mycobacteria. Theoretically, an aminopeptidase would be sufficient to degrade the N-terminus of MftA, releasing the AHDP-like core. Peptidases encoded outside the biosynthetic gene cluster have been observed in other RiPP biosyntheses as well.27
However, the removal of the glycine residue might be an apparent bottleneck of the alternative maturation pathway in M. smegmatis. Alternatively, GAHDPs could represent shunt products that cannot be further processed. In full agreement with the in vitro finding that MftD consumes AHDP to form PMFTH2,21 all metabolites downstream of (M)AHDP-n were abrogated in the ΔmftD strain, whereas AHDP-n and GAHDP-n accumulated (Fig. 4). The fact that GAHDP-n increased might suggest that the MftE step is impeded in the absence of MftD as well. Genetic dysregulation or cooperative effects between the two enzymes, like complex formation and substrate channeling, might account for this result.
Glycosylation of MFT is mediated by MftF
It has been speculated that the putative glycosyltransferase MftF catalyzes a final glycosylation of PMFT (Fig. 1C) to yield the mature cofactor.21 Our results at this point showed that multiple glucose residues are indeed attached to the aglycon in vivo. However, glycosylation appeared already at an early stage as mirrored by the presence of the glycosylated (G)AHDP-n series. In order to link oligoglycosylation to a given gene product, we analyzed the ΔmftF mutant for the production of glycosylated MFT congeners. Indeed, all glycosylated MFT congeners were abolished in the ΔmftF metabolome. Unexpectedly, ΔmftF mutants additionally ceased to produce the aglycons PMFT and PMFTH2. MftF did, however, produce trace amounts of AHDP, thus showing that at least residual MftC activity was present in the mutant (Fig. 4B). To exclude polar effects, we complemented ΔmftF by re-introduction of the mftF gene under control of the mftA promotor. The restoration of the full MFT metabolite spectrum (Data Set 2†) excluded polar effects and thus verified that MftF was the glycosyltransferase responsible for oligoglycosylation of MFT congeners. The appearance of glycosylated (G)AHDP species in WT together with the drastic decrease of aglycons in ΔmftF can be interpreted in a scenario where either glycosylation or the MftF protein itself are essential for the MftD step to efficiently take place in vivo. If missing, the biosynthetic machinery may fail to assemble a functional complex or may be unable to recruit the unglycosylated metabolic precursors. The finding that the mftF gene is a conserved constituent of MFT biosynthetic loci among different phyla supports the importance of this modification.11
The deduced MftF protein of M. smegmatis (MSMEG_1426) consists of 470 amino acids (aa) and belongs to the glycosyltransferase 2 family (GT2) according to PFAM (PF00535) and CAZy searches. These enzymes are known for an inverting mechanism of oligoglycoside formation. This is well in agreement with the proposed β-configuration of the MFT oligosaccharide chain. Sequence alignment (Fig. S29A†) showed a high degree of sequence conservation among mycobacterial species and other actinomycetes (e.g., 92% similarity to MftF of M. tuberculosis H37Rv). Prediction of transmembrane domains revealed a single helix spanning residues 324–346 with the N-terminus being located outside of the membrane (Fig. S29B†). The MMFT biosynthetic machinery, however, appears not to be fully encompassed within the MFT cluster since no methyltransferase was found. Future studies are warranted to identify the enzymes involved in MFT oligosaccharide methylation.
Cofactor role of mycofactocin
After discovery of the glycosylated mycofactocins, we examined to which extent their production was actually dependent on the presence of ethanol in culture media. We therefore systematically compared the metabolome of M. smegmatis WT after ethanol treatment with glucose controls (Data Set 3†). The results demonstrated that all MFT congeners or intermediates were strongly upregulated upon cultivation on ethanol (median: 34-fold upregulation) (Fig. 5A). These data perfectly support a recent report that MFT is involved in alcohol metabolism.12
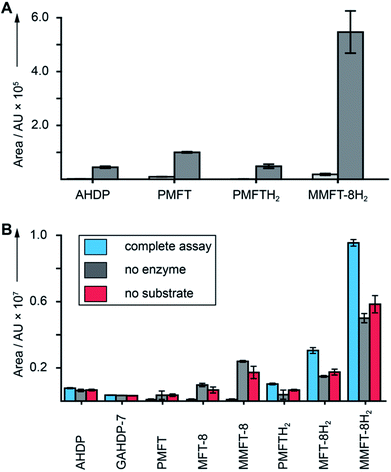 |
| Fig. 5 Cofactor role of mycofactocin. (A) MFT congeners are strongly upregulated (MMFT-8H2: 26-fold) on ethanol-containing media. Area under the curve of MFT species produced by M. smegmatis treated with ethanol (dark gray) versus glucose controls (light gray) are shown (Data Set 3†). (B) Reduction of mycofactocinones to mycofactocinols by treatment of extracts with LimC and carveol. Blue: complete assay with enzyme and L-carveol as substrate. Red: control without substrate, gray: control without enzyme. Bars represent average area under the curve, error bars standard deviation of 3 biological replicates (n = 3) for in both charts. | |
Finally, we sought to confirm that the MFT congeners identified in this study are indeed coenzymes of MFT-dependent enzymes. To assess this question, we turned to activity-based metabolic profiling.28 We incubated the extracted metabolome of M. smegmatis with the recombinant L-carveol dehydrogenase LimC (CAB54559.1) from Rhodococcus erythropolis (Fig. S30†), a nicotinoprotein with a non-exchangable NADH cofactor.29 This enzyme was proposed to require MFT as an external electron acceptor.11 A recent study showed that carveol dehydrogenase from M. smegmatis was able to reduce PMFT to PMFTH2 using carveol and internally bound NADH as an electron donor.21 Likewise, we observed full reduction of all mycofactocinones to mycofactocinols (Fig. 5B, Data Set 4†) by LimC when combined with carveol as a substrate. Controls lacking enzyme or substrate showed weak and no turnover, respectively. The low turnover by LimC alone can be explained by internally bound NADH as reported before.21 Both the aglycon PMFT as well as the oligoglycosylated MFT-n and MMFT-n species were completely turned over, while redox-inactive AHDP congeners remained unaffected. These data further validate the notion that all MFT candidates presented here are mycofactocins with full cofactor function. It remains to be clarified if there is a preference for the glycosylated coenzymes or their aglycons in the bacterial cell.
Conclusion
The redox cofactor mycofactocin has attracted considerable interest since it was postulated by bioinformatics. Despite recent progress made by in vitro studies, evidence for mycofactocin congeners in living microorganisms has been missing so far. Our integrated metabolomics approach combining stable isotope labeling, metabolite induction, MS/MS networking as well as genetic dissection of the biosynthetic pathway turned out to be a powerful approach to identify RiPP congeners in bacteria and could inspire similar projects in the future. Using this technique, we discovered natural MFT and found that it is decorated with oligosaccharides consisting of up to nine β-1,4-linked glucose units. Analyses of ΔmftF mutants and complement strains revealed that MftF is the glycosyltransferase responsible for the oligoglycosylation observed. Mycofactocins can be isolated in oxidized (mycofactocinones) and reduced forms (mycofactocinols) and are co-substrates of enzymatic reduction by carveol dehydrogenase. These data provide strong evidence that mycofactocins are indeed redox cofactors as proposed earlier.11,12,21 We, therefore, conclude that we have finally discovered the family of compounds that was tentatively called “mycofactocin” and thus close an important gap of knowledge in the field. Our results will guide further studies into the occurrence, physiological role, and biochemistry of mycofactocins in microorganisms. Finally, these and other studies will inspire future efforts to exploit mycofactocin, e.g., as a disease marker or as a potential drug target for the treatment mycobacterial infections.
Conflicts of interest
There are no conflicts to declare.
Acknowledgements
We would like to thank the Carl-Zeiss Foundation and the Leibniz Association as well as the European Regional Development Fund for financial support. CB was kindly supported by the CRC ChemBioSys 1127 (Deutsche Forschungsgemeinschaft). We thank Stefan Kaufmann and Gopinath Krishnamoorty for kindly providing M. smegmatis mutants. We also thank Heike Heinecke for NMR measurements.
Notes and references
- J. P. Klinman and F. Bonnot, Chem. Rev., 2014, 114, 4343–4365 CrossRef CAS PubMed.
- C. Greening, F. H. Ahmed, A. E. Mohamed, B. M. Lee, G. Pandey, A. C. Warden, C. Scott, J. G. Oakeshott, M. C. Taylor and C. J. Jackson, Microbiol. Mol. Biol. Rev., 2016, 80, 451–493 CrossRef CAS PubMed.
- N. A. Buchmeier, G. L. Newton, T. Koledin and R. C. Fahey, Mol. Microbiol., 2003, 47, 1723–1732 CrossRef CAS PubMed.
- G. L. Newton, N. Buchmeier and R. C. Fahey, Microbiol. Mol. Biol. Rev., 2008, 72, 471–494 CrossRef CAS PubMed.
- V. Saini, B. M. Cumming, L. Guidry, D. A. Lamprecht, J. H. Adamson, V. P. Reddy, K. C. Chinta, J. H. Mazorodze, J. N. Glasgow, M. Richard-Greenblatt, A. Gomez-Velasco, H. Bach, Y. Av-Gay, H. Eoh, K. Rhee and A. J. C. Steyn, Cell Rep., 2016, 14, 572–585 CrossRef CAS PubMed.
- E. Purwantini and B. Mukhopadhyay, PLoS One, 2013, 8, e81985 CrossRef PubMed.
- E. Purwantini and B. Mukhopadhyay, Proc. Natl. Acad. Sci. U. S. A., 2009, 106, 6333–6338 CrossRef CAS PubMed.
- C. K. Stover, P. Warrener, D. R. VanDevanter, D. R. Sherman, T. M. Arain, M. H. Langhorne, S. W. Anderson, J. A. Towell, Y. Yuan, D. N. McMurray, B. N. Kreiswirth, C. E. Barry and W. R. Baker, Nature, 2000, 405, 962–966 CrossRef CAS PubMed.
- R. Singh, U. Manjunatha, H. I. Boshoff, Y. H. Ha, P. Niyomrattanakit, R. Ledwidge, C. S. Dowd, I. Y. Lee, P. Kim, L. Zhang, S. Kang, T. H. Keller, J. Jiricek and C. E. Barry III, Science, 2008, 322, 1392–1395 CrossRef CAS PubMed.
- R. Ayikpoe, V. Govindarajan and J. A. Latham, Appl. Microbiol. Biotechnol., 2019, 103, 2903–2912 CrossRef CAS PubMed.
- D. H. Haft, BMC Genomics, 2011, 12, 21 CrossRef CAS PubMed.
- G. Krishnamoorthy, P. Kaiser, L. Lozza, K. Hahnke, H. J. Mollenkopf and S. H. E. Kaufmann, mBio, 2019, 10, e00190-19 CrossRef PubMed.
- A. A. Dubey and V. Jain, Biochem. Biophys. Res. Commun., 2019, 516, 1073–1077 CrossRef CAS PubMed.
- P. G. Arnison, M. J. Bibb, G. Bierbaum, A. A. Bowers, T. S. Bugni, G. Bulaj, J. A. Camarero, D. J. Campopiano, G. L. Challis, J. Clardy, P. D. Cotter, D. J. Craik, M. Dawson, E. Dittmann, S. Donadio, P. C. Dorrestein, K. D. Entian, M. A. Fischbach, J. S. Garavelli, U. Goransson, C. W. Gruber, D. H. Haft, T. K. Hemscheidt, C. Hertweck, C. Hill, A. R. Horswill, M. Jaspars, W. L. Kelly, J. P. Klinman, O. P. Kuipers, A. J. Link, W. Liu, M. A. Marahiel, D. A. Mitchell, G. N. Moll, B. S. Moore, R. Muller, S. K. Nair, I. F. Nes, G. E. Norris, B. M. Olivera, H. Onaka, M. L. Patchett, J. Piel, M. J. Reaney, S. Rebuffat, R. P. Ross, H. G. Sahl, E. W. Schmidt, M. E. Selsted, K. Severinov, B. Shen, K. Sivonen, L. Smith, T. Stein, R. D. Sussmuth, J. R. Tagg, G. L. Tang, A. W. Truman, J. C. Vederas, C. T. Walsh, J. D. Walton, S. C. Wenzel, J. M. Willey and W. A. van der Donk, Nat. Prod. Rep., 2013, 30, 108–160 RSC.
- N. A. Bruender and V. Bandarian, Biochemistry, 2016, 55, 2813–2816 CrossRef CAS PubMed.
- B. Khaliullin, P. Aggarwal, M. Bubas, G. R. Eaton, S. S. Eaton and J. A. Latham, FEBS Lett., 2016, 590, 2538–2548 CrossRef CAS PubMed.
- B. Khaliullin, R. Ayikpoe, M. Tuttle and J. A. Latham, J. Biol. Chem., 2017, 292, 13022–13033 CrossRef CAS PubMed.
- N. A. Bruender and V. Bandarian, J. Biol. Chem., 2017, 292, 4371–4381 CrossRef CAS PubMed.
- R. Ayikpoe, J. Salazar, B. Majestic and J. A. Latham, Biochemistry, 2018, 57, 5379–5383 CrossRef CAS PubMed.
- S. Billig, M. Schneefeld, C. Huber, G. A. Grassl, W. Eisenreich and F. C. Bange, Sci. Rep., 2017, 7, 6484 CrossRef PubMed.
- R. S. Ayikpoe and J. A. Latham, J. Am. Chem. Soc., 2019, 141, 13582–13591 CrossRef CAS PubMed.
- M. Wang, J. J. Carver, V. V. Phelan, L. M. Sanchez, N. Garg, Y. Peng, D. D. Nguyen, J. Watrous, C. A. Kapono, T. Luzzatto-Knaan, C. Porto, A. Bouslimani, A. V. Melnik, M. J. Meehan, W. T. Liu, M. Crusemann, P. D. Boudreau, E. Esquenazi, M. Sandoval-Calderon, R. D. Kersten, L. A. Pace, R. A. Quinn, K. R. Duncan, C. C. Hsu, D. J. Floros, R. G. Gavilan, K. Kleigrewe, T. Northen, R. J. Dutton, D. Parrot, E. E. Carlson, B. Aigle, C. F. Michelsen, L. Jelsbak, C. Sohlenkamp, P. Pevzner, A. Edlund, J. McLean, J. Piel, B. T. Murphy, L. Gerwick, C. C. Liaw, Y. L. Yang, H. U. Humpf, M. Maansson, R. A. Keyzers, A. C. Sims, A. R. Johnson, A. M. Sidebottom, B. E. Sedio, A. Klitgaard, C. B. Larson, C. A. Boya P., D. Torres-Mendoza, D. J. Gonzalez, D. B. Silva, L. M. Marques, D. P. Demarque, E. Pociute, E. C. O'Neill, E. Briand, E. J. N. Helfrich, E. A. Granatosky, E. Glukhov, F. Ryffel, H. Houson, H. Mohimani, J. J. Kharbush, Y. Zeng, J. A. Vorholt, K. L. Kurita, P. Charusanti, K. L. McPhail, K. F. Nielsen, L. Vuong, M. Elfeki, M. F. Traxler, N. Engene, N. Koyama, O. B. Vining, R. Baric, R. R. Silva, S. J. Mascuch, S. Tomasi, S. Jenkins, V. Macherla, T. Hoffman, V. Agarwal, P. G. Williams, J. Dai, R. Neupane, J. Gurr, A. M. C. Rodriguez, A. Lamsa, C. Zhang, K. Dorrestein, B. M. Duggan, J. Almaliti, P. M. Allard, P. Phapale, L. F. Nothias, T. Alexandrov, M. Litaudon, J. L. Wolfender, J. E. Kyle, T. O. Metz, T. Peryea, D. T. Nguyen, D. VanLeer, P. Shinn, A. Jadhav, R. Muller, K. M. Waters, W. Shi, X. Liu, L. Zhang, R. Knight, P. R. Jensen, B. O. Palsson, K. Pogliano, R. G. Linington, M. Gutierrez, N. P. Lopes, W. H. Gerwick, B. S. Moore, P. C. Dorrestein and N. Bandeira, Nat. Biotechnol., 2016, 34, 828–837 CrossRef CAS PubMed.
- A. Debettigniesdutz, G. Reznicek, B. Kopp and J. Jurenitsch, J. Chromatogr., 1991, 547, 299–306 CrossRef CAS.
- A. Trivedi, P. S. Mavi, D. Bhatt and A. Kumar, Nat. Commun., 2016, 7, 11392 CrossRef CAS PubMed.
- G. Stadthagen, T. Sambou, M. Guerin, N. Barilone, F. Boudou, J. Kordulakova, P. Charles, P. M. Alzari, A. Lemassu, M. Daffe, G. Puzo, B. Gicquel, M. Riviere and M. Jackson, J. Biol. Chem., 2007, 282, 27270–27276 CrossRef CAS PubMed.
- G. L. Newton, M. Rawat, J. J. La Clair, V. K. Jothivasan, T. Budiarto, C. J. Hamilton, A. Claiborne, J. D. Helmann and R. C. Fahey, Nat. Chem. Biol., 2009, 5, 625–627 CrossRef CAS PubMed.
- S. Chen, B. Xu, E. Chen, J. Wang, J. Lu, S. Donadio, H. Ge and H. Wang, Proc. Natl. Acad. Sci. U. S. A., 2019, 116, 2533–2538 CrossRef CAS PubMed.
- L. P. de Carvalho, H. Zhao, C. E. Dickinson, N. M. Arango, C. D. Lima, S. M. Fischer, O. Ouerfelli, C. Nathan and K. Y. Rhee, Chem. Biol., 2010, 17, 323–332 CrossRef CAS PubMed.
- M. J. van der Werf, C. van der Ven, F. Barbirato, M. H. Eppink, J. A. de Bont and W. J. van Berkel, J. Biol. Chem., 1999, 274, 26296–26304 CrossRef CAS PubMed.
Footnote |
† Electronic supplementary information (ESI) available: ESI Appendix: materials and methods, supplementary figures, supplementary results and discussion (.PDF). Data Set 1: 13C-labeled compounds (.xlsx). Data Set 2: comparative metabolomics comparing M. smegmatis WT, mutant and complement strains (.xlsx). Data Set 3: comparative metabolomics of M. smegmatis WT treated with glucose or ethanol (.xlsx). Data Set 4: activity-based metabolic profiling (.xlsx). See DOI: 10.1039/d0sc01172j |
|
This journal is © The Royal Society of Chemistry 2020 |
Click here to see how this site uses Cookies. View our privacy policy here.