DOI:
10.1039/D0BM01804J
(Review Article)
Biomater. Sci., 2021,
9, 2339-2361
Biomaterials for protein delivery for complex tissue healing responses
Received
23rd October 2020
, Accepted 30th December 2020
First published on 12th January 2021
Abstract
Tissue repair requires a complex cascade of events mediated by a variety of cells, proteins, and matrix molecules; however, the healing cascade can be easily disrupted by numerous factors, resulting in impaired tissue regeneration. Recent advances in biomaterials for tissue regeneration have increased the ability to tailor the delivery of proteins and other biomolecules to injury sites to restore normal healing cascades and stimulate robust tissue repair. In this review, we discuss the evolution of the field toward creating biomaterials that precisely control protein delivery to stimulate tissue regeneration, with a focus on addressing complex and dynamic injury environments. We highlight biomaterials that leverage different mechanisms to deliver and present proteins involved in healing cascades, tissue targeting and mimicking strategies, materials that can be triggered by environmental cues, and integrated strategies that combine multiple biomaterial properties to improve protein delivery. Improvements in biomaterial design to address complex injury environments will expand our understanding of both normal and aberrant tissue repair processes and ultimately provide a better standard of patient care.
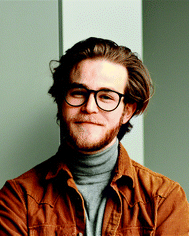 Jonathan Dorogin | Jonathan Dorogin is a Ph.D. student in the joint bioengineering program at University of Oregon and Oregon State University, working under the supervision of Professor Marian Hettiaratchi at the Knight Campus for Accelerating Scientific Impact. He received a Bachelor of Applied Science degree in Nanotechnology Engineering from the University of Waterloo and a Master of Biomedical Engineering degree from McMaster University. He is also a co-founder of an on-demand delivery start-up focused on reducing the negative side-effects of inefficient chemotherapy protocols. His current research focuses on developing biomaterials for the spatiotemporal control of multi-protein delivery for various wound healing applications. |
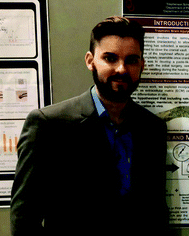 Jakob M. Townsend | Jakob Townsend received his Bachelor of Science degree in Bioengineering from Oregon State University in 2014 and Ph.D. in Biomedical Engineering from the University of Oklahoma in 2017. He then continued as a postdoctoral researcher in Professor Michael Detamore's group at the University of Oklahoma before joining Professor Marian Hettiaratchi's group at the University of Oregon. He also founded a start-up focused on developing biomaterials for craniofacial bone regeneration. His research has focused on biomaterials for bone and trachea regenerative medicine. |
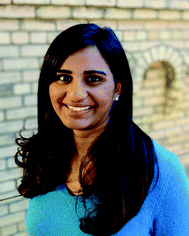 Marian H. Hettiaratchi | Marian Hettiaratchi is an Assistant Professor at the University of Oregon's Knight Campus for Accelerating Scientific Impact. She received her B.Sc. in Chemical Engineering from the University of Calgary and a Ph.D. in Biomedical Engineering from the Georgia Institute of Technology and Emory University. She completed postdoctoral training at the University of Toronto. Dr Hettiaratchi has received doctoral and postdoctoral fellowships from the Natural Sciences and Engineering Research Council of Canada and a Philanthropic Educational Organization Scholar Award. Her lab develops biomaterials for protein delivery using an interdisciplinary approach that leverages expertise in chemical engineering, polymer chemistry, and molecular biology. |
Introduction
Tissue injuries such as critically-sized bone defects, cartilage injuries, spinal cord injuries, and chronic skin ulcers pose major clinical challenges because they do not readily heal without intervention. Functional tissue regeneration, the process of cell proliferation and tissue repair, is governed by complex mechanisms that involve a carefully coordinated cascade of cytokines, growth factors, metabolites, and multiple cell types that is difficult to effectively mimic with clinical therapies.1 In the case of non-healing tissue defects, the normal healing paradigm (inflammation, proliferation, and remodelling) is impaired or ceases to progress due to ineffective protein signalling caused by poor tissue revascularization, injury severity, and systemic factors such as age and chronic disease.2 The use of implantable biomaterials to provide the missing building blocks and bridge the gap in the tissue repair process has been a major focus in the field of tissue engineering. Identification of biomaterials that can effectively mimic the healing process and restore missing protein signalling observed in disrupted tissue repair may overcome limitations of current clinically-approved treatment strategies and permit robust tissue regeneration.3
The role of proteins in the healing cascade
A major driving factor of successful healing is the appropriately timed presentation of proteins involved in the healing cascade.4 Although not fully understood for all tissue types, numerous proteins guide the phases of wound healing (e.g., inflammation, proliferation, and remodelling), including cytokines involved in cell recruitment and growth factors to promote cell differentiation and extracellular matrix (ECM) deposition.5 Altogether, the wound healing paradigm involves both the breakdown of cells and tissues due to damage as well as cell proliferation leading to regeneration and remodelling. Tissue healing, like wound healing, consists of both cellular breakdown and repair, but more broadly encompasses both wounds that occur due to distinct injury or trauma and events involved in maintaining tissue homeostasis. For instance, bone fractures and skin injuries are classified as wounds, but the gradual erosion of cartilage or demyelination of an axon are not necessarily caused by specific traumatic events. As such, repair of bone fractures and other types of traumatic injuries may be classified as both wound and tissue healing, but repair in response to gradual tissue degeneration such as axon demyelination and cartilage breakdown would only be considered tissue healing. Fig. 1 depicts the distinctions between tissue regeneration, wound healing, and tissue healing, as we have described them in this review.
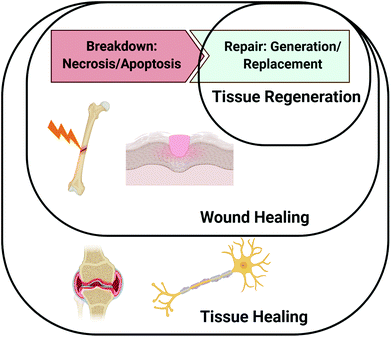 |
| Fig. 1 Distinction between wound healing, tissue healing, and tissue regeneration. The wound healing paradigm involves both the breakdown of cells and tissues due to trauma or injury as well as cell proliferation leading to regeneration and remodelling. Tissue healing broadly encompasses both wounds that occur due to distinct injury or trauma and events involved in maintaining tissue homeostasis. Tissue regeneration specifically refers to processes involved in the repair and replacement of damaged tissues. Figure created with BioRender.com. | |
The healing response is initiated by damage to the tissue, which causes ruptured blood vessels to fill the injury site with blood and clotting factors that create an ECM-rich hematoma.6 The hypoxic environment of the hematoma promotes the secretion of hypoxia inducible factor-1α (HIF-1α), which plays an important role in vascularization and angiogenesis. Platelet activation and degranulation within the newly formed ECM promotes the release of inflammatory mediators such as platelet-derived growth factor (PDGF), interleukin 1 (IL-1), IL-6, IL-8, tumor necrosis factor-α (TNFα), and transforming growth factor β1 (TGF-β1).7 The released cytokines act to recruit additional neutrophils, which amplify the signal, and eventually promote the release of monocyte chemoattractant protein-1 (MCP-1) to attract monocytes and T-lymphocytes.8 During the proliferative phase, progenitor cells, epithelial cells, and mesenchymal stem cells are recruited by the release of a multitude of morphogenic factors, such as fibroblast growth factors (FGFs), insulin-like growth factors (IGFs), bone morphogenetic proteins (BMPs), vascular endothelial growth factor (VEGF), and transforming growth factors (TGF-βs).9 These cells deposit ECM molecules, such as collagen, which are ultimately replaced and remodelled to resemble the ECM composition of healthy tissue. The numerous cell types and array of protein signals that participate in the healing process highlight its complex nature and the need for precisely timed events to achieve successful tissue regeneration.
Fig. 2 depicts the proteins involved in bone repair, as an example of a normal healing cascade. In bone repair, major morphogenic factors include angiogenic factors (Ang 1, FGFs, VEGF), which promote vascular network repair, BMPs, which promote osteogenic cell differentiation and mineralization, and RANK and RANKL, which promote bone remodelling. As the soft cartilaginous callus mineralizes and is remodelled, type II and type X collagen are replaced by type I collagen, the predominant ECM molecule in bone.
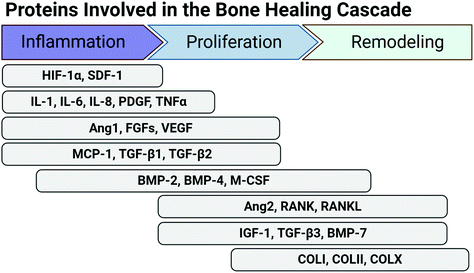 |
| Fig. 2 Proteins involved in the normal healing cascade specific to bone tissue repair. Successful tissue repair requires the coordinated presentation of multiple proteins. This timeline highlights proteins involved in the healing cascade specific to bone. Proteins are grouped based on time of presentation after injury and approximate phase of healing (inflammation, proliferation, remodelling). Ang: angiopoietin; BMP: bone morphogenetic protein; COL: collagen; FGFs: fibroblast growth factors; HIF: hypoxia inducible factor; IGF: insulin-like growth factor; IL: interleukin; MCP: monocyte chemoattractant protein; M-CSF: macrophage colony-stimulating factor; PDGF: platelet-derived growth factor; RANK: receptor activator of nuclear factor κB; RANKL: receptor activator of nuclear factor κB ligand; SDF: stromal cell-derived factor; TGF: transforming growth factor; TNF: tumor necrosis factor; VEGF: vascular endothelial growth factor. Figure created with BioRender.com. | |
Disruption of the normal tissue repair process can be caused by a variety of factors (e.g. severity of injury, systemic disease) and can drastically alter the protein cascade, culminating in tissue injuries that do not heal (Fig. 3). Tissues with low intrinsic regenerative capacity, such as cardiac tissue, neural tissue, and cartilage, often heal poorly, resulting in impaired function. However, tissues that normally heal readily, such as bone and skin, can also exhibit inadequate regeneration.
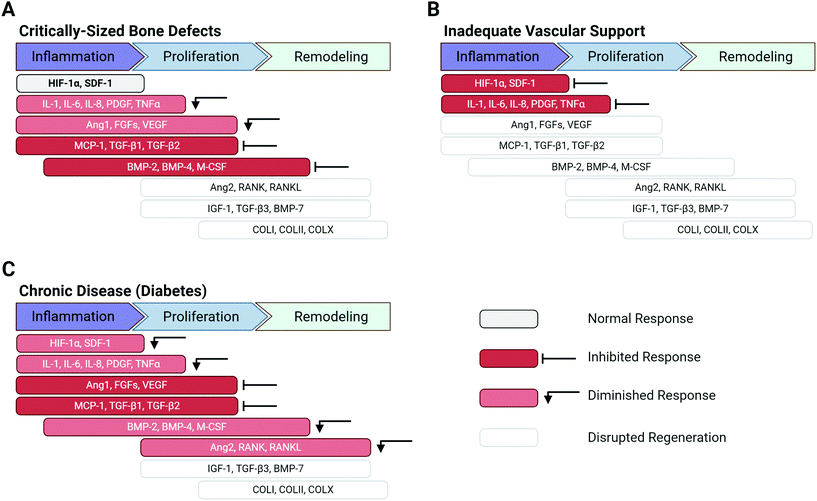 |
| Fig. 3 Disruption of proteins involved in the bone healing cascade impairs tissue regeneration. A number of complicating factors can dysregulate the normal healing response leading to delayed or incomplete tissue regeneration. (A) In the specific case of bone regeneration, severe, critically-sized defects reduce levels of inflammatory cytokines and angiogenic proteins and inhibit expression of osteogenic proteins. (B) Limited vascular network support inhibits the release of initial chemotactic and inflammatory mediators. (C) Chronic, systemic diseases, such as diabetes, disrupt the expression of numerous inflammatory cytokines and growth factors, while significantly decreasing levels of angiogenic proteins. The colours of the proteins indicate their response: normal response (dark grey, bold font), inhibited response (red), diminished response (light red), disrupted regeneration (light grey, light font). Ang: angiopoietin; BMP: bone morphogenetic protein; COL: collagen; FGFs: fibroblast growth factors; HIF: hypoxia inducible factor; IGF: insulin-like growth factor; IL: interleukin; MCP: monocyte chemoattractant protein; M-CSF: macrophage colony-stimulating factor; PDGF: platelet-derived growth factor; RANK: receptor activator of nuclear factor κB; RANKL: receptor activator of nuclear factor κB ligand; SDF: stromal cell-derived factor; TGF: transforming growth factor; TNF: tumor necrosis factor; VEGF: vascular endothelial growth factor. Figure created with BioRender.com. | |
The severity of the injury is a key factor that can disrupt the normal healing process. Critically-sized defects are tissue defects that are too large to naturally heal without additional intervention.10–12 In the case of bone, critically-sized defects can cause inadequate hematoma formation or lack of sufficient immune and progenitor cell infiltration, resulting in fracture non-union or delayed union.6 Critically-sized bone defects often display dysregulated inflammatory protein expression and decreased presence of vital cytokines such as PDGF, VEGF, and TGF-β1, ultimately inhibiting downstream BMP expression and bone formation (Fig. 3A).13,14 Significant vascular network damage caused by severe bone injury can prevent initiation of the bone repair process altogether, as the initial platelet plug formation and release of inflammatory mediators (e.g. HIF-1α, PDGF, and interleukins) do not occur (Fig. 3B).5
Systemic factors affecting the patient may also diminish the intrinsic regenerative capabilities of injured tissues and the overall healing process. Chronic, systemic diseases such as diabetes can disrupt the normal wound healing cascade by formation of prolonged hypoxic wounds and an impaired immune response.15 Hypoxic wounds are generally addressed in the normal protein cascade by HIF-1α release; however, in diabetic patients, tissue vascularity is impaired by insufficient levels of growth factors such as HIF-1α and VEGF, which would normally promote vascularization and angiogenesis.2 In bone repair, diabetes can diminish the initial inflammatory response (e.g. TNFα, PDGF, stromal derived factor-1α (SDF-1α), interleukins) and inhibit angiogenic factor secretion (Ang 1, VEGF, FGFs) – both of which can hinder overall bone formation and maturation (Fig. 3C). Taken together, these example scenarios of impaired bone regeneration illustrate that many factors can cause the dysregulation of proteins in the healing cascade and can disrupt the entire process of tissue regeneration. Similarly, aberrant protein expression caused by severe injuries, poor vascular network support, and chronic diseases can also result in impaired healing in other tissues in the body.
Tunable biomaterial properties to enhance tissue healing
Restoration of critical proteins involved in healing cascades, such as immunomodulatory cytokines, morphogenic factors, and ECM molecules, may provide the missing link to achieving functional tissue regeneration in non-healing tissue injuries. Thus, a critical challenge that the field of tissue engineering aims to address is the need for the precise, temporal delivery of proteins. By better understanding how the protein cascade can be disrupted in various situations, new biomaterials can be engineered to effectively augment the healing process.
A number of biomaterials have been developed as therapeutic strategies to overcome disrupted healing cascades and stimulate the repair of injured and diseased tissues. Hydrogels have been extensively investigated for tissue regeneration applications due to their innate hydrophilic characteristic, which makes them suitable delivery vehicles for cells and proteins.16,17 Hydrogels are 3D networks consisting of various natural and/or synthetic polymers, including alginate,18 gelatin,19 hyaluronic acid,20 polyethylene glycol (PEG),21 and devitalized or decellularized ECM.22 Synthetic polymer hydrogels are highly reproducible and can be extensively functionalized for modular addition of proteins, cells, and other bioactive ligands; however, since synthetic polymers are typically biologically inert, multiple functional groups may be required to achieve a desired biological response.23 In contrast, natural polymer hydrogels are biocompatible, biodegradable, and have cell-adhesive ligands and natural affinities for proteins, but are less reproducible due to batch-to-batch variability that affects material tunability.24
Hydrogels typically have highly tunable physical characteristics that influence their behaviour in the body, such as crosslinking density, polymer molecular weight, mesh size, and stiffness.25 These physical characteristics can significantly impact therapeutic protein delivery and tissue regeneration. Common protein delivery strategies from hydrogels include encapsulation in a degradable polymer or immobilization using chemical or physical interactions.26 Encapsulation methods where the protein does not interact with the material (e.g. minimal non-covalent interactions) rely on the crosslinking density and associated pore size of a hydrogel to control protein diffusion from the material and can impede cell migration if the pore size is too small.27 Protein encapsulation typically provides limited controlled protein release and subsequently exhibits burst release kinetics.28 Alternatively, protein immobilization strategies using chemical or physical interactions can drastically improve protein retention, stability, and enable controlled delivery.29 Polymer molecular weight can directly influence cell response due to cell-material interactions and alter the degradation profile of the hydrogel, which can also affect protein delivery and presentation.30 Hydrogel stiffness can influence cell behaviour due to cell mechanotransduction and alter interactions with the surrounding tissue, especially if the defect is load bearing.31
Engineering biomaterials that can adapt to changes in the complex injury environment during tissue repair may augment the healing process and overcome dysregulation of protein presentation in the healing cascade. This review highlights the evolution of the field toward creating biomaterials that precisely control protein delivery and stimulate tissue regeneration using a variety of methods, with a focus on addressing complex and dynamic injury environments. The framework of the following sections explores biomaterial strategies that deliver and present proteins involved in healing cascades, tissue targeting and mimicking strategies, materials that can be triggered by environmental cues, and integrated strategies that leverage multiple biomaterial properties to improve protein delivery. Recommendations for future work and strategies to accelerate biomaterial development conclude the review. Improvements in biomaterial design to better address complex injury environments will ultimately provide a better standard of patient care and expand our understanding of both normal and aberrant tissue repair processes.
Biomaterial strategies for protein delivery and presentation
Given the importance of coordinated protein expression in tissue repair, a common approach to restore normal healing mechanisms in injured tissues is to deliver exogenous, recombinant proteins to the tissue injury site to introduce or augment a missing biological function. Growth factors that stimulate cell differentiation, vessel formation, and ECM deposition, such as VEGF, BMPs, and FGFs, are often chosen for delivery. The materials chosen as protein delivery vehicles can also significantly impact the efficacy of the treatment by influencing both temporal and spatial protein presentation through interactions between the protein and biomaterial matrix.32 The chemical and physical properties of biomaterials can be manipulated to control the presentation of various proteins. Extended local presentation of bioactive proteins within injury sites is necessary for lengthy wound healing processes, and numerous biomaterial strategies have been developed to ensure sufficient local protein retention within injury sites (Fig. 4).
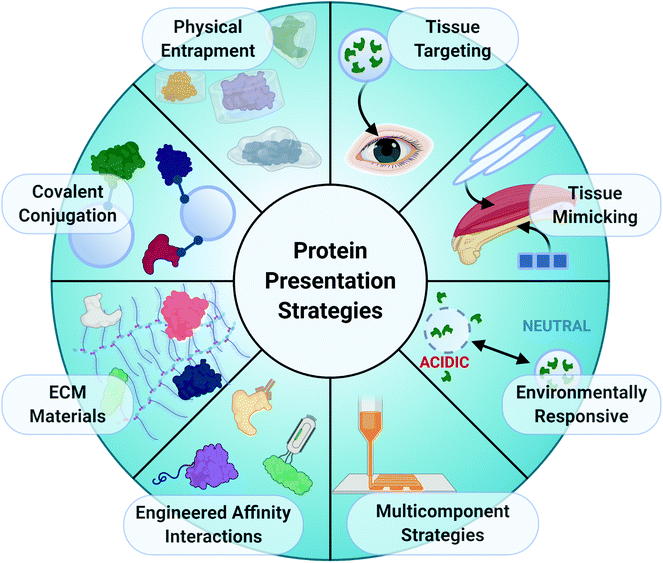 |
| Fig. 4 Overview of biomaterial strategies for protein presentation. The left side of the wheel identifies several biomaterial strategies for protein delivery and presentation, including the physical entrapment of proteins in hydrogels, covalent conjugation of proteins to materials, use of ECM-based materials with natural affinities for proteins, and engineered affinity interactions using antibodies, peptides, and phage display platforms. The strategies on the right side of the wheel can be broadly categorized as strategies to improve tissue integration, including tissue targeting, tissue mimicking, and environmentally-responsive materials. Multicomponent strategies combine bioengineering techniques, such as 3D printing, with other protein presentation strategies. Figure created with BioRender.com. | |
Physical encapsulation
Physical encapsulation of proteins, which does not rely on non-covalent or covalent interactions, typically controls protein release through porosity of the matrix (i.e. mesh size) and material degradation kinetics.33 Physical entrapment of proteins can provide predictable, reproducible protein release in vitro, where the variables affecting protein release can be easily controlled. Hydrogel mesh size and degradation rate are both controlled by polymer molecular weight. In a study using PEG hydrogels, the release of FGF-2 and bovine serum albumin (BSA) was accelerated in hydrogels made from higher molecular weight PEG polymers (4 kDa), which had a larger mesh size and degraded faster than their smaller counterparts (2.5 kDa).34 Protein release rate following physical encapsulation in a hydrogel is inversely proportional to the size of the payload and directly proportional to the pore or mesh size of the biomaterial.35,36 The Kloxin group demonstrated that mesh size of a hydrogel could be used to predict release and retention of proteins of different sizes.36 Using a PEG-based library of materials, they systematically demonstrated that the elasticity and swelling of the material directly correlated to mesh size, and that mesh size, in turn, impacted the release of a range of proteins, including aprotinin (7 kDa), myoglobin (17 kDa), BSA (66 kDa), lactoferrin (77 kDa), and thyroglobulin (663 kDa). Block copolymers can also be manipulated for controlled protein release by modifying the ratio of comonomers, as is typically done with polyesters.37,38 For example, increasing the ratio of lactic acid to glycolic acid (LA
:
GA ratio) increases hydrophobicity and reduces the rate of hydrolysis, resulting in nanoparticles that degrade more slowly.39,40 However, protein activity may potentially be affected by the choice of encapsulating polymer as harsh organic solvents may be required for fabricating polymeric nanoparticles.
Despite the ability to tune the release kinetics of encapsulated proteins by changing material properties, physical encapsulation of proteins in biomaterials can often lead to burst release pharmacokinetics in vivo, resulting in rapid diffusion of proteins away from the injury site and reduced therapeutic efficacy.41 PLGA nanoparticles are commonly used for protein delivery, but have a tendency to exhibit burst release of molecular cargo. Burst release kinetics can be mitigated by encapsulating smaller nanocarriers in larger bulk hydrogels to slow protein diffusion following release from the nanocarrier.42–44 Pakulska et al. encapsulated stromal-derived factor-1α (SDF-1α), neurotrophin-3 (NT-3), and brain-derived neurotrophic factor (BDNF) in PLGA nanoparticles which were then suspended in a bulk hyaluronic acid and methylcellulose hydrogel.44 Similarly, Mancipe Castro et al. entrapped rhodamine B in PLGA nanoparticles and suspended them in PEG microspheres.43 Both studies demonstrated that the dual-layer system prevented burst release from PLGA nanoparticles as they degraded. Pakulska et al. further noted that the method of drug entrapment within the composite material (i.e. encapsulation or adsorption to PLGA) did not significantly impact the release kinetics of the payload.
Covalent conjugation
Covalently tethering proteins to a biomaterial through irreversible chemical bonds provides increased spatiotemporal control over protein presentation in vivo compared to physical encapsulation.45,46 Covalent conjugation strategies are valuable in situations where long-term protein presentation is necessary to elicit a sustained cellular response in vivo. Protein immobilization is often achieved using amine-reactive chemistry, which requires an amine group available for nucleophilic attack, or carboxyl-reactive chemistry, which requires a carboxyl group for electrophilic attack. Proteins can be immobilized on polymers such as poly(lactic-co-glycolic) acid (PLGA) via carbodiimide crosslinker chemistry, using N-hydroxysuccinimide (NHS) and 1-ethyl-3-(3-dimethylaminopropyl) carbodiimide (EDC) coupling.47 Building upon this approach, Wang et al. recently demonstrated enhanced retention of VEGF on mussel-adhesion protein (MAP)-coated stents through carbodiimide chemistry.48 MAP, which is rich in 3,4-dihydroxy-L-phenylalanine (Dopa), has immense cell-adhesive capacity.49 MAP substrates coated onto a silicon wafer were dip-coated in VEGF, which was immobilized using EDC/NHS chemistry. Substrates containing crosslinked and non-crosslinked MAP, as well as immobilized and free VEGF were tested. The tethered VEGF maintained its bioactivity, resulting in increased spreading of endothelial cells, while crosslinking of the MAP increased its retention on the stent.48
Although covalent conjugation of proteins to materials prolongs local protein presentation within an implantation site, this strategy may reduce overall protein bioactivity by causing protein denaturation during processing or interfering with the ability of proteins to interact with cell integrins and receptors.50–55 For example, modification of collagen I with EDC reduced cell adhesion by inhibiting cationic cell interactions with collagen's integrin binding domains.50 Moreover, several studies have demonstrated that non-specific, stochastic conjugation of primary amines in proteins using NHS esters can significantly decrease protein bioactivity.56,57 As a result, site-specific protein modification has recently gained popularity as a method to install functional groups on proteins without decreasing protein bioactivity. Site-specific labelling techniques enable rational placement of functional groups, while avoiding enzymatic and receptor binding sites on proteins. For an in-depth review of methods to achieve site-specific protein modification, we recommend the recent publication of Shadish et al.58
Extracellular matrix-based materials
ECM molecules that naturally occur within tissues, including glycosaminoglycans (GAGs), collagens, and fibronectin, play a significant role in modulating protein presentation by retaining and releasing a variety of proteins. Morphogenic proteins involved in developmental processes, such as BMPs, TGFs, IGFs, and FGFs, must be presented with precise spatiotemporal control to enable patterning of the developing embryo and thus retain the ability to interact with multiple ECM molecules.59–61 The many cationic domains of these proteins, termed “heparin binding proteins,” interact with the anionic sulphate groups on a variety of GAGs, but often interact with higher affinity to heparin due to its increased degree of sulphation compared to other GAGs.62
ECM-based materials use electrostatic payload-vehicle interactions that mimic the natural affinity interactions between proteins and ECM in the body to provide controlled protein delivery. These electrostatic interactions enhance retention of charged biomolecules compared to purely physical encapsulation with minimal protein–material interactions.63 Heparin binding proteins bind reversibly to biomaterials containing sulphated GAGs (heparin10,64,65 and chondroitin sulphate66,67), non-sulphated GAGs such as hyaluronic acid,68–70 other natural polysaccharides derived from plants and animals (alginate71 and chitosan72,73), and combinations of these polymers.74–76 Sulphated GAG-based biomaterials interact with a variety of protein partners (heparan sulphate to FGF-2, VEGF-A165, and SDF-1α;65,77 heparin to BMP-2;78,79 chondroitin sulphate to TGF-β1 and BMPs66). The abundance of heparin and heparan sulphate in both normal and injured tissues strongly suggests that supplementation with GAG-based biomaterials may improve the efficacy of a protein therapy.65,80
The protein–material affinity interactions of ECM-based biomaterials were traditionally thought to be difficult to modify, as they involve complex interactions between the repeating units on the ECM molecules and specific tertiary structures of the protein.62 However, the protein binding properties of GAG-based, electrostatically-driven biomaterials can be tuned by modifying their sulphate content, which changes the electrostatic interactions between the protein and material and subsequently alters protein release from the material.70,77,81–83 Sulphating non-sulphated molecules such as hyaluronic acid and alginate increases protein retention, while desulphating GAGs such as heparin increases protein release. Sulphation of hyaluronic acid can also slow biomaterial degradation by reducing the availability of octosaccharides necessary for effective degradation by the enzyme hyaluronidase. Limasale et al. systematically manipulated the sulphation levels of heparin to assess the effect of sulphation on the diffusion rates of proteins from a composite PEG/heparin-based hydrogel (Fig. 5). They demonstrated precise control over the binding of VEGF-165 to the biomaterial with higher degrees of sulphation leading to increased protein retention. This strategy provided negligible control over the release kinetics of epidermal growth factor (EGF) and VEGF-121, the non-heparin binding isoform of VEGF, due to their low affinities for heparin, while additional heparin-binding proteins such as SDF-1α demonstrated similar, sustained release kinetics to VEGF-165. Although ECM-based biomaterials with affinities for proteins may improve the local retention of specific proteins within injury sites, this does not always translate to improved therapeutic outcomes.64 Andrews et al. demonstrated prolonged in vitro retention of BMP-2 in a sulphated GAG-based (chondroitin sulphate) scaffold compared to a collagen scaffold; yet, the overall impact on bone healing was comparable between the scaffolds after 12 weeks.66 Since protein release is dependent on both the overall degradation rate of the scaffold and the strength of the electrostatic interactions between the protein and material, the slower-degrading collagen matrix with lower BMP-2 affinity likely resulted in similar protein release to the faster-degrading, GAG-based scaffold.
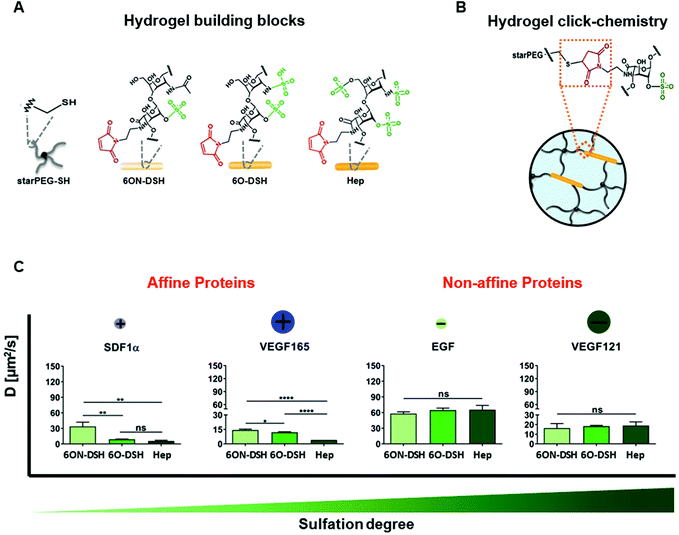 |
| Fig. 5 Modulation of heparin sulphation degree differentially affects diffusion of proteins from heparin-based hydrogels. (A) Hydrogel building blocks consist of thiol-functionalized starPEG (starPEG-SH) and maleimide-functionalized heparin (Hep) or heparin derivatives with selective degrees of sulphation. (B) Click chemistry creates covalent bonds between the starPEG-SH and maleimide-functionalized heparin and heparin derivatives. (C) Effect of heparin sulphation pattern on the diffusion of heparin affine and nonaffine proteins with heparin-based hydrogels. Heparin-binding proteins SDF-1α and VEGF-165 exhibited slower diffusion in hydrogels containing heparin with a higher degree of sulphation. Conversely, the diffusion of non-heparin-binding proteins EGF and VEGF121 was not affected by degree of heparin sulphation. Diffusion of the proteins through the heparin-based hydrogels was measured using fluorescence recovery after photobleaching (FRAP). “ns” stands for not significant, *p < 0.05, **p < 0.01, ***p < 0.001, and ****p < 0.0001. Reproduced from ref. 77 with permission from Wiley-VCH, copyright 2020. | |
Since many proteins interact with ECM molecules, multiple proteins may interact with the same biomaterial, which may be advantageous for developing strategies to deliver multiple ECM-binding proteins.84,85 However, the promiscuous binding of heparin and other ECM molecules to a variety of heparin binding proteins can also be disruptive when these biomaterials are delivered in vivo and non-specific, competitive binding to abundant serum proteins can result in accelerated payload release.86 Thus, caution should be exercised when employing ECM-based biomaterials, which could unintentionally bind additional proteins involved in other cellular processes, resulting in off-target effects and unpredictable protein release in vivo. Improving the specificity of protein–material interactions may improve the ability of biomaterials to more precisely control protein delivery in vivo and target specific phases of the healing cascade.
Engineered protein–material affinities
More sophisticated affinity interactions have been engineered between proteins and materials to overcome the limited specificity and tunability of natural ECM-based materials. Antibodies specific to proteins of interest can be covalently conjugated87 or physically adsorbed to biomaterials.48,88 Alternatively, high affinity molecules can be developed to achieve extremely specific protein–material interactions and can be produced inexpensively compared to antibodies.89–91 Some notable strategies include peptides that directly bind to the protein of interest,88,90 the synthesis of fusion proteins, consisting of a peptide- or ECM-binding domain and functional protein domain,91–96 and DNA aptamers that contain a protein sequestering component and a cell adhesive component that provides localized cargo release.97,98 A well-characterized fusion domain used to control protein delivery is the Src homology 3 (SH3) domain, which naturally binds to a variety of short peptide sequences with affinities that enable sustained protein release. The release of SH3 fusion proteins from hydrogels can be mediated by immobilizing high-affinity or low-affinity SH3 binding peptides onto the polymer network. This strategy has been extensively studied for the delivery of fusions of FGF-2,99 chondroitinase ABC,57,100,101 ciliary neurotrophic factor,95 and fibronectin,102 in both in vitro and in vivo applications.
An interesting alternative approach to enhance the therapeutic efficacy of exogenous growth factors is to increase their binding affinity to the target cell population instead of improving their retention within the delivery vehicle. Mochizuki et al. integrated a syndecan binding peptide into VEGF and the PDGF dimer PDGF-BB.32 The transmembrane proteoglycan syndecan demonstrated a higher affinity for growth factors expressed with syndecan-binding domains compared to unmodified growth factors and BSA, enabling higher affinity interactions between the cells and growth factors. This modification resulted in tonic signalling (i.e. continuous, low-intensity signalling) between PDGF and VEGF and their receptors, leading to extended downstream Akt and ERK phosphorylation and enhanced bone regeneration. Tonic signalling is beneficial in the wound healing cascade, as it overcomes the short half-life and loss of bioactivity commonly associated with exogenous protein delivery, though the inability to turn off signalling may cause adverse effects after the natural wound healing process has concluded.103
Biomaterials for endogenous protein sequestration
Although protein delivery is a promising strategy to stimulate tissue repair, poor control over protein release kinetics in vivo can reduce the effective protein dose within the injury site, necessitating the use of supraphysiological protein doses to stimulate lengthy healing processes. “Empty” biomaterials can be delivered to sequester endogenous proteins and biomolecules that are involved in tissue repair but are usually present in the body in concentrations that are too low to improve the healing response. This strategy creates a biomolecule depot within the site of interest and mimics how ECM molecules in tissues naturally sequester and present proteins to create morphogen gradients during development and regeneration. Heparin-based and heparin-sequestering biomaterials have been used to amplify the effects of heparin-binding growth factors, such as FGF-2 and IGF binding proteins, within in vitro cell culture environments.104,105 Crispim et al. used protein-binding peptides to sequester and present endogenous BMP-2 to promote integration of a ligament graft and endogenous TGF-β1 to improve vascularization and cell recruitment following subcutaneous implantation of polycaprolactone (PCL) films.88,90 PEG-based biomaterials functionalized with 3-(acrylamido)-phenylboronic acid have been developed to sequester adenosine, a potent signalling molecule, within bone injury sites.106 Phenylboronic acids are especially useful in sequestration applications because they form dynamic boronate-ester bonds, allowing them to attract many different biomolecules. This functionalized PEG biomaterial has demonstrated utility in both sequestering endogenous adenosine and delivering exogenous adenosine, resulting in improved fracture healing. Moreover, this strategy is responsive to the dynamic injury environment, as adenosine levels within the biomaterial gradually return back to physiological levels during fracture healing.
Multiple protein delivery
Since healing is a naturally complex process, requiring many bioactive components, the delivery of multiple proteins involved in tissue repair can lead to more effective healing. Replacing single and dual growth factor delivery strategies with the delivery of more complex combinations of relevant proteins involved in the healing cascade is an emerging focus of protein delivery for tissue repair. Liu et al. co-delivered stem cells with a “cocktail of growth factors” consisting of IGF-1, HGF, and PDGF-BB for myogenesis, NGF and FGF-2 for neurogenesis, and VEGF for angiogenesis in a hydrogel to assess the impact of multi-protein presentation.107 The combinations of proteins delivered achieved myogenesis, neurogenesis and angiogenesis; however, multiple growth factor delivery was not drastically better than the presentation of individual growth factors. Although release of these heparin binding proteins was controlled by a heparin-containing hydrogel, regenerative processes typically require sequential presentation and gradients of proteins. Thus, the results observed in this study may be due to the limited ability of the biomaterial to spatially and temporally control protein release. This suggests that the co-delivery of multiple growth factors for tissue repair may require additional focus on sequence and timing of protein delivery in the future.
Several strategies have been developed to achieve sequential protein delivery using several different mechanisms, including material degradation and logic-based systems. These include the use of multi-layered hydrogels containing different proteins in each layer and nanoparticles fabricated by layer-by-layer deposition of proteins and polymers.108,109 Lee et al. contained and released VEGF and BMP-4 in a sequential manner by encapsulating BMP-4-containing microparticles within a VEGF-containing hydrogel, resulting in a burst release of VEGF and sustained release of BMP-4. Shah et al. developed nanolayered constructs containing physiologically relevant amounts of BMP-2 and PDGF-BB in each layer with controlled degradation kinetics, which led to effective bone regeneration over 2 weeks.110 In these examples, the release kinetics of the proteins were based on material degradation and diffusion kinetics and not in response to specific cues from the surrounding microenvironment. In contrast, logic-based release of proteins can be programmed to respond to microenvironmental cues and external triggers. Several groups have demonstrated control over the release of specific payloads by providing light, pH, or hydrolysis triggers.111–113 These stimuli enable precise, on-demand protein delivery in vitro, but may be challenging to implement in vivo as the triggers mentioned are either endogenous stimuli that require many satisfying conditions throughout the body or difficult to systemically administer.
Ultimately, the choice of protein delivery method depends on the duration of protein presentation required and surrounding environment. We have described a myriad of recent advances available to tune protein retention and release from biomaterials in vivo that can be tailored to a variety of injury types and healing scenarios. Tissue repair that requires long-term protein presentation and precise spatial control may benefit from covalent protein immobilization or protein sequestration with high-affinity protein–material binding interactions. Conversely, the delivery of proteins involved in cell recruitment to injury sites may be more effective if rapidly released from biomaterials to promote long range chemotaxis. Finally, sequential protein delivery may be necessary to mimic the phases of the healing cascade (inflammation, proliferation, and remodelling) and can be achieved using composite materials that respond to different stimuli or layer-by-layer protein/polymer deposition.
Strategies to improve tissue integration
We have described a variety of approaches to enhance protein-biomaterial interactions for effective protein delivery. However, there are many other strategies to improve the efficacy of protein delivery for tissue repair that rely on mediating the interactions between the biomaterials and surrounding tissue, including tissue targeting,114 tissue mimicry,115 and stimuli-responsive materials. These strategies increase the ability of a biomaterial to respond to changes in the surrounding tissue that occur during injury and healing. Broadly, tissue targeting biomaterials leverage interactions with unique biochemical markers of an injury site to localize therapeutics, tissue mimicking biomaterials aim to optimize the biophysical congruency between the therapeutic system and the injury site, and stimuli-responsive materials respond to internal or external stimuli to trigger protein delivery within the effective therapeutic window. There is often overlap between strategies that enhance protein-biomaterial interactions and tissue targeting, mimicry, and stimuli-responsive material strategies, and the integration of these approaches have led to key developments in the field, which are discussed below.
Tissue targeting materials
Biomaterials fabricated with specific ligands or binding partners enable a material delivered locally to bind specifically to a target cell or tissue,114,116 thereby enhancing local material retention. Biomaterials containing target receptors63,114 or synthetic peptides94,116,117 have been fabricated to bind to a variety of tissues. A common approach is to target abundant ECM in the tissue of interest, which has been pursued with both collagen-binding domains for targeting biomaterials to bone,118 solid tumors,119 inflammatory sites,91 and hyaluronic acid-binding domains for targeting tumors120,121 and cartilage.46 Directed evolution platforms such as phage display can also be used to evolve peptides or small protein domains that bind strongly to specific tissues. In phage display, bacteriophages express proteins on their surface, enabling sorting and mutagenesis to yield a protein binding partner with specific binding characteristics.122 Phage display has been used to identify a variety of peptides that bind to cardiac and skeletal muscle123,124 and the mineral component of bone.125,126 Binding peptides for specific biomarkers have also been valuable for targeting other tissues, such as the intra-articular spaces within joints, from which drugs are often rapidly cleared. Mancipe Castro et al. fabricated PEG-based hydrogels with cartilage and synoviocyte targeting peptides, which demonstrated improved bulk material retention in relevant cell lines in vitro and local release of drug in the intra-articular space compared to biomaterials containing non-specific binding domains.43 HA-based hydrogels have demonstrated efficacy for tumor targeting, as many cancer cells overexpress the CD44 glycoprotein that binds to HA for cell attachment.82,127 Ding et al. developed pH-sensitive HA hydrogels that promoted MCF-7 cancer cells binding and endocytosis, allowing the HA hydrogels to disassemble within the low pH environment of the cell (Fig. 6).127 This method enabled the intracellular delivery of the otherwise membrane-impermeable protein saporin, which kills cancer cells by inactivating ribosomes. Overall, the use of tissue targeting strategies results in better directed treatment with outcomes that will continue to improve as the specificity and accuracy of the targets increases.
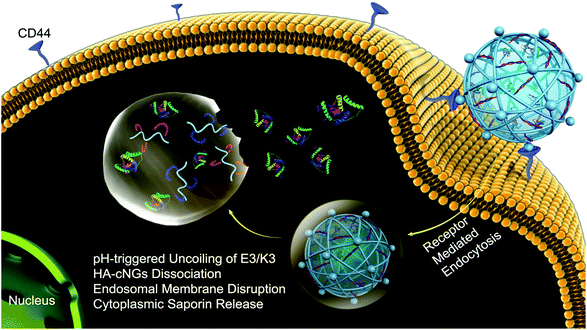 |
| Fig. 6 Delivery of saporin into MCF-7 breast cancer cells using pH-sensitive hyaluronic acid nanogels. MCF-7 cells overexpress CD44, which has a high affinity for hyaluronic acid (HA). This enables binding and subsequent uptake of the coiled-coil peptide-crosslinked HA nanogels (HAcNGs) by MCF-7 cells. Upon endocytosis, HAcNGs dissociate in the acidic conditions of the endosomes of the cell, due to the pH-sensitive dissociation of the crosslinker, releasing saporin. Reproduced from ref. 127 with permission from the American Chemical Society, copyright 2018. | |
Tissue mimicking materials
Biomaterials that aim to delivery proteins to regenerate tissues with complex architectures may better integrate into surrounding tissues if designed to mimic the physical or biochemical attributes of the surrounding native tissue. In doing so, the biomaterial scaffold can temporarily or permanently contribute to the physiology of the tissue. Engineered tissue scaffolds are often designed to match tissue mechanical properties (e.g. stiffness, strength, elasticity), as a mismatch of mechanical properties can lead to stress shielding,128 increased fibrosis,129 or early failure. Alignment of the fibers of the biomaterial is important in the fabrication of biomimetic ligaments88,115,130,131 and muscles,132,133 as effective cell growth and tissue function requires matching both native tissue strength and patterning. For example, Zhang et al. electrospun composite polyethylene terephthalate (PET) and polycaprolactone (PCL) mats and rolled them into cylindrical structures to imitate the braided morphology of the anterior cruciate ligament.115 BMP-7 was then immobilized on the ends on the graft. The combined strategy of an aligned biomaterial and local protein delivery led to improved calcification and integration of the synthetic ligament into the surrounding bone.
Many native tissues contain gradients, such as cartilage and the osteochondral gradient between cartilage and bone.134,135 As a result, biomaterial strategies have been developed to create gradients similar to tissues, including layer-by-layer136–139 and 3D printing140 strategies. Although there are three distinct layers in cartilage (superficial, middle, and deep zones), the challenge is to build many layers on top of one another to seamlessly transition from one zone to the next. Gegg and Yang developed seven unique biomaterial formulations containing different combinations of chondroitin sulphate and gelatin to mimic the different zones of cartilage.137 Cells were allowed to proliferate within the hydrogels for 21 days. Using this approach, the group identified the appropriate combinations of concentrations of the two materials to form the intended zones and stacked them, leading to a well-defined cartilage mimetic material.
Proteins have also been incorporated into gradient-containing materials. 3D-printed scaffolds were fabricated with gradients of BMP-2 and TGF-β3 to mimic the osteochondral interface and stimulate mesenchymal stem cell differentiation towards osteogenic and chondrogenic lineages, respectively.141 In this study, growth factor immobilization improved cell differentiation compared to soluble growth factor addition; however, the use of gradients did not promote cell differentiation. In contrast, osteochondral defects treated with a composite material containing BMP-2 in the subchondral layer and IGF-1 in the chondral layer exhibited robust bone repair, but modest cartilage repair.142 Given that precise protein presentation is necessary to mimic complex tissue interfaces, it is likely that further optimization is needed to create robust osteochondral tissues using gradient protein delivery. As the library of available biomaterials continues to expand, combinations of materials will continue to yield more physiologically relevant tissue mimetics, which will increase our ability to more effectively address site-specific tissue injuries.
Environmentally-responsive materials
Stimuli-responsive materials can change properties based on exposure to endogenous cues present in the surrounding microenvironment, such as pH, temperature, reactive oxygen species, or specific enzymes.143 Integrating components that can be triggered by microenvironmental cues can increase a biomaterial's adaptability to changes in the in vivo environment during tissue repair and provide an additional degree of temporal control over drug/protein release and material behaviour. There are numerous types and applications of stimuli-responsive biomaterials. For an in-depth review of both environmentally-responsive and externally-triggered biomaterials for biomolecule delivery, we recommend the recent publication of Oliva and Almquist.143 For the purpose of this review, which focuses specifically on biomaterials that address and respond to complex injury environments, we will describe how endogenous triggers in the in vivo environment can induce material changes that modulate drug and protein release.
Thermally-responsive materials provide a means of controlling protein loading and release based on body temperature. Polymers such as poly(N-isopropyl acrylamide) (PNIPAAm) and block copolymers consisting of variations of PEG, polylactic acid (PLA), PCL, and poly(L-lactide) (PLLA) change their conformation from hydrophilic to hydrophobic at a lowest critical solution temperature (LCST), allowing for protein release or sequestration at specific temperatures.144,145 PNIPAAm is particularly useful in thermally-mediated delivery applications because its hydrophobic/hydrophilic conformation changes are reversible at biologically relevant temperatures. Kim et al. developed nanoparticles consisting of a thermo-responsive PNIPAAm domain, hydrophobic PLLA domain, and hydrophilic poly(L-lysine) (PLL) domain to deliver nerve growth factor (NGF).146 PNIPAAm enabled loading of NGF under aqueous conditions and sustained NGF release at 37 °C, above the LCST. The uptake of the nanoparticles into neuroblast-like PC12 cells was also temperature-dependent, with higher uptake above the LCST, which stimulated increased neurite outgrowth. Although these studies were conducted in vitro, the ability of PNIPAAm nanoparticles to exhibit optimal sustained protein release and cellular uptake at physiological temperatures makes them valuable for in vivo therapeutic applications. Other phase changing molecules can also be used to align or open porous channels to allow biomolecule release. Tetradecanol, a fatty alcohol that is solid at 37 °C and melts at 39 °C, has been used to provide on-demand, heat-triggered release of doxorubicin in cancer cell cultures.147 While promising for in vitro use, tetradecanol has not yet been used extensively in vivo.
Targeting chemically unique attributes of injury sites such as pH can enhance site-specific protein delivery while reducing off-target release. Biomaterials can be engineered to respond specifically to the microenvironment of the site of injury or disease,148 as is often done with the acidic environment of cancer cells.114,149 pH sensitive vehicles are typically made through the integration of polyelectrolytes, such as phenylboronic acid (PBA),150–152 due to their ability to change their overall charge at different pH, resulting in triggered release.114,153,154 Cong et al. crosslinked chitosan with glycerol 2-phospate disodium to created pH sensitive micelles, which were then dispersed in an alginate hydrogel. The group analyzed the release rate of drug in various pH conditions with different formulations and found that the micelles alone would degrade too quickly while increasing the concentration of alginate surrounding the micelles slowed the release in a controlled fashion.
Additional strategies to create environmentally-responsive materials have also been employed. Biomaterials have been designed to degrade and release molecular cargo in the presence of reactive oxygen species, which are often increased in the initial inflammatory phase of tissue injury.155 Materials crosslinked using peptide sequences that can be cleaved by enzymes, such as matrix metalloproteinases (MMPs), offer a strategy for triggered protein release that responds to fluctuating MMP expression in the injury environment.156 These environmentally-responsive materials provide several methods to target a therapeutic to specific injury environments and thus have considerable overlap with tissue targeting strategies.
Collectively, the strategies described here leverage a variety of material properties to enhance material integration into regenerating tissues while providing sustained protein or drug release. Incorporating tissue targeting, tissue mimicking, and environmentally-responsive elements into biomaterial delivery vehicles is a promising approach to enhance the efficacy of biomaterial-based protein delivery to injured tissues.
Multi-component strategies
We have identified a number of strategies to design biomaterials that stimulate tissue repair through delivery of proteins involved in healing cascades. Combining multiple approaches to create complex biomaterials that perform multiple functions can further enhance the ability of biomaterials to respond to environmental changes during tissue injury and repair.
Biomaterials designed to repair tissues with electrical activity (e.g. cardiac, nerve, muscle) can incorporate responsiveness to electrical stimuli to be both tissue mimicking and stimuli-responsive. Conductive biomaterials, such as graphene, carbon nanotubes, gold, polypyrole (PPy), polyaniline (PANI), and poly(3,4-ethylenedioxythiophene) (PEDOT),132 can respond to electrical stimuli through contraction or expansion and subsequent relaxation, making them prime candidates for muscle mimicry. Graphene, gold, and carbon nanotubes are excellent conductors, but their long-term effects in the body are unknown. Conversely, PPy, PANI, and PEDOT are organic materials, but are not as effective for electrical signal conduction. Conductive materials have been used extensively for scaffolding for regenerating skeletal muscle132,157 and cardiac muscle.158 The Dvir group developed a complex cardiac patch consisting of gold electrodes that could be independently triggered by electrical stimuli to release proteins.159 50 μm electrodes were placed in an array, covered in negatively-charged chondroitin sulphate, and electrostatically loaded with positively-charged proteins – lysozyme, a model protein, and SDF-1α, a chemokine that stimulates cell recruitment to sites of myocardial injury. Each node monitored the immediate surrounding electrical activity of the cardiac muscle and, upon sufficient signalling, would release its payload. This strategy enabled both detection and stimulation of electrical signals in cardiomyocytes, as well as on-demand protein release. Future applications of this technology could include additional sensing capabilities and feedback loops that enable remote control over patient cardiac tissue regeneration. Overall, electrically active materials may provide an enhanced ability to restore signalling pathways and re-establish function of electrically active tissues.
Advancements in additive manufacturing have opened additional possibilities for integrated solutions by enabling the fabrication of biomaterials with complex architectures and spatially-defined properties for controlled protein and peptide presentation.73,140,160,161 This technique is valuable for mimicking tissues that naturally contain gradients, such as cartilage162 and osteochondral interfaces, or displaying complex architecture, such as vasculature.163,164 For example, peptide-polymer conjugates can be 3D-printed to control the spatial presentation of cell-adhesive (RGDS) and non-adhesive (RGES) peptides.162 This strategy achieved precise control over local cell behavior using a single biomaterial scaffold and has future applications in creating complex tissue architectures. Additive manufacturing has also been used to mimic the structure of cortical and cancellous bone. A bioceramic scaffold was 3D-printed to mimic the hierarchical structure of bone, including the Haversian canals present in cortical bone structure.165 This scaffold fostered proliferation and protein/ECM secretion by co-delivered mesenchymal stem cells, Schwann cells, and endothelial cells and fostered robust integration, bone formation, and revascularization in a rabbit femoral bone defect model. Additive manufacturing can also be combined with protein immobilization to create 3D printing structures that enable spatially localized protein presentation.166 Subbiah et al. demonstrated that lithography-based 3D-printing could be used to generate ceramic microcages that could be combined in a modular fashion (Fig. 7).167,168 Hydrogels containing a variety of growth factors (VEGF, PDGF, and BMP-2) were loaded into the microcages and combined to modulate cell behaviour both in vitro and in vivo. Together, these studies demonstrate that additive manufacturing can be used to create multifunctional materials with both defined architectures and protein presentation.
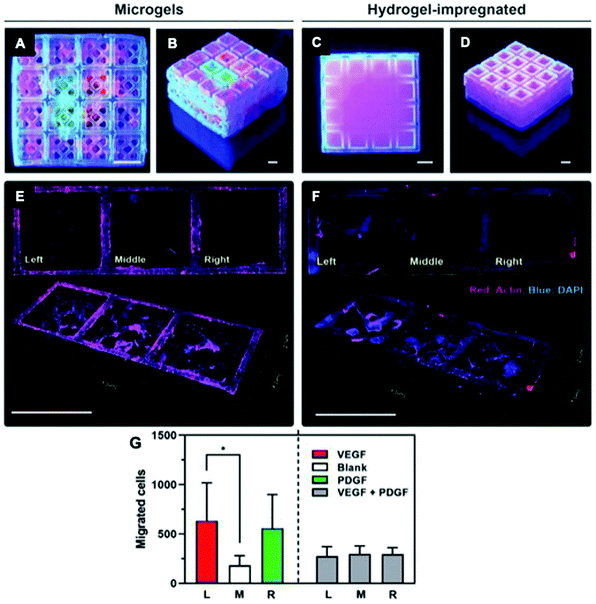 |
| Fig. 7 Use of 3D-printed, microgel-loaded microcages for protein delivery. (A and B) 3D-printed microcage scaffolds loaded with fluorescently labelled microparticles embedded within microgels, and (C and D) 3D printed microcage scaffolds loaded with fluorescently labelled microparticles within a bulk gelatin methacrylate hydrogel. (E) Directed cell migration achieved by the VEGF-laden microgels (left), no growth factor (middle), and PDGF-laden microgel (right). (F) Non-directed cell migration caused by the bulk hydrogel-impregnated scaffolds. Scale bars: 1.5 mm. Top: 2D view, bottom: 3D view. (G) Quantification of cell migration in each microcage (* p < 0.05). Reproduced from ref. 168 with permission from Wiley-VCH, copyright 2020. | |
Current market-available strategies
The use of therapeutic proteins in the clinic has exploded in the past several decades. By 2017, the FDA had approved a total of 239 proteins and peptides for therapeutic applications.169 The translation of innovative therapeutic protein strategies to market is challenging due to the complexity of regulatory approval processes. As a result, what is currently available on the market lags behind the recent advances introduced in this review. However, several protein therapeutics used in the clinic today use a biomaterial-based approach for in vivo protein localization. For example, Medtronic produces INFUSE®, which is a bone graft consisting of recombinant human BMP-2 soaked into a collagen sponge, and is used for bone surgery applications such as tibial fractures and spinal fusion. This industry standard uses two of the simpler approaches explored in this review – physical entrapment and ECM-based biomaterials.170 Boyne carried out clinical trials for what would become INFUSE® in 1996,171 with additional trials conducted by Riedel and Valentin-Opran in 1999.172 After nearly 10 years of clinical studies, the product was approved in the early 2000s by the United States Food and Drug Administration (FDA).173
Other tissue healing strategies that employ allografts and ECM-derived biomaterials are also currently used in the clinic. Although most of these ECM materials provide limited spatiotemporal control over protein retention and release, they retain many functional biological components, such as cells, proteins, and ECM molecules.174 Osiris Therapeutics, Inc. produces protein- and cell-rich scaffolds derived from placental membranes for a variety of applications, including dermal tissue,175 bone, and cartilage repair.176,177 Preserved placental membranes are also available from other companies for ocular applications.178,179 However, viable allograft tissue with suitable properties for tissue healing can be scarce. One strategy to overcome this challenge is to conjugate other biomaterials to allografts to both reduce the amount of foreign tissue required and adjust the mechanical properties of the allograft for specific applications.180
Although allografts and collagen sponges can be cut to fit the size of the tissue defect, they cannot be easily customized to a predefined structure. The 3D printing strategies described in this review are currently being used by numerous medical device companies to address the specific structural needs of a variety of tissues and organs. Biological structures can be 3D-printed with a variety of scaffold materials that enable direct integration of proteins into the bioprinting ink.181,182 Although the FDA has yet not cleared the use of 3D bioprinting solutions for whole organ and tissue transplantation, that is a key objective of many of these companies.
Future outlooks
The strategies described in this review are all at different stages of development, with some being primarily investigated in vitro, some being currently assessed in vivo, and others moving towards clinical translation. Achieving robust tissue regeneration is a complex challenge with no ubiquitous solution; thus, different biomaterial strategies may be required for tissue injuries that alter healing cascades in different ways. The use of high throughput technologies and computational models are recent developments in the field of biomaterials that have the potential to expand our ability to better address the complexity of the wound healing cascade.
Since numerous biomaterial properties (e.g. protein–material affinity, mechanical properties, degradation) interact to influence tissue repair, the ability to generate large numbers of new biomaterial formulations could drastically accelerate progress in the development of biomaterials for tissue regeneration. Recent advances in high throughput screening technologies are expected to enable automated combinatoric synthesis and material discovery. We have recently developed a technique to evaluate protein diffusion through hydrogels using fluorescent proteins loaded into hydrogel-filled capillary tubes.183 This technique enabled the determination of protein diffusion coefficients using small volumes of proteins and hydrogels and has been adapted for use with both physically and chemically crosslinked hydrogels (PEG, collagen, and alginate). On a larger scale, Xu et al. used a high throughput liquid handling robot to combine various charged (cationic or anionic poly(oligo ethylene glycol methacrylate) (POEGMA)), uncharged (dextran, carboxymethyl cellulose, and chitosan), thermoresponsive (PNIPAM, POEGMA), non-thermoresponsive, synthetic, and natural precursor polymers.184 The biomaterials fabricated were then loaded with ovalbumin, as a model protein to evaluate how biomaterial properties affected protein release. Almost 100 unique polymer combinations were made and analyzed, which was a substantial increase in the typical number of biomaterial formulations that can be tested simultaneously. Similarly, Hao et al. used One Bead One Compound (OBOC) high throughput screening technology to identify high affinity binding ligands for endothelial progenitor cells (EPCs) and endothelial cells (ECs) with minimal affinity for many other biological components.185 OBOC uses magnetically conjugated target proteins for binding and sorting through large numbers of peptide sequences, akin to phage sorting.186 The binding ligands conjugated to small vascular grafts achieved rapid endothelialization in vivo and retained high patency in vivo in a rat carotid bypass model.
High-throughput biomaterial screening can be combined with computational modelling approaches to further expand our ability to screen a vast number of biomaterial properties for tissue regeneration applications. Several studies have demonstrated the utility of statistical optimization techniques, such as design of experiments, to optimize biomaterial properties achieve specific outcomes, such as cell response and gelation kinetics.187–189 Additionally, we and others have employed computational bio-transport models to predict protein release kinetics from biomaterials and tune biomaterial properties to achieve desired release rates in vitro and in vivo.10,64,77 Limasale et al. demonstrated that a reaction-diffusion model could be used to predict the effect of heparin content and sulphation pattern on VEGF release, as well as the spatial distribution of VEGF throughout the hydrogel. Collectively, these examples demonstrate the value of high throughput screening technologies and computational modelling for the rational design of biomaterials for tissue engineering applications. Future work that translates these in vitro results and in silico models into viable predictions for biomaterial behaviour within in vivo injury environments has significant potential for improving biomaterials for better therapeutic outcomes.
Conclusions
The complex nature of tissue healing, in which numerous cells, proteins, and matrix molecules participate, is difficult to replicate or restore when normal repair mechanisms are disrupted. Here, we have compiled and reviewed a number of recent advancements in biomaterials for sustained protein delivery, with a focus on strategies that improve the ability of biomaterials to address the complexity of the injury environment during healing. Numerous strategies exist to tailor protein presentation to unique tissue regeneration applications, including covalent and physical entrapment of proteins, the use of ECM-based materials, engineered affinity interactions, environmentally-responsive materials for on-demand protein delivery, and complex fabrication methods to better mimic native tissue architecture. Ultimately, the choice of the most appropriate biomaterial strategy to enable regeneration of a particular tissue rests on gaining a better understanding of how spatiotemporal protein presentation affects healing in that tissue. With the broad spectrum of engineering tools available to create new biomaterials, we expect that we will be able to both expand our understanding of how proteins influence tissue healing responses and create advanced biomaterials that respond to and integrate into complex injury environments. While many challenges remain in identifying and creating the appropriate material properties to stimulate repair of non-healing tissue defects, emerging interest in designing biomaterials that better integrate and respond to injured tissues is expected to open numerous doors to improved strategies for patient treatment.
Conflicts of interest
There are no conflicts to declare.
Acknowledgements
Schematic figures were created with BioRender.com. We thank Dr. Kaitlin Fogg and Matthew Steele for their thoughtful review of this manuscript.
References
- A. K. Gaharwar, I. Singh and A. Khademhosseini, Engineered Biomaterials for in Situ Tissue Regeneration, Nat. Rev. Mater., 2020, 5(9), 686–705, DOI:10.1038/s41578-020-0209-x.
- S. Guo and L. A. DiPietro, Factors Affecting Wound Healing, J. Dent. Res., 2010, 89(3), 219–229, DOI:10.1177/0022034509359125.
- M. T. Ho, C. J. Teal and M. S. Shoichet, A Hyaluronan/Methylcellulose-Based Hydrogel for Local Cell and Biomolecule Delivery to the Central Nervous System, Brain Res. Bull., 2019, 148, 46–54, DOI:10.1016/j.brainresbull.2019.03.005.
- H. Sinno and S. Prakash, Complements and the Wound Healing Cascade: An Updated Review, Plast Surg Int, 2013, 2013 DOI:10.1155/2013/146764.
- E. Rand and A. C. Gellhorn, The Healing Cascade: Facilitating and Optimizing the System, Phys. Med. Rehabil. Clin. N. Am., 2016, 27(4), 765–781, DOI:10.1016/j.pmr.2016.07.001.
- M. Mehta, K. Schmidt-Bleek, G. N. Duda and D. J. Mooney, Biomaterial Delivery of Morphogens to Mimic the Natural Healing Cascade in Bone, Adv. Drug Delivery Rev., 2012, 64(12), 1257–1276, DOI:10.1016/j.addr.2012.05.006.
- S. Ellis, E. J. Lin and D. Tartar, Immunology of Wound Healing, Curr. Dermatol. Rep., 2018, 7(4), 350–358, DOI:10.1007/s13671-018-0234-9.
- V. Bianconi, A. Sahebkar, S. L. Atkin and M. Pirro, The Regulation and Importance of Monocyte Chemoattractant Protein-1, Curr. Opin. Hematol., 2018, 25(1), 44–51, DOI:10.1097/MOH.0000000000000389.
- N. Ganapathy, S. S. Venkataraman, R. Daniel, R. J. Aravind and V. B. Kumarakrishnan, Molecular Biology of Wound Healing, J. Pharm. BioAllied Sci., 2012, 4(Suppl 2), S334–S337, DOI:10.4103/0975-7406.100294.
- M. H. Hettiaratchi, L. Krishnan, T. Rouse, C. Chou, T. C. McDevitt and R. E. Guldberg, Heparin-Mediated Delivery of Bone Morphogenetic Protein-2 Improves Spatial Localization of Bone Regeneration, Sci. Adv., 2020, 6(1), eaay1240, DOI:10.1126/sciadv.aay1240.
- J. M. Townsend, G. Sali, H. B. Homburg, N. T. Cassidy, M. E. Sanders, K.-M. Fung, B. T. Andrews, R. J. Nudo, B. N. Bohnstedt and M. S. Detamore, Thiolated Bone and Tendon Tissue Particles Covalently Bound in Hydrogels for in Vivo Calvarial Bone Regeneration, Acta Biomater., 2020, 104, 66–75, DOI:10.1016/j.actbio.2019.12.035.
- J. M. Townsend, B. T. Andrews, Y. Feng, J. Wang, R. J. Nudo, E. Van Kampen, S. H. Gehrke, C. J. Berkland and M. S. Detamore, Superior Calvarial Bone Regeneration Using Pentenoate-Functionalized Hyaluronic Acid Hydrogels with Devitalized Tendon Particles, Acta Biomater., 2018, 71, 148–155, DOI:10.1016/j.actbio.2018.02.013.
- R. Chen, J. Wang and C. Liu, Biomaterials Act as Enhancers of Growth Factors in Bone Regeneration, Adv. Funct. Mater., 2016, 26(48), 8810–8823, DOI:10.1002/adfm.201603197.
- A. Cheng, L. Krishnan, P. Pradhan, L. D. Weinstock, L. B. Wood, K. Roy and R. E. Guldberg, Impaired Bone Healing Following Treatment of Established Nonunion Correlates with Serum Cytokine Expression, J. Orthop. Res., 2019, 37(2), 299–307, DOI:10.1002/jor.24186.
- H. Cho, M. R. Blatchley, E. J. Duh and S. Gerecht, Acellular and Cellular Approaches to Improve Diabetic Wound Healing, Adv. Drug Delivery Rev., 2019, 146, 267–288, DOI:10.1016/j.addr.2018.07.019.
- Z. Zhai, K. Xu, L. Mei, C. Wu, J. Liu, Z. Liu, L. Wan and W. Zhong, Co-Assembled Supramolecular Hydrogels of Cell Adhesive Peptide and Alginate for Rapid Hemostasis and Efficacious Wound Healing, Soft Matter, 2019, 15(42), 8603–8610, 10.1039/C9SM01296F.
- Z. Bagher, Z. Atoufi, R. Alizadeh, M. Farhadi, P. Zarrintaj, L. Moroni, M. Setayeshmehr, A. Komeili and S. K. Kamrava, Conductive Hydrogel Based on Chitosan-Aniline Pentamer/Gelatin/Agarose Significantly Promoted Motor Neuron-like Cells Differentiation of Human Olfactory Ecto-Mesenchymal Stem Cells, Mater. Sci. Eng., C, 2019, 101, 243–253, DOI:10.1016/j.msec.2019.03.068.
- S. Shafei, M. Khanmohammadi, R. Heidari, H. Ghanbari, V. T. Nooshabadi, S. Farzamfar, M. Akbariqomi, N. S. Sanikhani, M. Absalan and G. Tavoosidana, Exosome Loaded Alginate Hydrogel Promotes Tissue Regeneration in Full-Thickness Skin Wounds: An in Vivo Study, J. Biomed. Mater. Res., Part A, 2020, 108(3), 545–556, DOI:10.1002/jbm.a.36835.
- H. Wen, J. Li, G. F. Payne, Q. Feng, M. Liang, J. Chen, H. Dong and X. Cao, Hierarchical Patterning via Dynamic Sacrificial Printing of Stimuli-Responsive Hydrogels, Biofabrication, 2020, 12(3), 035007, DOI:10.1088/1758-5090/ab7e74.
- E. A. Kiyotake, A. W. Douglas, E. E. Thomas, S. L. Nimmo and M. S. Detamore, Development and Quantitative Characterization of the Precursor Rheology of Hyaluronic Acid Hydrogels for Bioprinting, Acta Biomater., 2019, 95, 176–187, DOI:10.1016/j.actbio.2019.01.041.
- Q. Vallmajo-Martin, N. Broguiere, C. Millan, M. Zenobi-Wong and M. Ehrbar, PEG/HA Hybrid Hydrogels for Biologically and Mechanically Tailorable Bone Marrow Organoids, Adv. Funct. Mater., 2020, 30(48), 1910282, DOI:10.1002/adfm.201910282.
- B. S. Kim, S. Das, J. Jang and D.-W. Cho, Decellularized Extracellular Matrix-Based Bioinks for Engineering Tissue- and Organ-Specific Microenvironments, Chem. Rev., 2020, 120(19), 10608–10661, DOI:10.1021/acs.chemrev.9b00808.
- S. H. Aswathy, U. Narendrakumar and I. Manjubala, Commercial Hydrogels for Biomedical Applications, Heliyon, 2020, 6(4), e03719, DOI:10.1016/j.heliyon.2020.e03719.
- M. C. Catoira, L. Fusaro, D. Di Francesco, M. Ramella and F. Boccafoschi, Overview of Natural Hydrogels for Regenerative Medicine Applications, J. Mater. Sci.: Mater. Med., 2019, 30(10), 115, DOI:10.1007/s10856-019-6318-7.
- S. Žigon-Branc, M. Markovic, J. Van Hoorick, S. Van Vlierberghe, P. Dubruel, E. Zerobin, S. Baudis and A. Ovsianikov, Impact of Hydrogel Stiffness on Differentiation of Human Adipose-Derived Stem Cell Microspheroids, Tissue Eng., Part A, 2019, 25(19–20), 1369–1380, DOI:10.1089/ten.tea.2018.0237.
- L. M. Caballero Aguilar, S. M. Silva and S. E. Moulton, Growth Factor Delivery: Defining the next Generation Platforms for Tissue Engineering, J. Controlled Release, 2019, 306, 40–58, DOI:10.1016/j.jconrel.2019.05.028.
- J. Li and D. J. Mooney, Designing Hydrogels for Controlled Drug Delivery, Nat. Rev. Mater., 2016, 1(12), 16071, DOI:10.1038/natrevmats.2016.71.
- J. M. Townsend, S. C. Dennis, J. Whitlow, Y. Feng, J. Wang, B. Andrews, R. J. Nudo, M. S. Detamore and C. J. Berkland, Colloidal Gels with Extracellular Matrix Particles and Growth Factors for Bone Regeneration in Critical Size Rat Calvarial Defects, AAPS J., 2017, 19(3), 703–711, DOI:10.1208/s12248-017-0045-0.
- J. C. Grim, T. E. Brown, B. A. Aguado, D. A. Chapnick, A. L. Viert, X. Liu and K. S. Anseth, A Reversible and Repeatable Thiol–Ene Bioconjugation for Dynamic Patterning of Signaling Proteins in Hydrogels, ACS Cent. Sci., 2018, 4(7), 909–916, DOI:10.1021/acscentsci.8b00325.
- F. Ma, X. Pang and B. Tang, Alginate/Chondroitin Sulfate Based Hybrid Hydrogel
with Different Molecular Weight and Its Capacity to Regulate Chondrocytes Activity, Carbohydr. Polym., 2019, 206, 229–237, DOI:10.1016/j.carbpol.2018.10.109.
- M. E. Melica, G. La Regina, M. Parri, A. J. Peired, P. Romagnani and L. Lasagni, Substrate Stiffness Modulates Renal Progenitor Cell Properties via a ROCK-Mediated Mechanotransduction Mechanism, Cells, 2019, 8(12), 1561, DOI:10.3390/cells8121561.
- M. Mochizuki, E. Güç, A. J. Park, Z. Julier, P. S. Briquez, G. A. Kuhn, R. Müller, M. A. Swartz, J. A. Hubbell and M. M. Martino, Growth Factors with Enhanced Syndecan Binding Generate Tonic Signalling and Promote Tissue Healing, Nat. Biomed. Eng., 2020, 4(4), 463–475, DOI:10.1038/s41551-019-0469-1.
- D. J. Page, C. E. Clarkin, R. Mani, N. A. Khan, J. I. Dawson and N. D. Evans, Injectable Nanoclay Gels for Angiogenesis, Acta Biomater., 2019, 100, 378–387, DOI:10.1016/j.actbio.2019.09.023.
- X. Tong, S. Lee, L. Bararpour and F. Yang, Long-Term Controlled Protein Release from Poly(Ethylene Glycol) Hydrogels by Modulating Mesh Size and Degradation: Long-Term Controlled Protein Release from Poly(Ethylene Glycol) Hydrogels…, Macromol. Biosci., 2015, 15(12), 1679–1686, DOI:10.1002/mabi.201500245.
- D. Perera, M. Medini, D. Seethamraju, R. Falkowski, K. White and R. M. Olabisi, The Effect of Polymer Molecular Weight and Cell Seeding Density on Viability of Cells Entrapped within PEGDA Hydrogel Microspheres, J. Microencapsulation, 2018, 35(5), 475–481, DOI:10.1080/02652048.2018.1526341.
- M. S. Rehmann, K. M. Skeens, P. M. Kharkar, E. M. Ford, E. Maverakis, K. H. Lee and A. M. Kloxin, Tuning and Predicting Mesh Size and Protein Release from Step Growth Hydrogels, Biomacromolecules, 2017, 18(10), 3131–3142, DOI:10.1021/acs.biomac.7b00781.
- J. Kim, R. Lima e Silva, R. B. Shmueli, A. C. Mirando, S. Y. Tzeng, N. B. Pandey, E. Ben-Akiva, A. S. Popel, P. A. Campochiaro and J. J. Green, Anisotropic Poly(Lactic-Co-Glycolic Acid) Microparticles Enable Sustained Release of a Peptide for Long-Term Inhibition of Ocular Neovascularization, Acta Biomater., 2019, 97, 451–460, DOI:10.1016/j.actbio.2019.07.054.
- L. N. Woodard and M. A. Grunlan, Hydrolytic Degradation and Erosion of Polyester Biomaterials, ACS Macro Lett., 2018, 7(8), 976–982, DOI:10.1021/acsmacrolett.8b00424.
- E. Vey, C. Rodger, J. Booth, M. Claybourn, A. F. Miller and A. Saiani, Degradation Kinetics of Poly(Lactic-Co-Glycolic) Acid Block Copolymer Cast Films in Phosphate Buffer Solution as Revealed by Infrared and Raman Spectroscopies, Polym. Degrad. Stab., 2011, 96(10), 1882–1889, DOI:10.1016/j.polymdegradstab.2011.07.011.
- L. C. Amann, M. J. Gandal, R. Lin, Y. Liang and S. J. Siegel, In Vitro–In Vivo Correlations of Scalable PLGA-Risperidone Implants for the Treatment of Schizophrenia, Pharm. Res., 2010, 27(8), 1730–1737, DOI:10.1007/s11095-010-0152-4.
- D. H. Choi, C. H. Park, I. H. Kim, H. J. Chun, K. Park and D. K. Han, Fabrication of Core–Shell Microcapsules Using PLGA and Alginate for Dual Growth Factor Delivery System, J. Controlled Release, 2010, 147(2), 193–201, DOI:10.1016/j.jconrel.2010.07.103.
- J. R. Yu, M. Janssen, B. J. Liang, H.-C. Huang and J. P. Fisher, A Liposome/Gelatin Methacrylate Nanocomposite Hydrogel System for Delivery of Stromal Cell-Derived Factor-1α and Stimulation of Cell Migration, Acta Biomater., 2020, 108, 67–76, DOI:10.1016/j.actbio.2020.03.015.
- L. M. Mancipe Castro, A. Sequeira, A. J. García and R. E. Guldberg, Articular Cartilage- and Synoviocyte-Binding Poly(Ethylene Glycol) Nanocomposite Microgels as Intra-Articular Drug Delivery Vehicles for the Treatment of Osteoarthritis, ACS Biomater. Sci. Eng., 2020, 6(9), 5084–5095, DOI:10.1021/acsbiomaterials.0c00960.
- M. M. Pakulska, I. Elliott Donaghue, J. M. Obermeyer, A. Tuladhar, C. K. McLaughlin, T. N. Shendruk and M. S. Shoichet, Encapsulation-Free Controlled Release: Electrostatic Adsorption Eliminates the Need for Protein Encapsulation in PLGA Nanoparticles, Sci. Adv., 2016, 2(5), e1600519, DOI:10.1126/sciadv.1600519.
- S. Rajabi, S. Jalili-Firoozinezhad, M. K. Ashtiani, G. Le Carrou, S. Tajbakhsh and H. Baharvand, Effect of Chemical Immobilization of SDF-1α into Muscle-Derived Scaffolds on Angiogenesis and Muscle Progenitor Recruitment, J. Tissue Eng. Regener. Med., 2018, 12(1), e438–e450, DOI:10.1002/term.2479.
- A. Singh, M. Corvelli, S. A. Unterman, K. A. Wepasnick, P. McDonnell and J. H. Elisseeff, Enhanced Lubrication on Tissue and Biomaterial Surfaces through Peptide-Mediated Binding of Hyaluronic Acid, Nat. Mater., 2014, 13(10), 988–995, DOI:10.1038/nmat4048.
- C. E. Tanase, O. Qutachi, L. J. White, K. M. Shakesheff, A. W. McCaskie, S. M. Best and R. E. Cameron, Targeted Protein Delivery: Carbodiimide Crosslinking Influences Protein Release from Microparticles Incorporated within Collagen Scaffolds, Regener. Biomater., 2019, 6(5), 279–287, DOI:10.1093/rb/rbz015.
- Y. Wang, H. Lan, T. Yin, X. Zhang, J. Huang, H. Fu, J. Huang, S. McGinty, H. Gao, G. Wang and Z. Wang, Covalent Immobilization of Biomolecules on Stent Materials through Mussel Adhesive Protein Coating to Form Biofunctional Films, Mater. Sci. Eng., C, 2020, 106, 110187, DOI:10.1016/j.msec.2019.110187.
- L. Petrone, A. Kumar, C. N. Sutanto, N. J. Patil, S. Kannan, A. Palaniappan, S. Amini, B. Zappone, C. Verma and A. Miserez, Mussel Adhesion Is Dictated by Time-Regulated Secretion and Molecular Conformation of Mussel Adhesive Proteins, Nat. Commun., 2015, 6(1), 8737, DOI:10.1038/ncomms9737.
- D. V. Bax, N. Davidenko, S. W. Hamaia, R. W. Farndale, S. M. Best and R. E. Cameron, Impact of UV- and Carbodiimide-Based Crosslinking on the Integrin-Binding Properties of Collagen-Based Materials, Acta Biomater., 2019, 100, 280–291, DOI:10.1016/j.actbio.2019.09.046.
- L. Magnan, G. Labrunie, M. Fénelon, N. Dusserre, M. P. Foulc, M. Lafourcade, I. Svahn, E. Gontier, J. H. Vélez V., T. N. McAllister and N. L’Heureux, Human Textiles: A Cell-Synthesized Yarn as a Truly “Bio” Material for Tissue Engineering Applications, Acta Biomater., 2020, 105, 111–120, DOI:10.1016/j.actbio.2020.01.037.
- J.-Y. Lai, L.-J. Luo and D. Ma, Effect of Cross-Linking Density on the Structures and Properties of Carbodiimide-Treated Gelatin Matrices as Limbal Stem Cell Niches, Int. J. Mol. Sci., 2018, 19(11), 3294, DOI:10.3390/ijms19113294.
- M. C. Murphy and N. K. Howell, Effects of the Attachment of Small Molecules by Carbodiimide-Mediated Condensation on the Physicochemical and Functional Properties of Bovine Serum Albumin: Carbodiimide-Mediated Condensation and BSA Properties, J. Sci. Food Agric., 1991, 55(1), 117–140, DOI:10.1002/jsfa.2740550112.
- S. Yamamoto, Y. Iwamaru, Y. Shimizu, Y. Ueda, M. Sato, K. Yamaguchi and J. Nakanishi, Epidermal Growth Factor-Nanoparticle Conjugates Change the Activity from Anti-Apoptotic to pro-Apoptotic at Membrane Rafts, Acta Biomater., 2019, 88, 383–391, DOI:10.1016/j.actbio.2019.02.026.
- C. Q. Vu, P. Rotkrua, B. Soontornworajit and Y. Tantirungrotechai, Effect of PDGF-B Aptamer on PDGFRβ/PDGF-B Interaction: Molecular Dynamics Study, J. Mol. Graphics Modell., 2018, 82, 145–156, DOI:10.1016/j.jmgm.2018.04.012.
- J. A. Shadish, G. M. Benuska and C. A. DeForest, Bioactive Site-Specifically Modified Proteins for 4D Patterning of Gel Biomaterials, Nat. Mater., 2019, 18(9), 1005–1014, DOI:10.1038/s41563-019-0367-7.
- M. H. Hettiaratchi, M. J. O'Meara, C. J. Teal, S. L. Payne, A. J. Pickering and M. S. Shoichet, Local Delivery of Stabilized Chondroitinase ABC Degrades Chondroitin Sulfate Proteoglycans in Stroke-Injured Rat Brains, J. Controlled Release, 2019, 297, 14–25, DOI:10.1016/j.jconrel.2019.01.033.
- J. A. Shadish and C. A. DeForest, Site-Selective Protein Modification: From Functionalized Proteins to Functional Biomaterials, Matter, 2020, 2(1), 50–77, DOI:10.1016/j.matt.2019.11.011.
- S. B. Selleck, Proteoglycans and Pattern Formation: Sugar Biochemistry Meets Developmental Genetics, Trends Genet., 2000, 16(5), 206–212, DOI:10.1016/S0168-9525(00)01997-1.
- T. Shimogori, Embryonic Signaling Centers Expressing BMP, WNT and FGF Proteins Interact to Pattern the Cerebral Cortex, Development, 2004, 131(22), 5639–5647, DOI:10.1242/dev.01428.
- S. Kishigami and Y. Mishina, BMP Signaling and Early Embryonic Patterning, Cytokine Growth Factor Rev., 2005, 16(3), 265–278, DOI:10.1016/j.cytogfr.2005.04.002.
- T. Miller, M. C. Goude, T. C. McDevitt and J. S. Temenoff, Molecular Engineering of Glycosaminoglycan Chemistry for Biomolecule Delivery, Acta Biomater., 2014, 10(4), 1705–1719, DOI:10.1016/j.actbio.2013.09.039.
- G. Tan, J. Li, Y. Song, Y. Yu, D. Liu and W. Pan, Phenylboronic Acid-Tethered Chondroitin Sulfate-Based Mucoadhesive Nanostructured Lipid Carriers for the Treatment of Dry Eye Syndrome, Acta Biomater., 2019, 99, 350–362, DOI:10.1016/j.actbio.2019.08.035.
- M. H. Hettiaratchi, T. Rouse, C. Chou, L. Krishnan, H. Y. Stevens, M.-T. A. Li, T. C. McDevitt and R. E. Guldberg, Enhanced in Vivo Retention of Low Dose BMP-2 via Heparin Microparticle Delivery Does Not Accelerate Bone Healing in a Critically Sized Femoral Defect, Acta Biomater., 2017, 59, 21–32, DOI:10.1016/j.actbio.2017.06.028.
- M. C. Z. Meneghetti, A. J. Hughes, T. R. Rudd, H. B. Nader, A. K. Powell, E. A. Yates and M. A. Lima, Heparan Sulfate and Heparin Interactions with Proteins, J. R. Soc., Interface, 2015, 12(110), 20150589, DOI:10.1098/rsif.2015.0589.
- S. Andrews, A. Cheng, H. Stevens, M. T. Logun, R. Webb, E. Jordan, B. Xia, L. Karumbaiah, R. E. Guldberg and S. Stice, Chondroitin Sulfate Glycosaminoglycan Scaffolds for Cell and Recombinant Protein–Based Bone Regeneration, Stem Cells Transl. Med., 2019, 8(6), 575–585, DOI:10.1002/sctm.18-0141.
- M. L. Talaga, N. Fan, A. L. Fueri, R. K. Brown, P. Bandyopadhyay and T. K. Dam, Multitasking Human Lectin Galectin-3 Interacts with Sulfated Glycosaminoglycans and Chondroitin Sulfate Proteoglycans, Biochemistry, 2016, 55(32), 4541–4551, DOI:10.1021/acs.biochem.6b00504.
- A. Abednejad, A. Ghaee, E. S. Morais, M. Sharma, B. M. Neves, M. G. Freire, J. Nourmohammadi and A. A. Mehrizi, Polyvinylidene Fluoride–Hyaluronic Acid Wound Dressing Comprised of Ionic Liquids for Controlled Drug Delivery and Dual Therapeutic Behavior, Acta Biomater., 2019, 100, 142–157, DOI:10.1016/j.actbio.2019.10.007.
- D. Bermejo-Velasco, S. Kadekar, M. V. Tavares da Costa, O. P. Oommen, K. Gamstedt, J. Hilborn and O. P. Varghese, First Aldol Cross-Linked Hyaluronic Acid Hydrogel: Fast and Hydrolytically Stable Hydrogel with Tissue Adhesive Properties, ACS Appl. Mater. Interfaces, 2019, 11(41), 38232–38239, DOI:10.1021/acsami.9b10239.
- Q. Feng, S. Lin, K. Zhang, C. Dong, T. Wu, H. Huang, X. Yan, L. Zhang, G. Li and L. Bian, Sulfated Hyaluronic Acid Hydrogels with Retarded Degradation and Enhanced Growth Factor Retention Promote HMSC Chondrogenesis and Articular Cartilage Integrity with Reduced Hypertrophy, Acta Biomater., 2017, 53, 329–342, DOI:10.1016/j.actbio.2017.02.015.
- A. Bhattacharyya, D. Mukherjee, R. Mishra and P. P. Kundu, Development of PH Sensitive Polyurethane–Alginate Nanoparticles for Safe and Efficient Oral Insulin Delivery in Animal Models, RSC Adv., 2016, 6(48), 41835–41846, 10.1039/C6RA06749B.
- Z. He, J. L. Santos, H. Tian, H. Huang, Y. Hu, L. Liu, K. W. Leong, Y. Chen and H.-Q. Mao, Scalable Fabrication of Size-Controlled Chitosan Nanoparticles for Oral Delivery of Insulin, Biomaterials, 2017, 130, 28–41, DOI:10.1016/j.biomaterials.2017.03.028.
- L. Dong, S.-J. Wang, X.-R. Zhao, Y.-F. Zhu and J.-K. Yu, 3D- Printed Poly(ε-Caprolactone) Scaffold Integrated with Cell-Laden Chitosan Hydrogels for Bone Tissue Engineering, Sci. Rep., 2017, 7(1), 13412, DOI:10.1038/s41598-017-13838-7.
- Y. Pan, C. Xiao, H. Tan, G. Yuan, J. Li, S. Li, Y. Jia, D. Xiong, X. Hu and X. Niu, Covalently Injectable Chitosan/Chondroitin Sulfate Hydrogel Integrated Gelatin/Heparin Microspheres for Soft Tissue Engineering, Int. J. Polym. Mater. Polym. Biomater., 2019, 1–9, DOI:10.1080/00914037.2019.1695210.
- C. Antich, J. de Vicente, G. Jiménez, C. Chocarro, E. Carrillo, E. Montañez, P. Gálvez-Martín and J. A. Marchal, Bio-Inspired Hydrogel Composed of Hyaluronic Acid and Alginate as a Potential Bioink for 3D Bioprinting of Articular Cartilage Engineering Constructs, Acta Biomater., 2020, 106, 114–123, DOI:10.1016/j.actbio.2020.01.046.
- Y. Gao, A. Sarode, N. Kokoroskos, A. Ukidve, Z. Zhao, S. Guo, R. Flaumenhaft, A. S. Gupta, N. Saillant and S. Mitragotri, A Polymer-Based Systemic Hemostatic Agent, Sci. Adv., 2020, 6(31), eaba0588, DOI:10.1126/sciadv.aba0588.
- Y. D. P. Limasale, P. Atallah, C. Werner, U. Freudenberg and R. Zimmermann, Tuning the Local Availability of VEGF within Glycosaminoglycan–Based Hydrogels to Modulate Vascular Endothelial Cell Morphogenesis, Adv. Funct. Mater., 2020, 2000068, DOI:10.1002/adfm.202000068.
- M. H. Hettiaratchi, T. Miller, J. S. Temenoff, R. E. Guldberg and T. C. McDevitt, Heparin Microparticle Effects on Presentation and Bioactivity of Bone Morphogenetic Protein-2, Biomaterials, 2014, 35(25), 7228–7238, DOI:10.1016/j.biomaterials.2014.05.011.
- T. E. Rinker, B. D. Philbrick and J. S. Temenoff, Core-Shell Microparticles for Protein Sequestration and Controlled Release of a Protein-Laden Core, Acta Biomater., 2017, 56, 91–101, DOI:10.1016/j.actbio.2016.12.042.
- P. Olczyk, Ł. Mencner and K. Komosinska-Vassev, Diverse Roles of Heparan Sulfate and Heparin in Wound Repair, BioMed Res. Int., 2015, 2015, 1–7, DOI:10.1155/2015/549417.
- J. Hogwood, A. Naggi, G. Torri, C. Page, P. Rigsby, B. Mulloy and E. Gray, The Effect of Increasing the Sulfation Level of Chondroitin Sulfate on Anticoagulant Specific Activity and Activation of the Kinin System, PLoS One, 2018, 13(3), e0193482, DOI:10.1371/journal.pone.0193482.
- D. S. Bhattacharya, D. Svechkarev, A. Bapat, P. Patil, M. A. Hollingsworth and A. M. Mohs, Sulfation Modulates the Targeting Properties of Hyaluronic Acid to P-Selectin and CD44, ACS Biomater. Sci. Eng., 2020, 6(6), 3585–3598, DOI:10.1021/acsbiomaterials.0c00115.
- Y. Peng, L. E. Tellier and J. S. Temenoff, Heparin-Based Hydrogels with Tunable Sulfation & Degradation for Anti-Inflammatory Small Molecule Delivery, Biomater. Sci., 2016, 4(9), 1371–1380, 10.1039/C6BM00455E.
- R. Subbiah, A. Cheng, M. A. Ruehle, M. H. Hettiaratchi, L. E. Bertassoni and R. E. Guldberg, Effects of Controlled Dual Growth Factor Delivery on Bone Regeneration Following Composite Bone-Muscle Injury, Acta Biomater., 2020, 114, 63–75, DOI:10.1016/j.actbio.2020.07.026.
- A. Zieris, K. Chwalek, S. Prokoph, K. R. Levental, P. B. Welzel, U. Freudenberg and C. Werner, Dual Independent Delivery of Pro-Angiogenic Growth Factors from StarPEG-Heparin Hydrogels, J. Controlled Release, 2011, 156(1), 28–36, DOI:10.1016/j.jconrel.2011.06.042.
- M. H. Hettiaratchi, C. Chou, N. Servies, J. M. Smeekens, A. Cheng, C. Esancy, R. Wu, T. C. McDevitt, R. E. Guldberg and L. Krishnan, Competitive Protein Binding Influences Heparin-Based Modulation of Spatial Growth Factor Delivery for Bone Regeneration, Tissue Eng., Part A, 2017, 23(13–14), 683–695, DOI:10.1089/ten.tea.2016.0507.
- M. R. Casanova, C. Oliveira, E. M. Fernandes, R. L. Reis, T. H. Silva, A. Martins and N. M. Neves, Spatial Immobilization of Endogenous Growth Factors to Control Vascularization in Bone Tissue Engineering, Biomater. Sci., 2020, 8(9), 2577–2589, 10.1039/D0BM00087F.
- J. F. Crispim, S. C. Fu, Y. W. Lee, H. A. M. Fernandes, P. Jonkheijm, P. S. H. Yung and D. B. F. Saris, Bioactive Tape With BMP-2 Binding Peptides Captures Endogenous Growth Factors and Accelerates Healing After Anterior Cruciate Ligament Reconstruction, Am. J. Sports Med., 2018, 46(12), 2905–2914, DOI:10.1177/0363546518787507.
- J. Ishihara, K. Fukunaga, A. Ishihara, H. M. Larsson, L. Potin, P. Hosseinchi, G. Galliverti, M. A. Swartz and J. A. Hubbell, Matrix-Binding Checkpoint Immunotherapies Enhance Antitumor Efficacy and Reduce Adverse Events, Sci. Transl. Med., 2017, 9(415), eaan0401, DOI:10.1126/scitranslmed.aan0401.
- J. Crispim, H. A. M. Fernandes, S. C. Fu, Y. W. Lee, P. Jonkheijm and D. B. F. Saris, TGF-B1 Activation in Human Hamstring Cells through Growth Factor Binding Peptides on Polycaprolactone Surfaces, Acta Biomater., 2017, 53, 165–178, DOI:10.1016/j.actbio.2017.01.066.
- K. Katsumata, J. Ishihara, A. Mansurov, A. Ishihara, M. M. Raczy, E. Yuba and J. A. Hubbell, Targeting Inflammatory Sites through Collagen Affinity Enhances the Therapeutic Efficacy of Anti-Inflammatory Antibodies, Sci. Adv., 2019, 5(11), eaay1971, DOI:10.1126/sciadv.aay1971.
- N. K. Botelho, B. O. Tschumi, J. A. Hubbell, M. A. Swartz, A. Donda and P. Romero, Combination of Synthetic Long Peptides and XCL1 Fusion Proteins Results in Superior Tumor Control, Front. Immunol., 2019, 10, 294, DOI:10.3389/fimmu.2019.00294.
- P. Romero, A. Donda and J. A. Hubbell, An Optimized Antigen–Protein Fusion, Nat. Biomed. Eng., 2020, 4(6), 583–584, DOI:10.1038/s41551-020-0572-3.
- J.-M. Williford, J. Ishihara, A. Ishihara, A. Mansurov, P. Hosseinchi, T. M. Marchell, L. Potin, M. A. Swartz and J. A. Hubbell, Recruitment of CD103+Dendritic Cells via Tumor-Targeted Chemokine Delivery Enhances Efficacy of Checkpoint Inhibitor Immunotherapy, Sci. Adv., 2019, 5(12), eaay1357, DOI:10.1126/sciadv.aay1357.
- V. Delplace, A. Ortin-Martinez, E. L. S. Tsai, A. N. Amin, V. Wallace and M. S. Shoichet, Controlled Release Strategy Designed for Intravitreal Protein Delivery to the Retina, J. Controlled Release, 2019, 293, 10–20, DOI:10.1016/j.jconrel.2018.11.012.
- S. Nori, M. Khazaei, C. S. Ahuja, K. Yokota, J.-E. Ahlfors, Y. Liu, J. Wang, S. Shibata, J. Chio, M. H. Hettiaratchi, T. Führmann, M. S. Shoichet and M. G. Fehlings, Human Oligodendrogenic Neural Progenitor Cells Delivered with Chondroitinase ABC Facilitate Functional Repair of Chronic Spinal Cord Injury, Stem Cell Rep., 2018, 11(6), 1433–1448, DOI:10.1016/j.stemcr.2018.10.017.
- A. Stejskalová, N. Oliva, F. J. England and B. D. Almquist, Biologically Inspired, Cell-Selective Release of Aptamer-Trapped Growth Factors by Traction Forces, Adv. Mater., 2019, 31(7), 1806380, DOI:10.1002/adma.201806380.
- Z. Feng, H. Wang and B. Xu, Instructed Assembly of Peptides for Intracellular Enzyme Sequestration, J. Am. Chem. Soc., 2018, 140(48), 16433–16437, DOI:10.1021/jacs.8b10542.
- K. Vulic and M. S. Shoichet, Tunable Growth Factor Delivery from Injectable Hydrogels for Tissue Engineering, J. Am. Chem. Soc., 2012, 134(2), 882–885, DOI:10.1021/ja210638x.
- M. M. Pakulska, K. Vulic and M. S. Shoichet, Affinity-Based Release of Chondroitinase ABC from a Modified Methylcellulose Hydrogel, J. Controlled Release, 2013, 171(1), 11–16, DOI:10.1016/j.jconrel.2013.06.029.
- M. H. Hettiaratchi, M. J. O'Meara, T. R. O'Meara, A. J. Pickering, N. Letko-Khait and M. S. Shoichet, Reengineering Biocatalysts: Computational Redesign of Chondroitinase ABC Improves Efficacy and Stability, Sci. Adv., 2020, 6(34), eabc6378, DOI:10.1126/sciadv.abc6378.
- B. R. Silverman and J. A. Champion, Presentation of Fibronectin Fragments Using Affinity Protein Interactions for Enhanced Retention and Function, Acta Biomater., 2014, 10(12), 4956–4960, DOI:10.1016/j.actbio.2014.08.026.
- D. R. Myers, J. Zikherman and J. P. Roose, Tonic Signals: Why Do Lymphocytes Bother?, Trends Immunol., 2017, 38(11), 844–857, DOI:10.1016/j.it.2017.06.010.
- T. E. Rinker, B. D. Philbrick, M. H. Hettiaratchi, D. M. Smalley, T. C. McDevitt and J. S. Temenoff, Microparticle-Mediated Sequestration of Cell-Secreted Proteins to Modulate Chondrocytic Differentiation, Acta Biomater., 2018, 68, 125–136, DOI:10.1016/j.actbio.2017.12.038.
- G. A. Hudalla, J. T. Koepsel and W. L. Murphy, Surfaces That Sequester Serum-Borne Heparin Amplify Growth Factor Activity, Adv. Mater., 2011, 23(45), 5415–5418, DOI:10.1002/adma.201103046.
- Y. Zeng, Y. V. Shih, G. S. Baht and S. Varghese, In Vivo Sequestration of Innate Small Molecules to Promote Bone Healing, Adv. Mater., 2020, 32(8), 1906022, DOI:10.1002/adma.201906022.
- G. Liu, R. Wu, B. Yang, Y. Shi, C. Deng, A. Atala, S. Mou, T. Criswell and Y. Zhang, A Cocktail of Growth Factors Released from a Heparin Hyaluronic-Acid Hydrogel Promotes the Myogenic Potential of Human Urine-Derived Stem Cells in Vivo, Acta Biomater., 2020, 107, 50–64, DOI:10.1016/j.actbio.2020.02.005.
- S. S. Lee, J. H. Kim, J. Jeong, S. H. L. Kim, R. H. Koh, I. Kim, S. Bae, H. Lee and N. S. Hwang, Sequential Growth Factor Releasing Double Cryogel System for Enhanced Bone Regeneration, Biomaterials, 2020, 257, 120223, DOI:10.1016/j.biomaterials.2020.120223.
- Y. Wang, M. J. Cooke, N. Sachewsky, C. M. Morshead and M. S. Shoichet, Bioengineered Sequential Growth Factor Delivery Stimulates Brain Tissue Regeneration after Stroke, J. Controlled Release, 2013, 172(1), 1–11, DOI:10.1016/j.jconrel.2013.07.032.
- N. J. Shah, M. N. Hyder, M. A. Quadir, N. M. Dorval Courchesne, H. J. Seeherman, M. Nevins, M. Spector and P. T. Hammond, Adaptive Growth Factor Delivery from a Polyelectrolyte Coating Promotes Synergistic Bone Tissue Repair and Reconstruction, Proc. Natl. Acad. Sci. U. S. A., 2014, 111(35), 12847–12852, DOI:10.1073/pnas.1408035111.
- P. M. Gawade, J. A. Shadish, B. A. Badeau and C. A. DeForest, Logic–Based Delivery of Site–Specifically Modified Proteins from Environmentally Responsive Hydrogel Biomaterials, Adv. Mater., 2019, 31(33), 1902462, DOI:10.1002/adma.201902462.
- B. A. Badeau, M. P. Comerford, C. K. Arakawa, J. A. Shadish and C. A. DeForest, Engineered Modular Biomaterial Logic Gates for Environmentally Triggered Therapeutic Delivery, Nat. Chem., 2018, 10(3), 251–258, DOI:10.1038/nchem.2917.
- P. J. LeValley, R. Neelarapu, B. P. Sutherland, S. Dasgupta, C. J. Kloxin and A. M. Kloxin, Photolabile Linkers: Exploiting Labile Bond Chemistry to Control Mode and Rate of Hydrogel Degradation and Protein Release, J. Am. Chem. Soc., 2020, 142(10), 4671–4679, DOI:10.1021/jacs.9b11564.
- Z. Li, G. Tian, H. Jiang, R. Pan, B. Lian, M. Wang, Z. Gao, B. Zhang and J. Wu, Liver-Targeting and PH-Sensitive Sulfated Hyaluronic Acid Mixed Micelles for Hepatoma Therapy, Int. J. Nanomedicine, 2019, 14, 9437–9452, DOI:10.2147/IJN.S214528.
- P. Zhang, F. Han, T. Chen, Z. Wu and S. Chen, “Swiss Roll”-like Bioactive Hybrid Scaffolds for Promoting Bone Tissue Ingrowth and Tendon-Bone Healing after Anterior Cruciate Ligament Reconstruction, Biomater. Sci., 2020, 8(3), 871–883, 10.1039/C9BM01703H.
- A. Ishihara, K. Sasaki, S. S.-Y. Lee, J.-M. Williford, J. Ishihara, M. Yasui, H. Abe, L. Potin, P. Hosseinchi, K. Fukunaga, M. M. Raczy, L. T. Gray, A. Mansurov, K. Katsumata, M. Fukayama, S. J. Kron, M. A. Swartz and J. A. Hubbell, Targeted Antibody and Cytokine Cancer Immunotherapies through Collagen Affinity, Sci. Transl. Med., 2019, 11(487), eaau3259 CrossRef.
- Z. F. Walls, M. Schwengels and V. Palau, Intracellular Sequestration of HER2 via Targeted Subcellular Peptide Delivery, J. Drug Targeting, 2018, 26(9), 840–844, DOI:10.1080/1061186X.2018.1450411.
- B. Chen, H. Lin, J. Wang, Y. Zhao, B. Wang, W. Zhao, W. Sun and J. Dai, Homogeneous Osteogenesis and Bone Regeneration by Demineralized Bone Matrix Loading with Collagen-Targeting Bone Morphogenetic Protein-2, Biomaterials, 2007, 28(6), 1027–1035, DOI:10.1016/j.biomaterials.2006.10.013.
- S. Zalba, A. M. Contreras, A. Haeri, T. L. M. ten Hagen, I. Navarro, G. Koning and M. J. Garrido, Cetuximab-Oxaliplatin-Liposomes for Epidermal Growth Factor Receptor Targeted Chemotherapy of Colorectal Cancer, J. Controlled Release, 2015, 210, 26–38, DOI:10.1016/j.jconrel.2015.05.271.
- H.-Y. Seok, N. Sanoj Rejinold, K. M. Lekshmi, K. Cherukula, I.-K. Park and Y.-C. Kim, CD44 Targeting Biocompatible and Biodegradable Hyaluronic Acid Cross-Linked Zein Nanogels for Curcumin Delivery to Cancer Cells: In Vitro and in Vivo Evaluation, J. Controlled Release, 2018, 280, 20–30, DOI:10.1016/j.jconrel.2018.04.050.
- X. Zhang, F. He, K. Xiang, J. Zhang, M. Xu, P. Long, H. Su, Z. Gan and Q. Yu, CD44-Targeted Facile Enzymatic Activatable Chitosan Nanoparticles for Efficient Antitumor Therapy and Reversal of Multidrug Resistance, Biomacromolecules, 2018, 19(3), 883–895, DOI:10.1021/acs.biomac.7b01676.
- L. Ledsgaard, M. Kilstrup, A. Karatt-Vellatt, J. McCafferty and A. H. Laustsen, Basics of Antibody Phage Display Technology, Toxins, 2018, 10(6), 236, DOI:10.3390/toxins10060236.
- T. I. Samoylova and B. F. Smith, Elucidation of Muscle–binding Peptides by Phage Display Screening, Muscle Nerve, 1999, 22(4), 460–466 CrossRef CAS.
- S. Kanki, D. E. Jaalouk, S. Lee, A. Y. C. Yu, J. Gannon and R. T. Lee, Identification of Targeting Peptides for Ischemic Myocardium by in Vivo Phage Display, J. Mol. Cell. Cardiol., 2011, 50(5), 841–848, DOI:10.1016/j.yjmcc.2011.02.003.
- S. J. Segvich, H. C. Smith and D. H. Kohn, The Adsorption of Preferential Binding Peptides to Apatite-Based Materials, Biomaterials, 2009, 30(7), 1287–1298, DOI:10.1016/j.biomaterials.2008.11.008.
- H. Ramaraju and D. H. Kohn, Cell and Material–Specific Phage Display Peptides Increase IPS–MSC Mediated Bone and Vasculature Formation In Vivo, Adv. Healthcare Mater., 2019, 8(9), 1801356, DOI:10.1002/adhm.201801356.
- L. Ding, Y. Jiang, J. Zhang, H.-A. Klok and Z. Zhong, PH-Sensitive Coiled-Coil Peptide-Cross-Linked Hyaluronic Acid Nanogels: Synthesis and Targeted Intracellular Protein Delivery to CD44 Positive Cancer Cells, Biomacromolecules, 2018, 19(2), 555–562, DOI:10.1021/acs.biomac.7b01664.
- C. G. Helguero, V. M. Mustahsan, S. Parmar, S. Pentyala, J. L. Pfail, I. Kao, D. E. Komatsu and S. Pentyala, Biomechanical Properties of 3D-Printed Bone Scaffolds Are Improved by Treatment with CRFP, J. Orthop. Surg. Res., 2017, 12(1), 195, DOI:10.1186/s13018-017-0700-2.
- W. Carver and E. C. Goldsmith, Regulation of Tissue Fibrosis by the Biomechanical Environment, BioMed Res. Int., 2013, 2013, 1–10, DOI:10.1155/2013/101979.
- S. Bansal, S. Mandalapu, C. Aeppli, F. Qu, S. E. Szczesny, R. L. Mauck and M. H. Zgonis, Mechanical Function near Defects in an Aligned Nanofiber Composite Is Preserved by Inclusion of Disorganized Layers: Insight into Meniscus Structure and Function, Acta Biomater., 2017, 56, 102–109, DOI:10.1016/j.actbio.2017.01.074.
- X. Cheng, C. Tsao, V. L. Sylvia, D. Cornet, D. P. Nicolella, T. L. Bredbenner and R. J. Christy, Platelet-Derived Growth-Factor-Releasing Aligned Collagen–Nanoparticle Fibers Promote the Proliferation and Tenogenic Differentiation of Adipose-Derived Stem Cells, Acta Biomater., 2014, 10(3), 1360–1369, DOI:10.1016/j.actbio.2013.11.017.
- R. Dong, P. X. Ma and B. Guo, Conductive biomaterials for muscle tissue engineering, Biomaterials, 2020, 229, 119584, DOI:10.1016/j.biomaterials.2019.119584.
- N. Narayanan, C. Jiang, C. Wang, G. Uzunalli, N. Whittern, D. Chen, O. G. Jones, S. Kuang and M. Deng, Harnessing Fiber Diameter-Dependent Effects of Myoblasts Toward Biomimetic Scaffold-Based Skeletal Muscle Regeneration, Front. Bioeng. Biotechnol., 2020, 8, 203, DOI:10.3389/fbioe.2020.00203.
- S. R. Inamdar, E. Barbieri, N. J. Terrill, M. M. Knight and H. S. Gupta, Proteoglycan Degradation Mimics Static Compression by Altering the Natural Gradients in Fibrillar Organisation in Cartilage, Acta Biomater., 2019, 97, 437–450, DOI:10.1016/j.actbio.2019.07.055.
- A. J. Sophia Fox, A. Bedi and S. A. Rodeo, The Basic Science of Articular Cartilage: Structure, Composition, and Function, Sports Health, 2009, 1(6), 461–468, DOI:10.1177/1941738109350438.
- K. Parratt, M. Smerchansky, Q. Stiggers and K. Roy, Effect of Hydrogel Material Composition on HBMSC Differentiation into Zone-Specific Neo-Cartilage: Engineering Human Articular Cartilage-like Tissue with Spatially Varying Properties, J. Mater. Chem. B, 2017, 5(31), 6237–6248, 10.1039/C7TB00896A.
- C. Gegg and F. Yang, Spatially Patterned Microribbon-Based Hydrogels Induce Zonally-Organized Cartilage Regeneration by Stem Cells in 3D, Acta Biomater., 2020, 101, 196–205, DOI:10.1016/j.actbio.2019.10.025.
- A. F. Girão, Â. Semitela, G. Ramalho, A. Completo and P. A. A. P. Marques, Mimicking Nature: Fabrication of 3D Anisotropic Electrospun Polycaprolactone Scaffolds for Cartilage Tissue Engineering Applications, Composites, Part B, 2018, 154, 99–107, DOI:10.1016/j.compositesb.2018.08.001.
- J. Wang, Y. Wang, X. Sun, D. Liu, C. Huang, J. Wu, C. Yang and Q. Zhang, Biomimetic Cartilage Scaffold with Orientated Porous Structure of Two Factors for Cartilage Repair of Knee Osteoarthritis, Artif. Cells, Nanomed., Biotechnol., 2019, 47(1), 1710–1721, DOI:10.1080/21691401.2019.1607866.
- S. Camarero-Espinosa, A. Calore, A. Wilbers, J. Harings and L. Moroni, Additive Manufacturing of an Elastic Poly(Ester)Urethane for Cartilage Tissue Engineering, Acta Biomater., 2020, 102, 192–204, DOI:10.1016/j.actbio.2019.11.041.
- A. Di Luca, M. Klein-Gunnewiek, J. G. Vancso, C. A. van Blitterswijk, E. M. Benetti and L. Moroni, Covalent Binding of Bone Morphogenetic Protein-2 and Transforming Growth Factor-B3 to 3D Plotted Scaffolds for Osteochondral Tissue Regeneration, Biotechnol. J., 2017, 12(12), 1700072, DOI:10.1002/biot.201700072.
- S. Lu, J. Lam, J. E. Trachtenberg, E. J. Lee, H. Seyednejad, J. J. J. P. van den Beucken, Y. Tabata, M. E. Wong, J. A. Jansen, A. G. Mikos and F. K. Kasper, Dual Growth Factor Delivery from Bilayered, Biodegradable Hydrogel Composites for Spatially-Guided Osteochondral Tissue Repair, Biomaterials, 2014, 35(31), 8829–8839, DOI:10.1016/j.biomaterials.2014.07.006.
- N. Oliva and B. D. Almquist, Spatiotemporal Delivery of Bioactive Molecules for Wound Healing Using Stimuli-Responsive Biomaterials, Adv. Drug Delivery Rev., 2020, 161–162, 22–41, DOI:10.1016/j.addr.2020.07.021.
- L. Massi, A. Najer, R. Chapman, C. D. Spicer, V. Nele, J. Che, M. A. Booth, J. J. Doutch and M. M. Stevens, Tuneable Peptide Cross-Linked Nanogels for Enzyme-Triggered Protein Delivery, J. Mater. Chem. B, 2020, 8(38), 8894–8907, 10.1039/D0TB01546F.
- S. Patel, R. Vaishya, X. Yang, D. Pal and A. Mitra, Novel Thermosensitive Pentablock Copolymers for Sustained Delivery of Proteins in the Treatment of Posterior Segment Diseases, PPL, 2014, 21(11), 1185–1200, DOI:10.2174/092986652111141001122054.
- Y. S. Kim, M. Gulfam and T. L. Lowe, Thermoresponsive- Co -Biodegradable Linear–Dendritic Nanoparticles for Sustained Release of Nerve Growth Factor To Promote Neurite Outgrowth, Mol. Pharmaceutics, 2018, 15(4), 1467–1475, DOI:10.1021/acs.molpharmaceut.7b01044.
- J. Liu, C. Detrembleur, M.-C. De Pauw-Gillet, S. Mornet, L. V. Elst, S. Laurent, C. Jérôme and E. Duguet, Heat-Triggered Drug Release Systems Based on Mesoporous Silica Nanoparticles Filled with a Maghemite Core and Phase-Change Molecules as Gatekeepers, J. Mater. Chem. B, 2014, 2(1), 59–70, 10.1039/C3TB21229G.
- B. C. Geiger, S. Wang, R. F. Padera, A. J. Grodzinsky and P. T. Hammond, Cartilage-Penetrating Nanocarriers Improve Delivery and Efficacy of Growth Factor Treatment of Osteoarthritis, Sci. Transl. Med., 2018, 10(469), eaat8800, DOI:10.1126/scitranslmed.aat8800.
- F. Mahmoodzadeh, M. Ghorbani and B. Jannat, Glutathione and PH-Responsive Chitosan-Based Nanogel as an Efficient Nanoplatform for Controlled Delivery of Doxorubicin, J. Drug Delivery Sci. Technol., 2019, 54, 101315, DOI:10.1016/j.jddst.2019.101315.
- J. Wu, Y. Yang, S. Li, A. Shi, B. Song, S. Niu, W. Chen and Z. Yao, Glucose-Sensitive Nanoparticles Based On Poly(3-Acrylamidophenylboronic Acid-Block-N-Vinylcaprolactam) For Insulin Delivery, Int. J. Nanomedicine, 2019, 14, 8059–8072, DOI:10.2147/IJN.S220936.
- S. Belbekhouche, S. Charaabi, L. Picton, D. Le Cerf and B. Carbonnier, Glucose-Sensitive Polyelectrolyte Microcapsules Based on (Alginate/Chitosan) Pair, Carbohydr. Polym., 2018, 184, 144–153, DOI:10.1016/j.carbpol.2017.12.054.
- X. Liang, M. Trentle, V. Kozlovskaya, E. Kharlampieva and M. Bonizzoni, Carbohydrate Sensing Using Water-Soluble Poly(Methacrylic Acid)- Co-3-(Acrylamido)Phenylboronic Acid Copolymer, ACS Appl. Polym. Mater., 2019, 1(6), 1341–1349, DOI:10.1021/acsapm.9b00141.
- Z. Cong, Y. Shi, Y. Wang, Y. Wang, J. Niu, N. Chen and H. Xue, A Novel Controlled Drug Delivery System Based on Alginate Hydrogel/Chitosan Micelle Composites, Int. J. Biol. Macromol., 2018, 107, 855–864, DOI:10.1016/j.ijbiomac.2017.09.065.
- P. Treenate and P. Monvisade, In Vitro Drug Release Profiles of PH-Sensitive Hydroxyethylacryl Chitosan/Sodium Alginate Hydrogels Using Paracetamol as a Soluble Model Drug, Int. J. Biol. Macromol., 2017, 99, 71–78, DOI:10.1016/j.ijbiomac.2017.02.061.
- W. C. Ballance, E. C. Qin, H. J. Chung, M. U. Gillette and H. Kong, Reactive Oxygen Species-Responsive Drug Delivery Systems for the Treatment of Neurodegenerative Diseases, Biomaterials, 2019, 217, 119292, DOI:10.1016/j.biomaterials.2019.119292.
- J. L. Holloway, H. Ma, R. Rai and J. A. Burdick, Modulating Hydrogel Crosslink Density and Degradation to Control Bone Morphogenetic Protein Delivery and in Vivo Bone Formation, J. Controlled Release, 2014, 191, 63–70, DOI:10.1016/j.jconrel.2014.05.053.
- J. Park, J. H. Choi, S. Kim, I. Jang, S. Jeong and J. Y. Lee, Micropatterned Conductive Hydrogels as Multifunctional Muscle-Mimicking Biomaterials: Graphene-Incorporated Hydrogels Directly Patterned with Femtosecond Laser Ablation, Acta Biomater., 2019, 97, 141–153, DOI:10.1016/j.actbio.2019.07.044.
- D. M. Pedrotty, V. Kuzmenko, E. Karabulut, A. M. Sugrue, C. Livia, V. R. Vaidya, C. J. McLeod, S. J. Asirvatham, P. Gatenholm and S. Kapa, Three-Dimensional Printed Biopatches With Conductive Ink Facilitate Cardiac Conduction When Applied to Disrupted Myocardium, Circ.: Arrhythmia Electrophysiol., 2019, 12(3), e006920, DOI:10.1161/circep.118.006920.
- R. Feiner, L. Engel, S. Fleischer, M. Malki, I. Gal, A. Shapira, Y. Shacham-Diamand and T. Dvir, Engineered Hybrid Cardiac Patches with Multifunctional Electronics for Online Monitoring and Regulation of Tissue Function, Nat. Mater., 2016, 15(6), 679–685, DOI:10.1038/nmat4590.
- F. E. Freeman, P. Pitacco, L. H. A. van Dommelen, J. Nulty, D. C. Browe, J.-Y. Shin, E. Alsberg and D. J. Kelly, 3D Bioprinting Spatiotemporally Defined Patterns of Growth Factors to Tightly Control Tissue Regeneration, Sci. Adv., 2020, 6(33), eabb5093, DOI:10.1126/sciadv.abb5093.
- W. J. Grigsby, S. M. Scott, M. I. Plowman-Holmes, P. G. Middlewood and K. Recabar, Combination and Processing Keratin with Lignin as Biocomposite Materials for Additive Manufacturing Technology, Acta Biomater., 2020, 104, 95–103, DOI:10.1016/j.actbio.2019.12.026.
- P. Camacho, H. Busari, K. B. Seims, P. Schwarzenberg, H. L. Dailey and L. W. Chow, 3D Printing with Peptide–Polymer Conjugates for Single-Step Fabrication of Spatially Functionalized Scaffolds, Biomater. Sci., 2019, 7(10), 4237–4247, 10.1039/C9BM00887J.
- R. J. Nagao, R. Marcu, Y. Wang, L. Wang, C. Arakawa, C. DeForest, J. Chen, J. A. López and Y. Zheng, Transforming Endothelium with Platelet–Rich Plasma in Engineered Microvessels, Adv. Sci., 2019, 6(24), 1901725, DOI:10.1002/advs.201901725.
- C. Arakawa, C. Gunnarsson, C. Howard, M. Bernabeu, K. Phong, E. Yang, C. A. DeForest, J. D. Smith and Y. Zheng, Biophysical and Biomolecular Interactions of Malaria-Infected Erythrocytes in Engineered Human Capillaries, Sci. Adv., 2020, 6(3), eaay7243, DOI:10.1126/sciadv.aay7243.
- M. Zhang, R. Lin, X. Wang, J. Xue, C. Deng, C. Feng, H. Zhuang, J. Ma, C. Qin, L. Wan, J. Chang and C. Wu, 3D Printing of Haversian Bone–Mimicking Scaffolds for Multicellular Delivery in Bone Regeneration, Sci. Adv., 2020, 6(12), eaaz6725, DOI:10.1126/sciadv.aaz6725.
- C. W. Peak, K. A. Singh, M. Adlouni, J. Chen and A. K. Gaharwar, Printing Therapeutic Proteins in 3D Using Nanoengineered Bioink to Control and Direct Cell Migration, Adv. Healthcare Mater., 2019, 8(11), 1801553, DOI:10.1002/adhm.201801553.
- R. Subbiah and R. E. Guldberg, Materials Science and Design Principles of Growth Factor Delivery Systems in Tissue Engineering and Regenerative Medicine, Adv. Healthcare Mater., 2019, 8(1), 1801000, DOI:10.1002/adhm.201801000.
- R. Subbiah, C. Hipfinger, A. Tahayeri, A. Athirasala, S. Horsophonphong, G. Thrivikraman, C. M. França, D. A. Cunha, A. Mansoorifar, A. Zahariev, J. M. Jones, P. G. Coelho, L. Witek, H. Xie, R. E. Guldberg and L. E. Bertassoni, 3D Printing of Microgel-Loaded Modular Microcages as Instructive Scaffolds for Tissue Engineering, Adv. Mater., 2020, 32(36), 2001736, DOI:10.1002/adma.202001736.
- S. S. Usmani, G. Bedi, J. S. Samuel, S. Singh, S. Kalra, P. Kumar, A. A. Ahuja, M. Sharma, A. Gautam and G. P. S. Raghava, THPdb: Database of FDA-Approved Peptide and Protein Therapeutics, PLoS One, 2017, 12(7), e0181748, DOI:10.1371/journal.pone.0181748.
- R. S. Thies, M. Bauduy, B. A. Ashton, L. Kurtzberg, J. M. Wozney and V. Rosen, Recombinant Human Bone Morphogenetic Protein-2 Induces Osteoblastic Differentiation in W-20-17 Stromal Cells, Endocrinology, 1992, 130(3), 1318–1324, DOI:10.1210/endo.130.3.1311236.
- P. J. Boyne, Animal Studies of the Applications of RhBMP-2 in Maxillofacial Reconstruction, Bone, 1996, 19(1), S83–S92, DOI:10.1016/S8756-3282(96)00144-5.
- G. E. Riedel and A. Valentin-Opran, Clinical Evaluation of RhBMP-2/ACS in Orthopedic Trauma: A Progress Report, Orthopedics, 1999, 22(7), 663–665 CAS.
- W. F. McKay, S. M. Peckham and J. M. Badura, A Comprehensive Clinical Review of Recombinant Human Bone Morphogenetic Protein-2 (INFUSE® Bone Graft), Int. Orthop., 2007, 31(6), 729–734, DOI:10.1007/s00264-007-0418-6.
- J. Lei, L. B. Priddy, J. J. Lim, M. Massee and T. J. Koob, Identification of Extracellular Matrix Components and Biological Factors in Micronized Dehydrated Human Amnion/Chorion Membrane, Adv. Wound Care, 2016, 6(2), 43–53, DOI:10.1089/wound.2016.0699.
- H. Niknejad, H. Peirovi, M. Jorjani, A. Ahmadiani, J. Ghanavi and A. M. Seifalian, Properties of the Amniotic Membrane for Potential Use in Tissue Engineering, Eur. Cells Mater., 2008, 15, 88–99, DOI:10.22203/ecm.v015a07.
- R. G. Frykberg, G. W. Gibbons, J. L. Walters, D. K. Wukich and F. C. Milstein, A Prospective, Multicentre, Open-Label, Single-Arm Clinical Trial for Treatment of Chronic Complex Diabetic Foot Wounds with Exposed Tendon and/or Bone: Positive Clinical Outcomes of Viable Cryopreserved Human Placental Membrane, Int. Wound J., 2017, 14(3), 569–577, DOI:10.1111/iwj.12649.
- J. Brandeisky and D. Kurtz Phelan, Clinical Outcome of Achilles Tendon Repair Using Viable Intact Cryopreserved Umbilical Tissue Versus Standard of Case, Wounds, 2017, 29(11), E111–E114 Search PubMed.
- R. Narayan, Amniotic Membranes in Ocular Surface Therapy, Optician Select, 2016, 2016(3), 119–111, DOI:10.12968/opti.2016.3.119.
- K. Suri, M. Kosker, I. M. Raber, K. M. Hammersmith, P. K. Nagra, B. D. Ayres, C. P. Halfpenny and C. J. Rapuano, Sutureless Amniotic Membrane ProKera for Ocular Surface Disorders: Short-Term Results, Eye Contact Lens, 2013, 39(5), 341–347, DOI:10.1097/ICL.0b013e3182a2f8fa.
- A. Ha, E. T. Criman, W. E. Kurata, K. W. Matsumoto and L. M. Pierce, Evaluation of a Novel Hybrid Viable Bioprosthetic Mesh in a Model of Mesh Infection, Plast. Reconstr. Surg. Glob. Open, 2017, 5(8), e1418, DOI:10.1097/GOX.0000000000001418.
- A. Arslan-Yildiz, R. E. Assal, P. Chen, S. Guven, F. Inci and U. Demirci, Towards Artificial Tissue Models: Past, Present, and Future of 3D Bioprinting, Biofabrication, 2016, 8(1), 014103, DOI:10.1088/1758-5090/8/1/014103.
- A. J. Engler and J. Cooper-White, Academic vs Industry Perspectives in 3D Bioprinting, APL Bioeng., 2020, 4(1), 010401, DOI:10.1063/5.0004340.
- M. H. Hettiaratchi, A. Schudel, T. Rouse, A. J. García, S. N. Thomas, R. E. Guldberg and T. C. McDevitt, A Rapid Method for Determining Protein Diffusion through Hydrogels for Regenerative Medicine Applications, APL Bioeng., 2018, 2(2), 026110, DOI:10.1063/1.4999925.
- F. Xu, B. Corbett, S. Bell, C. Zhang, M. Budi Hartono, Z. J. Farsangi, J. MacGregor and T. Hoare, High-Throughput Synthesis, Analysis, and Optimization of Injectable Hydrogels for Protein Delivery, Biomacromolecules, 2020, 21(1), 214–229, DOI:10.1021/acs.biomac.9b01132.
- D. Hao, Y. Fan, W. Xiao, R. Liu, C. Pivetti, T. Walimbe, F. Guo, X. Zhang, D. L. Farmer, F. Wang, A. Panitch, K. S. Lam and A. Wang, Rapid Endothelialization of Small Diameter Vascular Grafts by a Bioactive Integrin-Binding Ligand Specifically Targeting Endothelial Progenitor Cells and Endothelial Cells, Acta Biomater., 2020, 108, 178–193, DOI:10.1016/j.actbio.2020.03.005.
- M. S. Zambrano-Mila, K. E. S. Blacio and N. S. Vispo, Peptide Phage Display: Molecular Principles and Biomedical Applications, Ther. Innov. Regul. Sci., 2020, 54(2), 308, DOI:10.1007/s43441-019-00059-5.
- M. M. Pakulska, K. Vulic, R. Y. Tam and M. S. Shoichet, Hybrid Crosslinked Methylcellulose Hydrogel: A Predictable and Tunable Platform for Local Drug Delivery, Adv. Mater., 2015, 27(34), 5002–5008, DOI:10.1002/adma.201502767.
- K. C. Murphy, J. Whitehead, P. C. Falahee, D. Zhou, S. I. Simon and J. K. Leach, Multifactorial Experimental Design to Optimize the Anti-Inflammatory and Proangiogenic Potential of Mesenchymal Stem Cell Spheroids: Optimizing MSC Spheroid Potential, Stem Cells, 2017, 35(6), 1493–1504, DOI:10.1002/stem.2606.
- N. N. T. Le, T. L. Liu, J. Johnston, J. D. Krutty, K. M. Templeton, V. Harms, A. Dias, H. Le, P. Gopalan and W. L. Murphy, Customized Hydrogel Substrates for Serum-Free Expansion of Functional HMSCs, Biomater. Sci., 2020, 8(14), 3819–3829, 10.1039/D0BM00540A.
|
This journal is © The Royal Society of Chemistry 2021 |
Click here to see how this site uses Cookies. View our privacy policy here.