Efficient cosensitization of new organic dyes containing bipyridine anchors with porphyrins for dye-sensitized solar cells†
Received
16th September 2019
, Accepted 22nd October 2019
First published on 22nd October 2019
Abstract
One of the core problems facing DSSCs is that they only partially absorb the solar spectrum, and a feasible solution is to co-sensitize two or more dyes with different absorption spectra to achieve panchromatic absorption. Herein, two organic dyes S3 and S4 containing bipyridine anchoring groups were prepared and used as co-sensitizers to assemble solar cells with porphyrin dye JA3. In the structural design of S3 and S4, we use diphenylthiazide and carbazole with a long carbon chain as electron donors and dipyridine with better planarity as an electron acceptor, and this is conducive to increasing the intramolecular charge transfer performance of dyes, inhibiting dye aggregation and increasing the amount of dye loading. Optical performance analysis shows that the light-harvesting ability of the device has been significantly improved after the co-sensitization of the two organic molecules with porphyrin dye JA3. At the same time, the charge recombination of porphyrin dyes has been significantly reduced because the appropriate molecular volume structures of S3 and S4 can fill the adsorption gap between porphyrin dyes. The DSSC based on JA3 shows a PCE of 6.23%, with a Voc of 801 mV, a Jsc of 12.23 mA cm−2, and a FF of 63.55%. After co-sensitization, the overall performance of the device has been significantly improved; both short-circuit current and open-circuit voltage have been greatly enhanced. The DSSC based on JA3 + S3 shows a high PCE of 8.20%, with a Voc of 821 mV, a Jsc of 15.46 mA cm−2, and a FF of 64.55%. The co-sensitizer not only successfully compensates for the absorption defects of porphyrin dyes in the range of 500–600 nm and improves the light response current, but also fills the adsorption gap between the main dyes, and effectively suppresses the charge recombination behavior, thus improving the open-circuit voltage of the device.
Introduction
Although the development of the times and the progress of science and technology have brought convenience to people's life, they have also caused serious environmental pollution and energy crisis. At present, how to effectively develop and utilize new energy sources to replace traditional fossil fuels is one of the important problems faced by many countries. Dye-sensitized solar cells (DSSCs) are considered to be one of the most promising alternatives to fossil energy, and they have many advantages, such as a simple fabrication process, low price, wide material sources, multi-color transparency, good flexibility and so on.1–3 Sensitizers play an important role in the performance of DSSCs, and developing efficient sensitizers has always been one of the key research directions. In the past 30 years, researchers have developed a series of highly effective dyes; generally speaking, they mainly include ruthenium complex dyes, metalloporphyrin dyes and metal-free organic dyes.4–8 At present, important progress has been made in the research of these dyes, and some highly effective dyes have been reported one after another. The energy conversion efficiency (PCE) of many highly efficient sensitizers has been stabilized at about 13%.9–12 It is believed that with the deepening of the research, new breakthroughs will be made in DSSCs.
Imitating the principle of photosynthesis in plants is an important feature of DSSCs, and dyes play an important role in broadening the spectral response of devices; excited dye molecules inject electrons into the conduction bands of titanium dioxide semiconductors to achieve photogenerated charge separation and transfer.13–16 Developing efficient dyes is the most direct and effective way to improve the energy conversion efficiency of DSSCs; the zinc porphyrin dye SM315 prepared by the Grätzel team in 2014 has a PCE of 13.0%,17 and in 2015, Kakiage and co-workers synthesized carbazole dye ADEKA-1 and the conversion efficiency of devices was up to 14.3% with cobalt-based redox electrolytes.18 Although great progress has been made in the design of dyes, many dyes still have the problem of insufficient spectral responses. A poor spectral response directly limits the light-harvesting performance of devices, which is very disadvantageous to short-circuit current. A large number of literature studies show that one of the core problems DSSCs still face is that they only partially absorb the solar spectrum, and when the solar spectrum cannot be completely covered by the optical absorption of a single dye, a feasible solution is to co-sensitize two or more dyes with different absorption spectra to achieve panchromatic absorption.19–23 The co-sensitization strategy has become an effective method to improve the spectral response, and the working electrodes prepared by this method contain two or more different dyes with complementary optical absorption properties.24,25 However, how to choose a suitable co-sensitizer is an important issue. A co-sensitizer should have not only a complementary effect on optical absorption, but also a very suitable size. Otherwise, serious desorption of the main dye will occur during co-sensitization, which will reduce the energy conversion efficiency of the device.
We found that although porphyrin dyes have good photoelectric properties and wide absorption in the 400–450 and 600–650 nm regions, they also have some unavoidable disadvantages. The planarity of porphyrin rings leads to dye aggregation and π–π interaction, and there is no absorption in the range of 500–600 nm.26–29 In this paper, we designed organic co-sensitizers S3 and S4 containing bipyridine anchoring groups; we first sensitize the main dye JA3 on the surface of the photoanode, and then sensitize it with the co-sensitizer to make up for the absorption defect of the main dye and fill the gap between the main dyes (Fig. 1).30 This strategy effectively enhances and broadens the spectral response of dyes, makes up for the deficiency of porphyrin dye absorption, and reduces the charge recombination behavior by molecular filling. As expected, the spectral response of the device after co-sensitization has been significantly improved. The PCE of the device based on JA3 is 6.23%, and after co-sensitization, both short circuit current and open circuit voltage have been greatly improved. In particular for the device based on JA3 + S3, the PCE reached 8.20%, with a Voc of 821 mV, a Jsc of 15.46 mA cm−2, and a FF of 64.55%. We also studied the optical properties, electrochemical properties, and photovoltaic performance to analyze the differences between these devices.
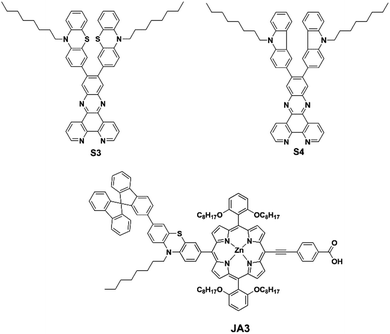 |
| Fig. 1 Structures of S3, S4 and JA3. | |
Experimental
General information
All solvents were treated by standard methods before use and all chemicals were purchased from commercial suppliers and used without further purification unless indicated otherwise. N,N-Dimethylformamide (DMF) and tetrahydrofuran (THF) were dried and distilled from CaH2. The 1H NMR spectra were recorded on a Bruker DRX NMR spectrometer with tetramethylsilane (TMS) as the internal standard.
Synthesis of dyes
The synthetic routes of S3 and S4 are depicted in Scheme S1.† The synthesis details and characterization data are shown in the ESI.†
Fabrication of DSSCs
For the preparation of a DSSC, FTO glass plates were cleaned in a detergent solution using an ultrasonic bath for 30 min two times and then rinsed with water and ethanol. Then, the plates were immersed in 40 mM TiCl4 (aqueous) at 70 °C for 30 min and washed with water and ethanol. The TiO2 paste consisted of a 12 μm thick film (particle size, 20 nm; pore size, 32 nm). The TiO2 films were treated with a programmed procedure: (1) 80 °C for 15 min; (2) 135 °C for 10 min; (3) 325 °C for 30 min; (4) 375 °C for 5 min; (5) 450 °C for 15 min; and (6) 500 °C for 15 min. Then the films were treated again with TiCl4 at 70 °C for 30 min and sintered at 500 °C for 30 min. Then the electrode was immersed in 0.2 mM JA3 (TFH/EtOH = 1/4) for 2 h; the porphyrin-sensitized films were washed with ethanol and dried, and then immersed in 0.3 mM co-sensitizer (S3 and S4) solution in a mixture of THF and ethanol (THF/ethanol = 1/1) for 1 h at room temperature. The working electrode and the Pt counter electrode were then sealed with a Surlyn film (25 μm) by heating the sandwich-type cell at 110 °C. The electrolyte was introduced through pre-drilled holes in the counter electrode and was driven into the cell via vacuum backfilling, and the holes were sealed with a Surlyn film and a thin glass plate (0.1 mm thickness) cover by heating. The electrolyte was composed of 0.6 M 1-butyl-3-methylimidazolium iodide (BMII), 50 mM I2, 50 mM LiI, 0.5 M tert-butylpyridine and 0.1 M guanidinium thiocyanate (GuNCS) in acetonitrile.
Characterization of DSSCs
The photocurrent–voltage (I–V) curves of the DSSCs were measured on a Keithley 2400 source meter under standard global AM 1.5G solar irradiation supplied by a xenon light source (Oriel). The incident photo-to-electron conversion efficiency (IPCE) spectra of the DSSCs were measured by a DC method. The light source was a 300 W xenon lamp (Oriel 6258) coupled with a flux controller to improve the stability of the irradiance. The single wavelength was selected by using a monochromator (Cornerstone 260 Oriel 74125). Light intensity was measured by using a NREL traceable Si detector (Oriel 71030NS) and the short circuit currents of the DSSCs were measured by using an optical power meter (Oriel 70310).
UV-vis spectroscopy, photoluminescence, electrochemical properties and measurement amounts of dye loading
The UV-vis absorption spectra were recorded on a Shimadzu UV-3600 spectrometer. Fluorescence spectra were recorded on a PerkinElmer LS55 spectrophotometer. Cyclic voltammetry (CV), differential pulse voltammetry (DPV), and Electrochemical Impedance Spectroscopy (EIS) were performed using a Chenhua CHI760E model Electrochemical Workstation (Shanghai). The amounts of dye loading on the TiO2 films were measured by using a Shimadzu UV-3600 spectrometer. The sensitized electrodes were immersed in a 0.1 M NaOH solution in a mixed solvent (H2O/THF = 1/4), which resulted in desorption of each dye. The amounts of dye loading can be estimated according to the following formula: C = AV/εS0, where C stands for the amount of dye loading, A is the optical absorbance of the dye, V is the volume of desorption solution, ε is the molar extinction coefficients, and S0 is the effective area of TiO2 films.
Results and discussion
Optical properties
As shown in Fig. 2, the UV-vis absorption spectra of S3 and S4 were measured in DCM, and the relevant parameters are collected in Table S1.† Obviously, the two dyes have obvious absorption in the visible region, and there are major absorption bands in the range of 400–600 nm, and this can be attributed to intramolecular charge transfer (ICT) properties. For S3, it shows a strong absorption peak at 456 nm, with a high molar absorption coefficient (ε) of 1.49 × 104 M−1 cm−1. In addition, the spectral response of S3 reached 600 nm, and this means that S3 has a good light-harvesting ability and is very suitable as a co-sensitizer. The absorption spectrum of S4 was blue-shifted by 16 nm compared to that of S3, and the highest ε of S4 was 1.46 × 104 M−1 cm−1 at 440 nm. In addition, the spectra response of S1 reached about 650 nm, while the spectral response of S2 reached about 600 nm. By comparison, S3 has better spectral response performance, and this should be attributed to the better electron-donor properties and more conjugated structures of phenothiazine.
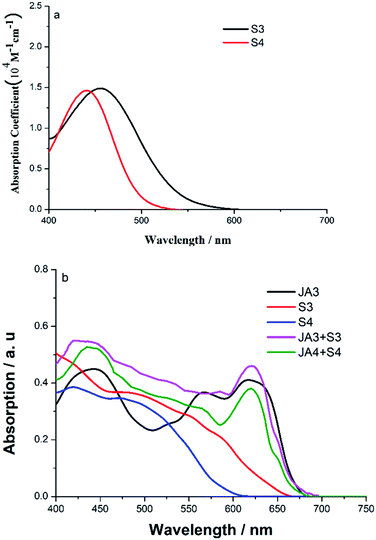 |
| Fig. 2 (a) UV-vis absorption spectra of S3 and S4 in DCM, and (b) UV-vis absorption spectra of JA3, S3, S4, JA3 + S3 and JA3 + S4 anchored on TiO2 surfaces. | |
In order to observe the spectral response after co-sensitization, the absorption spectra of the dyes anchored on TiO2 films were further measured. As shown in Fig. 2b, compared with the absorption spectra of S3 and S4 in DCM, their absorption curves widen markedly. The spectral response of S3 reached about 680 nm, and the spectral response of S4 reached about 610 nm. Moreover, their absorption peaks were redshifted compared with those in the solution state; S3 showed a strong absorption peak at 473 nm and S3 showed a strong absorption peak at 470 nm, and this should be attributed to the J-aggregation effect. Although we introduced double long carbon chains into the donor part to inhibit aggregation, the smaller molecular volume of the co-sensitizer and the better planarity of the acceptor make it inevitable that the aggregation effect will occur. However, the broadening of the spectral response range is beneficial to enhance the photon capture ability of devices. After co-sensitization, we found that the absorption spectra of JA3 changed significantly. We can clearly see that the response ability of JA3 in the visible region is enhanced, especially in the 450–600 nm region, and the spectral response of JA3 + S3 has been greatly improved. Although the absorption response of JA3 + S4 in the long wavelength region is slightly reduced, which may be due to a certain degree of desorption of JA3, its absorption in the 400–550 nm region increased significantly. This indicates that the light-harvesting ability of the device will be greatly improved after co-sensitization, and the short circuit current will also be improved.
Electrochemical studies
In the design of the dye molecular structure, level matching is a very important prerequisite. Better regeneration efficiency and high electron injection efficiency of dyes are prerequisites for high open-circuit voltage and short-circuit current of devices. In order to observe the ability of electron injection and dye regeneration, we can use cyclic voltammetry (CV) and differential pulse voltammetry (DPV) to study the corresponding ground state oxidation potential (EOX) of S3 and S4, so as to evaluate the regeneration ability and the ability of excited state electron injection of these two dyes.31–33 As shown in Fig. S1–S3,† we tested the CV and DPV properties of these two dyes. In order to facilitate a clearer analysis of dye regeneration ability and excitation state charge injection ability, we show the levels in Fig. 3. It is clear that the ground state oxidation potentials (EOX) of S3 and S4 are 0.94 V and 0.96 V (versus NHE) from DPV curves, respectively. Compared with the redox potential of the I−/I3− couple (0.4 V), they are much more positive than 0.4 V, which means that the oxidized dyes can be effectively recycled. In addition, the zero–zero excitation energy (E0–0) can be estimated by the intersection of fluorescence and absorption spectra (Fig. S4†), and the values of S3 and S4 are 2.37 eV and 2.40 eV, respectively. Thus, the LUMO of S3 and S4 is −1.43 V and −1.44 V, respectively. They are all more negative than the conduction band of TiO2 (−0.5 V versus NHE), and the result indicates that the electrons from the two excited dyes can efficiently inject into the conduction band of TiO2.
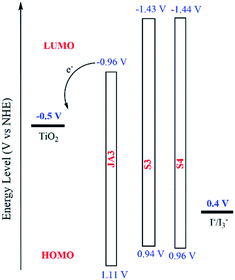 |
| Fig. 3 Schematic energy diagram of S3 and S4. | |
Photovoltaic performance of DSSCs
In order to study the photovoltaic properties of these dyes, DSSCs were prepared under the same conditions. The corresponding photovoltaic parameters are listed in Table S2,† and the J–V curves of DSSCs are given in Fig. 4. We can see that the PCE of the DSSC based on S3 is 4.06%, with a Voc is 723 mV, Jsc is 8.25 mA cm−2, and FF is 68.00%, while the PCE of the DSSC based on S4 is 3.23%, with a Voc is 686 mV, Jsc is 6.46 mA cm−2, and FF is 73.02%. Obviously, the high open-circuit voltage and short-circuit current of the DSSC based on S3 are the main reasons for its high conversion efficiency. Compared with the carbazole electron donor, the phenothiazine donor exhibits better electron donation and conjugation properties, and previous absorption spectroscopic studies also showed that S3 has a better light-harvesting ability, and this is conducive to increasing the Jsc of the DSSCs. In addition, we also found that the devices based on these two dyes have higher open-circuit voltage, especially the Voc of S3 reaches 723 mV. The compactness of dye molecule arrangement is conducive to inhibiting the penetration of the oxidizing electrolyte into the photoanode, which helps to reduce the charge recombination behavior. Through previous work, we know that JA3 shows a high energy conversion efficiency of 6.23%, with a Voc of 801 mV, a Jsc of 12.23 mA cm−2, and a FF of 63.55%. Although JA3 has a high open-circuit voltage, its spectral response is not ideal, especially in the 500–600 nm region; therefore, we use a sequential co-sensitization strategy to improve its spectral response ability. As expected, the overall performance of the devices after co-sensitization has been greatly improved. In particular for the DSSC based on JA3 + S3, the Jsc was enhanced from 12.23 mA cm−2 to 15.46 mA cm−2, which is consistent with the improvement of their spectral responsiveness. Besides, we also found that the Voc of the DSSCs was improved significantly after co-sensitization, increasing by nearly 20 mV.
 |
| Fig. 4 (a) The J–V curves of DSSCs based on JA3, S3, S4, JA3 + S3 and JA3 + S4 and (b) the IPCE curves of DSSCs based on JA3, S3, S4, JA3 + S3 and JA3 + S4. | |
In addition, as shown in Table S2,† we extract the shunt and series resistances of each device from I–V curves. Generally speaking, the series resistance will affect the filling factor and short-circuit current of the device, and the shunt resistance reflects the leakage level of the device, thus affecting the filling factor of the device. The Rs of the DSSCs decreased in the following order: S3 (98 Ω cm−2) > JA3 + S3 (77 Ω cm−2) > S4 (74 Ω cm−2) > JA3 + S4 (64 Ω cm−2) > JA3 (59 Ω cm−2), and the Rsh decreased in the following order: S4 (38
989 Ω cm−2) > JA3 (20
577 Ω cm−2) > S3 (20
444 Ω cm−2) > JA3 + S4 (17
925 Ω cm−2) > JA3 + S3 (12
242 Ω cm−2). We can see that S4 has smaller Rs and largest Rsh, which corresponds to its maximum FF (73.02%). Although the Rs of S3 is slightly higher, its Rsh is larger, so its filling factor reaches 68.00%. After cosensitization, the Rs of the device does not change much, and the Rsh decreases to a certain extent, which may be the main reason for the slight decrease of the filling factor.
We further measured the incident photon-to-current conversion efficiency (IPCE) spectra of the DSSCs to study the differences between Jsc. As shown in Fig. 4b, we can see from the curve of IPCE that S3 has a photocurrent response range of nearly 660 nm, which is wider than that of S4, and has a relatively high response value. The IPCE value of S3 reached 62.7% at 464 nm, and the IPCE value of S4 was 59.9% at 458 nm; the photocurrent response range of S4 was about 600 nm, and we can see that the response range of photocurrent is consistent with their absorption spectrum. In order to improve the PCE of the DSSCs, S3 and S4 were used as the co-sensitizers. Obviously, the Jsc (15.46 mA cm−2) of JA3 + S3 increased 3.23 mA cm−2 compared with the Jsc (12.23 mA cm−2) of JA3, whereas the Jsc (13.08 mA cm−2) of JA3 + S4 increased 0.85 mA cm−2 compared with the Jsc (12.23 mA cm−2) of JA3. The main reason for the increase of short-circuit current density is that the spectral response performance of devices has been significantly enhanced after co-sensitization, which can be seen from the analysis of their optical properties. In addition, we can also clearly see that their IPCE curves have changed significantly. For JA3, the IPCE values reached 58.4%, 38.7%, and 49.7% at 440 nm, 570 nm, and 630 nm, respectively. Compared with JA3, the photocurrent response of JA3 + S3 and JA3 + S4 increases obviously. For JA3 + S3, the IPCE value increased to 64.2% at 440 nm, and the overall response of the IPCE curve has been significantly improved. Especially in the range of 450–600 nm, the increase of the photocurrent response amplitude of JA3 + S3 is more obvious, which corresponds to its highest current. Like the spectral response, although the IPCE of JA3 + S4 in the long wavelength region is slightly reduced, the photocurrent response in the 400–550 nm region increased significantly. In addition, we integrated the IPCE curves. The Jsc for the DSSCs under solar illumination was predicted by convolution of the IPCE spectra with the photo-flux density distribution for 1 sun as the following equation:34
where
q is the electron charge and
F(
λ) is the incident photon flux density (AM 1.5, ASTM G173) at wavelength
λ. The
Jsc values from the IPCE curves of JA3, S3, S4, JA3 + S3 and JA3 + S4 are 8.21, 5.45, 4.44, 10.32, and 8.81 mA cm
2, respectively, and this trend is consistent with
I–
V test results. The differences from the experimental results are probably due to the slight spectral mismatch, and the response of IPCE was different from that of the simulated xenon lamp. In the process of co-sensitization, a small amount of desorption may occur in the main dye, which is also the main reason for the slight decrease of photoresponse current at 650 nm, so the time of co-sensitization cannot be too long. We further measured the amounts of dye loading, and the values of JA3, S3, and S4 are 1.25 × 10
−7, 3.23 × 10
−7, and 2.17 × 10
−7 mol cm
−2, respectively. After co-sensitization, the amount of dye loading has changed. For JA3 + S3, the value of JA3 is about 1.12 × 10
−7 mol cm
−2, and the value of S3 is about 0.68 × 10
−7 mol cm
−2. For JA3 + S4, the value of JA3 is about 0.94 × 10
−7 mol cm
−2, and the value of S4 is about 0.44 × 10
−7 mol cm
−2, and the dye JA3 undergoes a slight desorption. Moreover, we found that the
Voc values of the cosensitized DSSCs were both larger than those of the corresponding individual DSSCs. It is mainly because the co-sensitizers can occupy the voids between the main dye molecules, which can avoid the main dye aggregation and prevent the I
3− of the electrolyte from penetrating into the TiO
2 surface, so as to reduce the charge recombination and improve the
Voc of the devices.
23,35
Electrochemical impedance spectroscopy measurements
Under the same assembly conditions, including the same photoanode, electrolyte and counter electrode, the fluctuation of charge density (charge recombination rate) in the conduction band of the photoanode has a great influence on the open-circuit voltage of the device.36,37 Considering that charge recombination is an important factor limiting Voc, EIS was performed to study the electron recombination dynamics under dark conditions, and the applied voltage was −0.7 V and scanned from 105 to 1 Hz.
We can see two obvious semicircles in the Nyquist plots of Fig. 5. The small semicircle in the high frequency region represents the electron transfer resistance at the Pt/electrolyte interface, while the large semicircle in the middle frequency region represents the charge transfer resistance at the TiO2/dye/electrolyte interface. As can be seen from Table S3,† the radius of the semicircle decreases in the intermediate frequency range in the following order: JA3 + S3 (596 Ω cm−2) ≈ JA3 + S4 (568 Ω cm−2) > JA3 (117 Ω cm−2). The larger Rct at the TiO2/dye/electrolyte interface also indicates that the charge recombination is less, under similar operating conditions, and it also shows obvious advantages over some reported values, which is also consistent with its high open-circuit voltage.38,39 This indicates that the charge recombination is obviously inhibited after co-sensitization, and the larger charge recombination impedance is conducive to suppressing the recombination reaction between the electrons in the conduction band of TiO2 and the I3− in the electrolyte. This is also consistent with their high Voc, so appropriate sensitizers can effectively improve the Voc of the devices. In addition, we can also evaluate the dark current by measuring the charge lifetime of the battery devices, which will also have a certain impact on the open circuit voltage of the devices. Generally speaking, the longer the charge lifetime, the lower the dark current, which is conducive to improving its open-circuit voltage.40,41 By measuring the Bode phase of devices, we analyze the differences between the charge lifetimes of various devices, as shown in Fig. S5.† The peak frequency (f) in the lower frequency region from the Bode phase plots and the electron lifetime (τ) can be calculated by using τ = 1/(2πf). The f of JA3, JA3 + S3, and JA3 + S4 is 7.0, 3.8 and 3.5 Hz, respectively. Thus, the corresponding electron lifetime values are 22.7, 41.9 and 45.5 ms, respectively, and the trend is also consistent with the Voc of DSSCs.
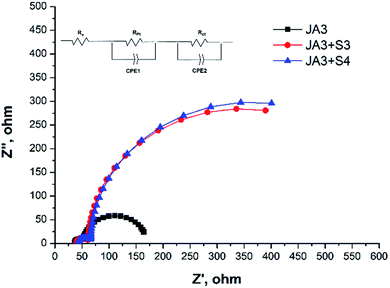 |
| Fig. 5 Nyquist plots of the DSSCs based on JA3, JA3 + S3, and JA3 + S4. The interpolated graph is the equivalent circuit (Rs, RPt, and Rct are the series resistance, charge transfer resistance at the Pt/electrolyte, and at the TiO2/dye/electrolyte interface, respectively; CPE1 and CPE2 are the constant phase element for the Pt/electrolyte interface and TiO2/dye/electrolyte, respectively). | |
Conclusions
The co-sensitization strategy has become an effective method to improve the performance of DSSCs, and the combination of dyes can greatly broaden the spectral response range of dyes, so it can improve the light-harvesting ability of devices. In this work, two organic dyes S3 and S4 containing bipyridine anchoring groups were prepared and used as co-sensitizers to assemble solar cells with porphyrin dye JA3. The results indicate that the light-harvesting ability of the devices has been significantly improved after the co-sensitization of the two organic molecules with porphyrin dye JA3. In addition, the charge recombination of porphyrin dyes has been significantly reduced because the appropriate molecular volume structures of S3 and S4 can fill the adsorption gap between porphyrin dyes. After co-sensitization, the DSSC based on JA3 + S3 shows a high PCE of 8.20%, with a Voc of 821 mV, a Jsc of 15.46 mA cm−2, and a FF of 64.55%. The co-sensitizers used in this paper not only successfully compensate for the absorption defect of porphyrin dyes in the range of 500–600 nm and improve the photoresponse current, but also fill the adsorption gap between the main dyes through reasonable structure design and effectively suppress the charge recombination rate, thus improving the open-circuit voltage of the devices. The strategy has a good scientific guiding significance for improving the overall performance of DSSCs.
Conflicts of interest
There are no conflicts to declare.
Acknowledgements
This work was supported by grants from the National Natural Science Foundation of China (21701060), Changzhou Sci Tech Program (CJ20190079), China and Natural Science Foundation of the Higher Education Institutions of Jiangsu Province (17KJB150015, 18KJA150003), China.
Notes and references
- S. Ardo and G. J. Meyer, Chem. Soc. Rev., 2009, 38, 115 RSC.
- M.-E. Ragoussi and T. Torres, Chem. Commun., 2015, 51, 3957 RSC.
- F. Bella, C. Gerbaldi, C. Barolo and M. Grätzel, Chem. Soc. Rev., 2015, 44, 3431 RSC.
- K. L. V. Joseph, N. T. M. Rosana, R. Easwaramoorthi, J. J. Vijaya, S. Karthikeyan and J. K. Kim, New J. Chem., 2019, 43, 10834 RSC.
- H. Chen, G. Lyu, Y. Yue, T. Wang, D.-P. Li, H. Shi, J. Xing, J. Shao, R. Zhang and J. Liu, J. Mater. Chem. C, 2019, 7, 7249 RSC.
- A. F. Buene, N. Boholm, A. Hagfeldt and B. H. Hoff, New J. Chem., 2019, 43, 9403 RSC.
- R. Kesavan, I. M. Abdellah, S. P. Singh, A. El-Shafei and A. V. Adhikari, Phys. Chem. Chem. Phys., 2019, 21, 10603 RSC.
- A. Slodek, D. Zych, S. Golba, S. Zimosz, P. Gnida and E. Schab-Balcerzak, J. Mater. Chem. C, 2019, 7, 5830 RSC.
- Z. Yao, H. Wu, Y. Li, J. Wang, J. Zhang, M. Zhang, Y. Guo and P. Wang, Energy Environ. Sci., 2015, 8, 3192 RSC.
- Z. Yao, M. Zhang, H. Wu, L. Yang, R. Li and P. Wang, J. Am. Chem. Soc., 2015, 137, 3799 CrossRef CAS PubMed.
- J. Luo, M. Xu, R. Li, K.-W. Huang, C. Jiang, Q. Qi, W. Zeng, J. Zhang, C. Chi, P. Wang and J. Wu, J. Am. Chem. Soc., 2014, 136, 265 CrossRef CAS PubMed.
- Y. Xie, Y. Tang, W. Wu, Y. Wang, J. Liu, X. Li, H. Tian and W.-H. Zhu, J. Am. Chem. Soc., 2015, 137, 14055 CrossRef CAS PubMed.
- C. A. Echeverry-Gonzalez, A. Ortiz and B. Insuasty, New J. Chem., 2019, 43, 8781 RSC.
- J.-M. Ji, S. H. Kim, H. Zhou, C. H. Kim and H. K. Kim, ACS Appl. Mater. Interfaces, 2019, 11(27), 24067 CrossRef CAS PubMed.
- Y. Kurumisawa, T. Higashino, S. Nimura, Y. Tsuji, H. Iiyama and H. Imahori, J. Am. Chem. Soc., 2019, 141(25), 9910 CrossRef CAS PubMed.
- L. Veronese, E. Q. Procopio, T. Moehl, M. Panigati, K. Nonomura and A. Hagfeldt, Phys. Chem. Chem. Phys., 2019, 21, 7534 RSC.
- S. Mathew, A. Yella, P. Gao, R. Humphry-Baker, B. F. E. Curchod, N. Ashari-Astani, I. Tavernelli, U. Rothlisberger and M. Grätzel, Nat. Chem., 2014, 6, 242 CrossRef CAS PubMed.
- K. Kakiage, Y. Aoyama, T. Yano, K. Oya, J. Fujisawa and M. Hanaya, Chem. Commun., 2015, 51, 15894 RSC.
- H. Choi, S. Kim, S. O. Kang, J. Ko, M.-S. Kang, J. N. Clifford, A. Forneli, E. Palomares, M. K. Nazeeruddin and M. Grätzel, Angew. Chem., Int. Ed., 2008, 47, 8259 CrossRef CAS PubMed.
- D. D. Babu, R. Su, A. El-Shafei and A. V. Adhikari, RSC Adv., 2016, 6, 30205 RSC.
- K. Zeng, Y. Lu, W. Tang, S. Zhao, Q. Liu, W. Zhu, H. Tian and Y. Xie, Chem. Sci., 2019, 10, 2186 RSC.
- C. Li, L. Luo, D. Wu, R. Jiang, J. Lan, R. Wang, L. Huang, S. Yang and J. You, J. Mater. Chem. A, 2016, 4, 11829 RSC.
- J. Liu, B. Liu, Y. Tang, W. Zhang, W. Wu, Y. Xie and W.-H. Zhu, J. Mater. Chem. C, 2015, 3, 11144 RSC.
- X. Sun, Y. Wang, X. Li, H. Ågren, W. Zhu, H. Tian and Y. Xie, Chem. Commun., 2014, 50, 15609 RSC.
- G. D. Sharma, S. P. Singh, R. Kurchania and R. J. Ball, RSC Adv., 2013, 3, 6036 RSC.
- C.-W. Lee, H.-P. Lu, C.-M. Lan, Y.-L. Huang, Y.-R. Liang, W.-N. Yen, Y.-C. Liu, Y.-S. Lin, E. W.-G. Diau and C.-Y. Yeh, Chem.–Eur. J., 2009, 15, 1403 CrossRef CAS PubMed.
- Y. J. Liu, N. Xiang, X. M. Feng, P. Shen, W. P. Zhou, C. Weng, B. Zhao and S. T. Tan, Chem. Commun., 2009, 2499 RSC.
- C.-P. Hsieh, H.-P. Lu, C.-L. Chiu, C.-W. Lee, S.-H. Chuang, C.-L. Mai, W.-N. Yen, S.-J. Hsu, E. W. G. Diau and C. Y. Yeh, J. Mater. Chem., 2010, 20, 1127 RSC.
- C.-L. Wang, J.-Y. Hu, C.-H. Wu, H.-H. Kuo, Y.-C. Chang, Z.-J. Lan, H.-P. Wu, E. W.-G. Diau and C.-Y. Lin, Energy Environ. Sci., 2014, 7, 1392 RSC.
- H.-L. Jia, M.-D. Zhang, W. Yan, X.-H. Ju and H.-G. Zheng, J. Mater. Chem. A, 2016, 4, 11782 RSC.
- Y. Liu, X. Zhang, C. Li, Y. Tian, F. Zhang, Y. Wang, W. Wu and B. Liu, J. Phys. Chem. C, 2019, 123, 13531 CrossRef CAS.
- H. Zhou, J.-M. Ji, S. H. Kang, M. S. Kim, H. S. Lee, C. H. Kim and H. K. Kim, J. Mater. Chem. C, 2019, 7, 2843 RSC.
- Y. Kubota, K. Kimura, J. Jin, K. Manseki, K. Funabiki and M. Matsui, New J. Chem., 2019, 43, 1156 RSC.
- C.-J. Lin, W.-Y. Yu and S.-H. Chien, J. Mater. Chem., 2010, 20, 1073 RSC.
- U. Mehmood, I. A. Hussein, K. Harrabi, N. Tabete and G. R. Berdiyorovf, RSC Adv., 2016, 6, 7897 RSC.
- W. Zhang, Y. Wu, H. Zhu, Q. Chai, J. Liu, H. Li, X. Song and W.-H. Zhu, ACS Appl. Mater. Interfaces, 2015, 7, 26802 CrossRef CAS PubMed.
- K. Mahmood and H. J. Sung, J. Mater. Chem. A, 2014, 2, 5408 RSC.
- K. Pei, Y. Wu, A. Islam, Q. Zhang, L. Han, H. Tian and W. Zhu, ACS Appl. Mater. Interfaces, 2013, 5, 4986 CrossRef CAS PubMed.
- A. Atli, A. Atilgan and A. Yildiz, Sol. Energy, 2018, 173, 752 CrossRef CAS.
- J.-S. Ni, W.-S. Kao, H.-J. Chou and J. T. Lin, ACS Appl. Mater. Interfaces, 2014, 6, 22612 CrossRef CAS PubMed.
- A. Baheti, K. R. J. Thomas, C. P. Lee, C. T. Li and K. C. Ho, J. Mater. Chem. A, 2014, 2, 5766 RSC.
Footnote |
† Electronic supplementary information (ESI) available: Synthesis details and characterization data; details of all physical characterization. See DOI: 10.1039/c9se00810a |
|
This journal is © The Royal Society of Chemistry 2020 |
Click here to see how this site uses Cookies. View our privacy policy here.