DOI:
10.1039/D0SC02559C
(Edge Article)
Chem. Sci., 2020,
11, 7921-7926
Reductive radical-initiated 1,2-C migration assisted by an azidyl group†
Received
6th May 2020
, Accepted 6th July 2020
First published on 7th July 2020
Abstract
We report here a novel reductive radical-polar crossover reaction that is a reductive radical-initiated 1,2-C migration of 2-azido allyl alcohols enabled by an azidyl group. The reaction tolerates diverse migrating groups, such as alkyl, alkenyl, and aryl groups, allowing access to n+1 ring expansion of small to large rings. The possibility of directly using propargyl alcohols in one-pot is also described. Mechanistic studies indicated that an azidyl group is a good leaving group and provides a driving force for the 1,2-C migration.
Since the groups of Ryu and Sonoda described the reductive radical-polar crossover (RRPCO) concept in the 1990s,1 it has attracted considerable attention in modern organic synthesis.2 By using this concept, a variety of complex molecules could be assembled in a fast step-economic fashion which is not possible using either radical or polar chemistry alone. However, only two RRPCO reaction modes are known to date: nucleophilic addition and nucleophilic substitution (Fig. 1A). The first RRPCO reaction is the nucleophilic addition of organometallic species, which is generated in situ from the reduction of a strong reducing metal with a carbon-centered radical intermediate and cations (E+ = H+, I+, Br+, path 1).3 However, the necessity for a large amount of harmful and strong reducing metals has greatly limited the scope and functional group tolerance of the reaction. Recently, photoredox catalysis has not only successfully overcome the shortcomings of using toxic strong reducing metals in the RRPCO reaction,4 but also enabled the development of several new RRPCO reaction types, including the nucleophilic addition with carbonyl compounds or carbon dioxide (path 2),5 the cyclization of alkyl halides/tosylates (path 3),6 and β-fluorine elimination (path 4).7 Although the RRPCO reaction has been greatly advanced by photoredox catalysis, it is still in its infancy, and the development of a novel RRPCO reaction is of great importance.
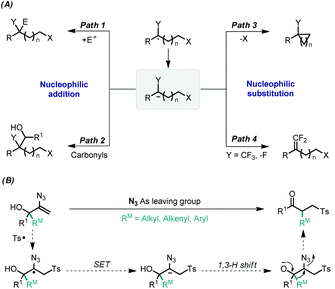 |
| Fig. 1 (A) Reductive radical-polar crossover reactions; (B) this work: reductive radical-initiated 1,2-C migration assisted by an azidyl group. | |
Herein, we wish to report a new type of reductive radical-polar crossover cascade reaction that is the reductive radical-initiated 1,2-C migration under metal-free conditions (Fig. 1B). The development of this approach is not only to further expand the application of the RRPCO reaction, but also to solve the problems associated with the oxidative radical-initiated 1,2-C migration, such as the necessity for an oxidant and/or transition metal for the oxidative termination of the radicals, and also required sufficient ring strain to avoid the generation of epoxy byproducts.8 To realize this reaction, a driving force is needed to drive the 1,2-C migration after reductive termination, to avoid the otherwise inevitable protonation of the generated anion.9 Inspired by the leaving group-induced semipinacol rearrangement,10 we envisaged that 2-azidoallyl alcohols11 might be the ideal substrates for the reductive radical-initiated 1,2-C migration because these compounds contain both an allylic alcohol motif, which is vital for the radical-initiated 1,2-C migration, and an azidyl group, a good leaving group,12 which may facilitate the 1,2-C migration after the reductive termination of the radicals.
With the optimal conditions established (ESI, Table S1†), we then explored the scope of this radical-initiated 1,2-migration. As shown in Table 1, a series of naphthenic allylic alcohols could undergo n+1 ring expansion with minimal impact on the product yield (Table 1, 3aa–aq). Notably, only the alkyl groups were migrated when using benzonaphthenic allylic alcohols in the reaction. These results might be attributed to the aryl group possessing greater steric resistance. The structure of 3an was further verified by single-crystal diffraction. Interestingly, the vinyl azide derived from a pharmaceutical ethisterone was also a viable substrate, affording the migration product 3aq in 57% yield, which highlighted the applicability of this strategy in the late-stage modification of pharmaceuticals. Moreover, the acyclic allylic alcohol with an alkyl chain also successfully delivered the migration product 3ar in 64% yield.
Table 1 Substrate scope of 2-azidoallyl alcoholsab
Standard reaction conditions: 1 (0.5 mmol), TMSN3 (2.0 mmol), 2a (3.0 mmol) in H2O (0.7 mL) and DMSO (1.4 mL) at 50 °C in air for 48 h.
Isolated yields.
|
|
Next, we extend the reaction scope to a range of aryl allylic alcohols. In comparison with alkyl allylic alcohols, aryl allylic alcohols gave the migration products in higher yields. The structure of 3ba was unambiguously confirmed by X-ray single crystal diffraction (CCDC 1897779).† As demonstrated by the arene scope (Table 1, 3ba–bl), a variety of aryl allylic alcohols, including electron-withdrawing phenyl, electron-donating phenyl, polysubstituted phenyl, and fused rings, afforded the corresponding products in moderate to high yields (67–89%). Unsurprisingly, the substrates containing electron-donating groups afforded higher yields than those containing electron-withdrawing groups.
Phenols and their derivatives are important structural constituents of numerous pharmaceuticals, agrochemicals, polymers, and natural products.13 The most common method for synthesising phenols is the hydroxylation of aryl halides.14 However, the method usually requires transition metals and harsh reaction conditions. Interestingly, by using the current strategy, inexpensive and abundant cyclopentadiene moieties can also be easily converted into phenols (Table 1, 3ca–cc) in moderate to good yield. Thus, this strategy provides metal-free and mild conditions for accessing phenols.
Next, we investigated the migration capabilities of different groups (Table 2). When using a substrate that contains two different alkyl groups (1da), the product with the less sterically hindered alkyl group is obtained in a higher migration ratio. A comparison of aryl groups and alkyl groups in the same allylic alcohols showed that the migration of aryl groups was more facile, and the migration ratio ranged from 1
:
4 to 1
:
1.3 (3db–dd). The results of the migration ratio of different aryl groups (3de–dh) revealed that aryl moieties with electron-donating groups possessed higher migration ratios than aryl moieties with electron-withdrawing groups.
Table 2 Investigation of the migration efficiency

|
Entry |
1
|
R1 |
R2 |
Yielda (%) |
3d
|
3d′
|
Isolated yields.
|
1 |
1da
|
Me |
t-Bu |
15 |
42 |
2 |
1db
|
Me |
C6H5 |
53 |
26 |
3 |
1dc
|
Me |
4-MeOC6H5 |
56 |
14 |
4 |
1dd
|
Me |
4-CF3C6H5 |
42 |
32 |
5 |
1de
|
C6H5 |
4-MeC6H5 |
42 |
40 |
6 |
1df
|
C6H5 |
4-MeOC6H5 |
46 |
39 |
7 |
1dg
|
C6H5 |
4-ClC6H5 |
41 |
44 |
8 |
1dh
|
C6H5 |
4-CF3C6H5 |
36 |
48 |
After the evaluation of the scope of our allylic alcohols, we turned our attention to sulfonyl radical precursors (Table 3). We carried out the reaction of various sodium sulfinates with allylic alcohol 1ba under standard conditions. Pleasingly, the sodium sulfinates with straight chain alkyl (3ea), cyclic alkyl (3eb), and aryl (3ec–ef) groups were all suitable for this radical-initiated 1,2-carbon migration, and afforded corresponding products in 71–91% yield.
Table 3 Substrate scope of sodium sulfinatesa
Isolated yields.
|
|
In this work, the 2-azidoallyl alcohols substrates were derived from propargylic alcohols through a silver-catalyzed hydroazidation of alkynes.15 Consequently, we hypothesized that the radical-initiated 1,2-carbon migration could be directly achieved from propargylic alcohols in a one pot process. With a slight modification of the reaction conditions, we realized the one-pot preparation of the desired products from propargylic alcohols (Table 4). Propargylic alcohols containing cyclic alkyl (3ag and 3ah), heterocyclic alkyl (3ak and 3al), acyclic alkyl (3ar), and aryl (3ba) groups all gave the desired migration products, although the yields were slightly lower than those from the reactions of the 2-azidoallyl alcohols. It should be noted that the ring expansion products could be directly generated from a bioactive compound, ethisterone (3aq). Performing such a reaction in a single step could greatly reduce the cost of pharmaceutical modification. The fused phenol (3cd) could also be obtained in moderate yield via the one-step reaction. In addition, the migration order of the different substituted groups (3db) was nearly identical to that observed in vinyl azide-based protocol. Furthermore, alkyl sodium sulfinates (3ea) were also well tolerated.
Table 4 Substrate scope of propargyl alcoholsa,b
Standard reaction conditions: 4 (0.5 mmol), TMSN3 (2.0 mmol), 2 (3.0 mmol), Ag2CO3 (0.05 mmol) in H2O (0.7 mL) and DMSO (1.4 mL) at 50 °C in air for 48 h.
Isolated yields.
|
|
To gain more insight into the mechanism of radical-initiated 1,2-carbon migration, we conducted various experiments to confirm the presence or absence of radical and carbanion intermediates (Scheme 1). When the reaction of 1ba was performed in the presence of TEMPO (6.0 equiv.), the reaction was suppressed under the standard conditions (Scheme 1, eqn (1)), supporting the involvement of a radical intermediate. To prove the formation of a carbanion intermediate, we carried out two deuterium labeling experiments (Scheme 1, eqn (2) and (3)). The resulting products [D]-3ba and MA-1 contain the deuterium atom α in the carbonyl group, confirming the formation of a carbanion intermediate. To identify the key intermediate of the 1,2-migration, we prepared a potential intermediate M1 and subjected it to the standard conditions (Scheme 1, eqn (4)). But, the product 3ba was not observed and almost all of the M1 was recovered, which indicates that M1 is not a key intermediate. However, the product 3ba was obtained in a yield of 41% while M2 was subjected to the standard conditions (eqn (5)). If the hydroxyl group in the 2-azidoallyl alcohols was protected (M3), the reaction would not give the corresponding migration product (3ga), but generate product 5 with a yield of 51% (eqn (6)).11c These results proved that the reaction involved a 1,3-H migration process thereby enabling an oxygen anion intermediate IV (other mechanistic studies are discussed in ESI Fig. S1†).
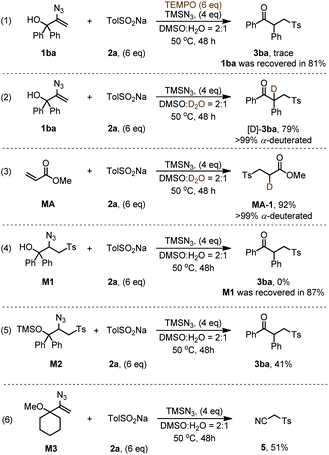 |
| Scheme 1 Mechanistic investigations. | |
Based on the above experimental results and relevant literature, a possible reaction pathway was proposed as shown in Fig. 2. First, TolSO2TMS (I) is generated by the anion exchange of TolSO2Na with TMSN3. Such intermediates are known to be somewhat unstable,16 as similar to the analogous compounds, such as TolSO2I,17 and TMSTePh18 and thus undergo homolysis. Therefore, we anticipated that TolSO2TMS (I) should also yield sulfonyl and trimethylsilyl radicals.19 Then the 2-azidoallyl alcohol 1ba is readily attacked by the sulfonyl radical, leading to carbon-centered radical II. Subsequently, the carbon-centered radical II undergoes single electron transfer by the oxidation of sulfinate to the sulfonyl radical yielding the carbanion III.20 A 1,3-H shift of carbanion III affords the intermediate IV21 which rapidly undergoes 1,2-migration with the assistance of the azidyl leaving group, generating the desired product. It is worth noting that the present work is a novel radical reaction mode for vinyl azides compared to the existing reports that involve N–N bond breaking in the presence of radicals. Moreover, the development of this strategy is of great significance for the application of vinyl azides in the reconstruction of C–C bonds.
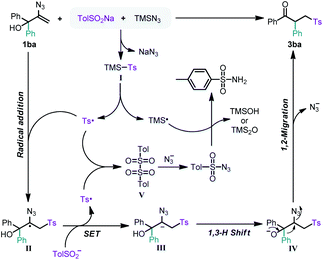 |
| Fig. 2 Proposed mechanism. | |
On the other hand, the coupling of sulfonyl radicals produces intermediate V.22 The azidyl anion that is generated in the reaction is more prone to attack intermediate V to afford tosyl azide.23 Subsequently, tosyl azide is reduced to p-toluenesulfonamide by the trimethylsilyl radical.24 The sideproducts tosyl azide and p-toluenesulfonamide were isolated by column chromatography, and the associated TMSOH and TMS2O have been detected by GC-MS.25
Conclusions
In conclusion, we report a novel RRPCO reaction: reductive radical-initiated 1,2-C migration under transition-metal free conditions. The key driving force for this procedure was the presence of an azidyl group as a good leaving group. This reaction features broad substrate scope, good functional group tolerance, and the facile generation of diverse ketones and phenols. Moreover, the direct use of propargyl alcohols in a one-pot process was also established, providing high step economy. Mechanistic studies reveal that the combination of sodium sulfinates and TMSN3 plays a vital role in the generation of sulfonyl radicals and the reduction of carbon radicals. Further efforts to develop an asymmetrical version of this novel reductive radical-initiated 1,2-C migration are underway in our laboratory.
Conflicts of interest
There are no conflicts to declare.
Acknowledgements
The authors thanks the National Natural Science Foundation of China (Grant #. 21604082), the Department of Science and Technology of Jilin Province (Grant #. 20190201076JC and 20190103128JH), and the Fundamental Research Funds for the Central Universities (Grant #. 2412019FZ006).
Notes and references
- S. Tsunoi, I. Ryu, S. Yamasaki, M. Tanaka, N. Sonoda and M. Komatsu, Chem. Commun., 1997, 1889–1890 RSC.
-
(a)
Radicals in organic synthesis, ed. P. Renaud and M. P. Sibi, Wiley-VCH Verlag GmbH, Weinheim, 2001 Search PubMed;
(b) L. Pitzer, J. L. Schwarz and F. Glorius, Chem. Sci., 2019, 10, 8285–8291 RSC.
-
(a) F. Denes, F. Chemla and J. F. Normant, Angew. Chem., Int. Ed., 2003, 42, 4043–4046 CrossRef CAS PubMed;
(b) F. Denes, S. Cutri, A. Perez-Luna and F. Chemla, Chem.–Eur. J., 2006, 12, 6506–6513 CrossRef CAS PubMed;
(c) A. Perez-Luna, C. Botuha, F. Ferreira and F. Chemla, Chem.–Eur. J., 2008, 14, 8784–8788 CrossRef CAS PubMed.
-
(a) Y. Miyake, K. Nakajima and Y. Nishibayashi, J. Am. Chem. Soc., 2012, 134, 3338–3341 CrossRef CAS PubMed;
(b) P. Kohls, D. Jadhav, G. Pandey and O. Reiser, Org. Lett., 2012, 14, 672–675 CrossRef CAS PubMed;
(c) Y. Yasu, T. Koike and M. Akita, Adv. Synth. Catal., 2012, 354, 3414–3420 CrossRef CAS;
(d) L. Chu, C. Ohta, Z. Zuo and D. W. C. MacMillan, J. Am. Chem. Soc., 2014, 136, 10886–10889 CrossRef CAS PubMed;
(e) A. El Marrouni, C. B. Ritts and J. Balsells, Chem. Sci., 2018, 9, 6639–6646 RSC.
-
(a) V. R. Yatham, Y. Shen and R. Martin, Angew. Chem., Int. Ed., 2017, 56, 10915–10919 CrossRef CAS PubMed;
(b) J. L. Schwarz, F. Schäfers, A. Tlahuext-Aca, L. Lückemeier and F. Glorius, J. Am. Chem. Soc., 2018, 140, 12705–12709 CrossRef CAS PubMed;
(c) A. Hu, Y. Chen, J. Guo, N. Yu, Q. An and Z. Zuo, J. Am. Chem. Soc., 2018, 140, 13580–13585 CrossRef CAS PubMed;
(d) L. Liao, G. Cao, J. Ye, G. Sun, W. Zhou, Y. Gui, S. Yan, G. Shen and D. Yu, J. Am. Chem. Soc., 2018, 140, 17338–17342 CrossRef CAS PubMed;
(e) T. Ju, Q. Fu, J. Ye, Z. Zhang, L. Liao, S. Yan, X. Tian, S. Luo, J. Li and D. Yu, Angew. Chem., Int. Ed., 2018, 57, 13897–13901 CrossRef CAS PubMed;
(f) Q. Fu, Z. Bo, J. Ye, T. Ju, H. Huang, L. Liao and D. Yu, Nat. Commun., 2019, 10, 3592–3600 CrossRef PubMed;
(g) H. Mitsunuma, S. Tanabe, H. Fuse, K. Ohkubo and M. Kanai, Chem. Sci., 2019, 10, 3459–3465 RSC;
(h) K. Donabauer, M. Maity, A. L. Berger, G. S. Huff, S. Crespi and B. König, Chem. Sci., 2019, 10, 5162–5166 RSC;
(i) Q. Meng, T. E. Schirmer, A. L. Berger, K. Donabauer and B. König, J. Am. Chem. Soc., 2019, 141, 11393–11397 CrossRef CAS PubMed;
(j) S. Ni, N. M. Padial, C. Kingston, J. C. Vantourout, D. C. Schmitt, J. T. Edwards, M. M. Kruszyk, R. R. Merchant, P. K. Mykhailiuk, B. B. Sanchez, S. Yang, M. A. Perry, G. M. Gallego, J. J. Mousseau, M. R. Collins, R. J. Cherney, P. S. Lebed, J. S. Chen, T. Qin and P. S. Baran, J. Am. Chem. Soc., 2019, 141, 6726–6739 CrossRef CAS PubMed.
-
(a) Y. Zhang, R. Qian, X. Zheng, Y. Zeng, J. Sun, Y. Chen, A. Ding and H. Guo, Chem. Commun., 2015, 51, 54–57 RSC;
(b) T. Guo, L. Zhang, X. Liu, Y. Fang, X. Jin, Y. Yang, Y. Li, B. Chen and M. Ouyang, Adv. Synth. Catal., 2018, 360, 4459–4463 CrossRef CAS;
(c) C. Shu, R. S. Mega, B. J. Andreassen, A. Noble and V. K. Aggarwal, Angew. Chem., Int. Ed., 2018, 57, 15430–15434 CrossRef CAS PubMed;
(d) C. Shu, R. A. Noble and V. K. Aggarwal, Angew. Chem., Int. Ed., 2019, 58, 3870–3874 CrossRef CAS PubMed;
(e) J. P. Phelan, S. B. Lang, J. S. Compton, C. B. Kelly, R. Dykstra, O. Gutierrez and G. A. Molander, J. Am. Chem. Soc., 2018, 140, 8037–8047 CrossRef CAS PubMed;
(f) J. A. Milligan, J. P. Phelan, V. C. Polites, C. B. Kelly and G. A. Molander, Org. Lett., 2018, 20, 6840–6844 CrossRef CAS PubMed;
(g) L. R. E. Pantaine, J. A. Milligan, J. K. Matsui, C. B. Kelly and G. A. Molander, Org. Lett., 2019, 21, 2317–2321 CrossRef CAS PubMed;
(h) W. Luo, Y. Yang, Y. Fang, X. Zhang, X. Jin, G. Zhao, L. Zhang, Y. Li, W. Zhou, T. Xia and B. Chen, Adv. Synth. Catal., 2019, 361, 4215–4221 CrossRef CAS.
-
(a) S. B. Lang, R. J. Wiles, C. B. Kelly and G. A. Molander, Angew. Chem., Int. Ed., 2017, 56, 15073–15077 CrossRef CAS PubMed;
(b) J. P. Phelan, S. B. Lang, J. Sim, S. Berritt, A. J. Peat, K. Billings, L. Fan and G. A. Molander, J. Am. Chem. Soc., 2019, 141, 3723–3732 CrossRef CAS PubMed.
-
(a) Z. M. Chen, W. Bai, S. H. Wang, B. M. Yang, Y. Q. Tu and F. M. Zhang, Angew. Chem., Int. Ed., 2013, 52, 9781–9785 CrossRef CAS PubMed;
(b) Z. M. Chen, Z. Zhang, Y. Q. Tu, M. H. Xu, F. M. Zhang, C. C. Li and S. H. Wang, Chem. Commun., 2014, 50, 10805–10808 RSC;
(c) A. Bunescu, Q. Wang and J. Zhu, Angew. Chem., Int. Ed., 2015, 54, 3132–3135 CrossRef CAS PubMed;
(d) R. Honeker, R. A. Garza-Sanchez, M. N. Hopkinson and F. Glorius, Chem.–Eur. J., 2016, 22, 4395–4399 CrossRef CAS PubMed;
(e) W. Z. Weng, J. G. Sun, P. Li and B. Zhang, Chem.–Eur. J., 2017, 23, 9752–9755 CrossRef CAS PubMed;
(f) E. E. Touney, N. J. Foy and S. V. Pronin, J. Am. Chem. Soc., 2018, 140, 16982–16987 CrossRef CAS PubMed.
-
(a) K. Iwasaki, K. K. Wan, A. Oppedisano, S. W. M. Crossley and R. Shenvi, J. Am. Chem. Soc., 2014, 136, 1300–1303 CrossRef CAS PubMed;
(b) C. Obradors, R. M. Martinez and R. A. Shenvi, J. Am. Chem. Soc., 2016, 138, 4962–4971 CrossRef CAS PubMed.
-
(a) Z. L. Song, C. A. Fan and Y. Q. Tu, Chem. Rev., 2011, 111, 7523–7556 CrossRef CAS PubMed;
(b) B. Wang and Y. Q. Tu, Acc. Chem. Res., 2011, 44, 1207–1222 CrossRef CAS PubMed.
-
(a) J. Fu, G. Zanoni, E. A. Anderson and X. Bi, Chem. Soc. Rev., 2017, 46, 7208–7228 RSC;
(b) H. Hayashi, A. Kaga and S. Chiba, J. Org. Chem., 2017, 82, 11981–11989 CrossRef CAS PubMed;
(c) J. R. Donald and S. L. Berrell, Chem. Sci., 2019, 10, 5832–5836 RSC;
(d) Y. Gai, Y. Ning, P. Sivaguru, X. Li, Y. Zhao, J. Wu and X. Bi, Sci. China: Chem., 2020, 63, 460–466 CrossRef CAS.
-
(a) J. P. Richard, T. L. Amyes, Y. Lee and V. Jagannadham, J. Am. Chem. Soc., 1994, 116, 10833–10834 CrossRef CAS;
(b) X. Zhu, Y. Wang, F. Zhang and S. Chiba, Chem.–Asian J., 2014, 9, 2458–2462 CrossRef CAS PubMed;
(c) B. Hu and S. G. DiMagno, Org. Biomol. Chem., 2015, 13, 3844–3855 RSC;
(d) L. Zhang, G. Sun and X. Bi, Chem.–Asian J., 2016, 11, 3018–3021 CrossRef CAS PubMed;
(e) N. Thirupathi, C. Tung and Z. Xu, Adv. Synth. Catal., 2018, 360, 3585–3589 CrossRef CAS;
(f) M. Lorenzin, A. Guerriero and F. Pietra, J. Org. Chem., 1980, 45, 1704–1705 CrossRef CAS.
-
Z. Rappport, The Chemistry of Phenols, Wiley-VCH Verlag GmbH & Co. KGaA, Weinheim, 2003 Search PubMed.
-
(a) M. C. Willis, Angew. Chem., Int. Ed., 2007, 46, 3402–3404 CrossRef CAS PubMed;
(b) S. Enthaler and A. Company, Chem. Soc. Rev., 2011, 40, 4912–4924 RSC;
(c)
A. Tlili and M. Taillefer, in Copper-mediated cross-coupling reactions, ed. E. Evano and N. Blanchard, John Wiley & Sons Inc., Hoboken, NJ, USA, 2013, pp. 41–91 Search PubMed.
-
(a) Z. Liu, J. Liu, L. Zhang, P. Liao, J. Song and X. Bi, Angew. Chem., Int. Ed., 2014, 53, 5305–5309 CrossRef CAS PubMed;
(b) Z. Liu, P. Liao and X. Bi, Org. Lett., 2014, 16, 3668–3671 CrossRef CAS PubMed.
-
(a) D. C. Lenstra, V. Vedovato, E. F. Flegeau, J. Maydom and M. C. Willis, Org. Lett., 2016, 18, 2086–2089 CrossRef CAS PubMed;
(b) X. Huang, C. Craita, L. Awad and P. Vogel, Chem. Commun., 2005, 1297–1299 RSC;
(c) B. K. Zambroń, S. R. Dubbaka, D. Marković, E. Moreno-Clavijo and P. Vogel, Org. Lett., 2013, 15, 2550–2553 CrossRef PubMed.
-
(a) Y. Fang, Z. Luo and X. Xu, RSC Adv., 2016, 6, 59661–59676 RSC;
(b) Y. Guo, S. Lu, L. Tian, E. Huang, X. Hao, X. Zhu, T. Shao and M. Song, J. Org. Chem., 2018, 83, 338–349 CrossRef CAS PubMed.
-
(a) H. Miyazoe, S. Yamago and J. Yoshida, Angew. Chem., Int. Ed., 2000, 39, 3669–3671 CrossRef CAS;
(b) S. Yamago, H. Miyazoe, K. Iida and J. Yoshida, Org. Lett., 2000, 2, 3671–3673 CrossRef CAS PubMed;
(c) S. Yamago, M. Miyoshi, H. Miyazoe and J. Yoshida, Angew. Chem., Int. Ed., 2002, 41, 1407–1409 CrossRef CAS.
-
(a) Y. Ning, Q. Ji, P. Liao, E. A. Anderson and X. Bi, Angew. Chem., Int. Ed., 2017, 56, 13805–13808 CrossRef CAS PubMed;
(b) Y. Ning, X. Zhao, Y. Wu and X. Bi, Org. Lett., 2017, 19, 6240–6243 CrossRef CAS PubMed;
(c) B. Liu, Y. Ning, M. Virelli, G. Zanoni, E. A. Anderson and X. Bi, J. Am. Chem. Soc., 2019, 141, 1593–1598 CrossRef CAS PubMed.
- D. Marković, A. Varela-Álvarez, J. A. Sordo and P. Vogel, J. Am. Chem. Soc., 2006, 128, 7782–7795 CrossRef PubMed.
-
(a) I. F. Galván, M. A. Aguilar and M. F. Ruiz-López, J. Phys. Chem. B, 2005, 109, 23024–23030 CrossRef PubMed;
(b) A. Vázquez-Romero, A. B. Gómz and B. Martín-Matute, ACS Catal., 2015, 5, 708–714 CrossRef.
- L. Cao, S. Luo, K. Jiang, Z. Hao, B. Wang, C. Pang and Z. Wang, Org. Lett., 2018, 20, 4754–4758 CrossRef CAS PubMed.
- J. L. Kice, G. J. Kasperek and D. Patterson, J. Am. Chem. Soc., 1969, 91, 5516–5522 CrossRef CAS.
- L. Benati, G. Bencivenni, R. Leardini, M. Minozzi, D. Nanni, R. Scialpi, P. Spagnolo and G. Zanardi, J. Org. Chem., 2006, 71, 5822–5825 CrossRef CAS PubMed.
-
(a) T. Wang and N. Jiao, J. Am. Chem. Soc., 2013, 135, 11692–11695 CrossRef CAS PubMed;
(b) X. Sun, X. Li, S. Song, Y. Zhu, Y. Liang and N. Jiao, J. Am. Chem. Soc., 2015, 137, 6059–6066 CrossRef CAS PubMed.
Footnotes |
† Electronic supplementary information (ESI) available. CCDC 1897875 and 1897779. For ESI and crystallographic data in CIF or other electronic format see DOI: 10.1039/d0sc02559c |
‡ These authors contributed equally to this work. |
|
This journal is © The Royal Society of Chemistry 2020 |
Click here to see how this site uses Cookies. View our privacy policy here.