DOI:
10.1039/D0CE01195A
(Paper)
CrystEngComm, 2021,
23, 221-226
High dual-state blue emission of a functionalized pyrazoline derivative for picric acid detection†
Received
15th August 2020
, Accepted 6th November 2020
First published on 10th November 2020
Abstract
Developing dual-state fluorescence materials with high emission efficiency both in solution and solid states is still a challenge. Herein, a functionalized pyrazoline derivative (NF) was designed and synthesized. NF exhibited high blue emission efficiency in both THF solution and solid states with the absolute fluorescence quantum yields of 58% and 43%, respectively. The mechanism of this unique fluorescence behavior was systematically studied with the help of single-crystal structure analysis. The results showed that a close-knit molecular structure existed due to intramolecular C–H⋯π interactions, which can impair the intramolecular rotation to accelerate the radiative transition in the mono-molecule state (in dilute solution). Moreover, the steric hindrance and intermolecular hydrogen-bond interactions of the substituent groups can restrict the close packing and prompt the formation of J-aggregates in the aggregation state, which resulted in high emission performance in the solid state. Meanwhile, the dual-state blue emission property of NF was utilized for the picric acid (PA) detection in the solution state and on a test paper by the synergistic effects of photo-induced electron transfer (PET) and Förster resonance energy transfer (FRET) processes. The limit of detection as low as 6.80 × 10−7 M achieved in the THF–H2O mixture. This work provided a new scope for the design of high dual-state blue emission materials.
1. Introduction
The design and development of organic high fluorescent materials are of fundamental importance due to the wide applications in the fields of fluorescence bioimaging, chemosensors, organic light-emitting diodes and organic semiconductors.1–5 However, traditional organic luminophores in the solid state have faced great challenges because of their notorious aggregation-induced quenching (ACQ) behaviors. In contrast, luminogens with aggregation-induced emission (AIEgens) can emit intense luminescence in the aggregated or solid state.6–10 Nevertheless, incompatible solution/aggregation fluorescence means a large gap between ACQgens and AIEgens, thereby driving the development of unprecedented luminogens with efficient dual-state emission (DSEgens) in both solution and solid states.11–13 These DSEgens broaden the applications of the luminescent materials such as in biosensing and organic optoelectronic systems.14–16
To gain excellent DSEgens with highly efficient fluorescence, the rational design of molecules is critical for tuning the molecular photophysical properties.17,18 Few DSEgens with high fluorescence quantum yields have recently been reported. Typically, Tang et al. had obtained three DSEgens decorated with triphenylamine owing to the rigidity of planarized conformation limiting intramolecular rotation and substantial twisting conformation preventing detrimental exciton interactions.19 Coincidentally, in 2019, Yang et al. designed and synthesized a new-style tetraphenylethylene DSEgen by locking its structure into the rigid framework.20 Our previous work has introduced a cyclohexyl structure of chair conformation into triphenylamine derivatives, whereby achieving dual-state emission.21 However, the emission color of developed DSEgens had not covered the whole visible region, particularly for pure blue emission materials. To develop new blue emission DSEgens as well as to explore the mechanism of molecular luminescence are crucial to facilitate practical applications.
Pyrazoline derivatives with good thermal stability and high fluorescence quantum yields have been recognized as excellent hole transport and emissive layer materials in organic electroluminescence devices.22,23 Therefore, we employed the fluorine atom and N,N-dimethylphenyl to modify the pyrazoline unit to design and synthesize the twisted and partial planarization molecule NF. We expect the linear conjugated structure and the relative small distance between the different substituent groups to impede the intramolecular motion to enhance the emission efficiency in the solution state. The steric hindrance and intermolecular interactions of fluorophenyl could induce the molecule to form J-aggregates to achieve the bright emission in the solid state. The relevant luminescence mechanism was systematically investigated by a structure–property study. In addition, the high dual-state emission luminogen NF could be utilized to trace the presence of picric acid (PA) selectively and sensitively in solution and solid states.24–28 The recognition properties of NF towards PA were studied, and the corresponding sensor mechanism was analyzed by absorption and fluorescence spectroscopies, 1H NMR and density functional theory (DFT) calculations.
2. Results and discussion
2.1 Materials and instruments
Intermediate compound ZF has been reported in literature reviewed.29 FT-IR spectra were recorded on a Nicolet NEXUS 380 spectrometer. NMR spectra were recorded on Brüker Avance 400, using TMS as the internal standard. Mass spectrometry (MS) analyses were conducted on an LTQ Orbitrap XL mass spectrometer. The UV-vis absorption spectra were recorded on a TU-1901 spectrophotometer. The fluorescence emission was investigated on a Hitachi FL-7000 spectrometer. The time-resolved PL decay spectra and absolute PL quantum yields (ΦPL) of the sample in solids were measured on a HORIBA FluoroMax-4P fluorescence spectrometer using an integrating sphere at room temperature (R. M.). The relative fluorescence quantum yields in solutions were measured by comparing to a standard (quinine sulfate in 0.1 M H2SO4). The digital photographs were captured by a Nikon D7000 camera. The thermogravimetry (TG) analysis was performed by TGA5500.
2.2 Synthesis
Pyrazoline derivative NF was synthesized via the Michael addition and cyclization reaction in a respectable yield, as shown in Scheme 1. 1H NMR and 13C NMR spectra of NF in DMSO-d6 are presented in Fig. S1 and S2.†
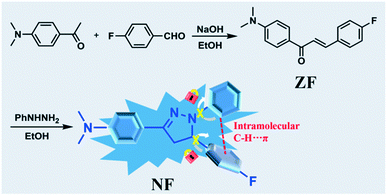 |
| Scheme 1 Synthetic routes of compound NF. | |
To get NF, compound ZF (200 mg, 0.74 mmol), phenylhydrazine (80 mg, 0.74 mmol) and HCl (5.0 mL, 1.0 mol L−1) were dissolved in 10 mL ethanol. The mixture was heated for 24 h at 80 °C. The precipitated product was filtered under a reduced pressure. The residue was purified via recrystallization in ethanol to afford NF as a white solid (107 mg, 40.1%). FT-IR (cm−1, KBr) v: 3120, 2910, 2805, 1655, 1597, 1509, 1498, 1511, 1388, 1356, 1324, 1297, 1228, 1195, 1160, 1138, 1117, 1099, 1000, 953, 866, 838, 820, 748, 692. 1H NMR (DMSO-d6, 400 MHz) δ (ppm): 7.53 (d, J = 9.00 Hz, 2H), 7.29–7.26 (m, 2H), 7.13–7.07 (m, 4H), 6.91–6.89 (m, 2H), 6.65–6.61 (m, 1H), 5.34 (dd, J1 = 6.28 Hz, J2 = 11.96 Hz, 1H), 3.80 (dd, J1 = 11.96 Hz, J2 = 17.28 Hz, 1H), 2.98 (dd, J1 = 6.28 Hz, J2 = 17.28 Hz, 1H), 2.91 (s, 6H). 13C NMR (DMSO-d6, 100 MHz) δ (ppm): 161.25 (d, 1JC–F = 239.29 Hz), 150.65, 148.00, 144.73, 138.99 (d, 4JC–F = 2.88 Hz), 128.77, 127.86 (d, 3JC–F = 4.59 Hz), 126.96, 119.66, 117.93, 115.66 (d, 2JC–F = 21.37 Hz), 112.65, 111.80, 62.12, 43.26. HR-MS (ESI†) (C23H23FN3) [MH]+: anal. calcd: 360.1876. Found: 360.1855.
2.3 Thermal analysis
The thermal stability of NF was conducted via thermogravimetric (TG) analysis. The compound was heated at 10 °C min−1 to detect the decomposition temperature under nitrogen atmosphere. As shown in Fig. S3,† the thermal decomposition temperature is 301.4 °C, which demonstrates that compound NF exhibits high thermal stability.
2.4 Photophysical properties both in solution and solid states
The UV-vis absorption and fluorescence properties of compound NF were determined in different solvents in Fig. 1 and S4,† and the results are shown in Table S1.† It can be seen that NF exhibited 365 nm maximum absorption band due to the ICT transition in different polar solutions, employed for the geometry optimization of pyrazoline NF using DFT methods (B3LYP/6-31g* level). Meanwhile, the fluorescence spectra of compound NF exhibited red-shifted in the ethanol (EtOH) comparing to that in benzene, dichloromethane (DCM) tetrahydrofuran (THF), ethyl acetate (EA), acetonitrile (MeCN), and N,N-dimethylformamide (DMF). The relatively bathochromic shift in the fluorescence spectra of EtOH was possibly attributed to the increasing dipole moment of the excited state by hydrogen bond interactions between molecules and EtOH solvents.30,31 Compound NF displayed high blue emission efficiencies of about 435 nm in solutions at the concentration of 10 μM (Fig. 1). The relative fluorescence quantum yields of the solutions are determined to be 40% in benzene, 51% in DCM, 58% in THF, 44% in EA, 53% in MeCN and 53% in DMF, respectively, but the value reduced to 25% in EtOH. The phenomena proved that hydrogen bonds between the molecular and solution can destroy a close-knit molecular structure, the breakdown of the C–H⋯π bond, accelerating molecular rotation to reduce fluorescence efficiency, and prompt to intense intramolecular charge transfer (ICT), dedicated to the redshift of fluorescence in EtOH.
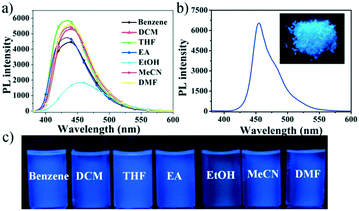 |
| Fig. 1 The PL spectra in different solvents (a) and in the solid state (b), inset: photo of the solid; (c) photos of compound NF in different solvents. | |
We further investigated the solid-state photophysical properties of compound NF (Fig. 1). The broad absorption and emission bands of NF in the solid state were subjected to a significant red-shift compared to that of in the THF solution, possibly due to the intermolecular interactions forming between NF molecules in the solid state. Furthermore, the absolute fluorescence quantum yields of compound NF was determined to be 58% in the THF solution state and 43% in the solid state (Fig. 1c). These results indicated that this molecule had the best of both ACQgens and AIEgens, achieving effective dual-state blue emission.
2.5 Crystal structure of compound NF
In order to investigate the mechanism of high fluorescence properties of the NF molecule, the single crystal was prepared through slowing the evaporation of its ethanol solution at room temperature. The single crystal structure is shown in Fig. 2, and the corresponding crystallographic data of NF are listed in Table S2.† The results revealed that NF crystallizes in an orthorhombic system. As shown in Fig. 1a, for crystal NF, the dihedral angles between the benzene ring (A, B and C) and the pyrazoline unit are 5.67°, 13.77° and 83.79°, respectively. The results indicated that NF possessed a twisted molecular conformation. Moreover, the hydrogen of the benzene ring (B) was connected with the benzene ring (C) by C–H⋯π (2.470 Å), which implied that intramolecular steric hindrance effectively limited molecular rotation. This might be the reason why luminogen NF, a close-knit molecular structure, exhibited efficiently high solution-state emission. Also, in Fig. 2c, the crystal NF has a non-coplanar molecular arrangement of a zigzag shape, thereby adopting a similar stacking mode of J-type aggregation. The π system of two adjacent molecules had no overlaps (Fig. 2c), which can prevent close π–π stacking. In addition, in the crystal structure, the molecules are stabilized by the C–H⋯π bond with a distance of 3.229 Å, and different pairs are linked by the C–H⋯F bond with a distance of 2.518 Å. The intermolecular interactions hindered molecular rotation and vibration that reduced the non-radiative transition to enhance the solid fluorescence in the crystal.
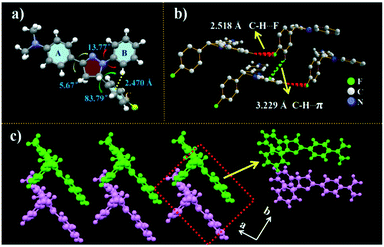 |
| Fig. 2 Crystal structures of NF: (a) selected dihedral angles along with the π-plane of NF; (b) intermolecular interactions; (c) zigzag stacking arrangement viewed along the a-axis. | |
The time-resolved emission decay behaviors of compound NF were further performed to testify high emission in both solution and solid states (Fig. S5†). NF showed an almost identical fluorescence lifetime in the THF solution and solid state. The phenomenon indicated that NF might possess a similar vibration mode under different states, resulting in similar excited state stability, which contributed to achieve high emission performance in both solids and solutions.
2.6 Nitro-explosive detection in an aqueous phase
Based on the properties of high dual-state emission, we explore whether compound NF can selectively and efficiently recognize picric acid (PA) in solution and solid states. First, NF was performed in the mixtures of THF/water (8
:
2, v/v) with the addition of 10 equiv. nitroaromatic compounds (2,4-dinitrotoluene (DNT), m-dihydroxybenzene (m-DOB), nitrobenzene (NB), o-nitrophenol (o-NP), p-dihydroxybenzene (p-DOM), p-methyl phenol (p-MP), p-nitrophenol (p-NP), p-nitrotoluene (p-NT), 2,4-dinitrophenol (DNP), and 2,4,6-trinitrophenol (PA)) in an aqueous medium. As shown in Fig. 3a, in the selective experiment, other nitro aromatics exhibited relatively little effect on the emission quenching compared with PA under the similar conditions. In Fig. 3b, the competitive experiment further demonstrated that NF can also show a remarkable response towards PA in the presence of various potentially interfering compounds. Thus, this indicated that NF can be considered as a promising luminescent probe for the selective detection of PA in actual samples. As shown in Fig. 3c, upon the addition of incremental amounts of 100 μM PA, quenching of the fluorescence emission at 450 nm can be observed. Quantitatively, these sensitivities of quenching effects can be explained by the Stern–Volmer equation I0/I = 1 + KSV[M], where I0 and I are fluorescence intensities before and after the addition of PA, respectively, [M] is the molar concentration of PA, and KSV is the quenching effect coefficient. The KSV of luminogen NF is determined to be 4.36 × 104 M−1 through the fitting of the linear parts of the plots. The limit of detection (LOD) of luminogen NF for PA is calculated using LOD = 3 σ/k, where σ is the standard deviation for a blank measurement, and k is the slope of a fluorescence intensity versus the PA concentration plot (Fig. S6†). The LOD of luminogen NF was calculated to be 6.80 × 10−7 M for PA, which made NF suitable to be a candidate of the fluorescence probe for the detection of PA.
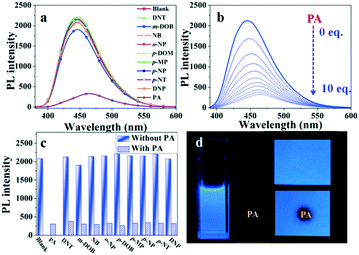 |
| Fig. 3 (a) PL spectra response of NF towards different analytes (10 equiv.) in the THF/water (v/v = 8 : 2) medium; (b) quenching percentages of compound NF (10.0 mM) with different analytes in THF/water (v/v = 8 : 2) mixtures before and after the addition of 10 equiv.; (c) fluorometric titration of NF towards PA (0–10 equiv.) in the THF/water (v/v = 8 : 2) medium; (d) photographs of the fluorescence quenching of compound NF for detecting PA in THF/water and Whatman filter papers. | |
To utilize the detection of PA in a feasible method, test strips were prepared by placing Whatman filter papers into dichloromethane solutions of NF for 1 min, and then drying it under vacuum. The test strips showed a strong blue fluorescence. As shown in Fig. 3d, upon the addition of incremental amounts of 10 mM PA, the fluorescence quenching decreased almost completely, and the results suggested that NF was an efficient fluorescent probe for conveniently detecting PA.
2.7 Quenching mechanism studies of sensing PA
In order to get insight into the quenching mechanism of luminogen NF for PA, the 1H NMR titration experiments of NF (Fig. 4) with PA (0–2 equiv.) in DMSO-d6 were conducted. The 1H NMR spectrum of NF showed resonances corresponding to the pyrazoline ring protons at 5.34, 3.80 and 2.98 ppm. The benzene ring of 1-(4-(dimethylamino)phenyl)ethanone protons appeared at 7.53, 7.10 and 2.91 ppm. The resonances corresponding to the benzene ring of phenylhydrazine protons appeared at 6.90, 6.70 and 6.63 ppm.
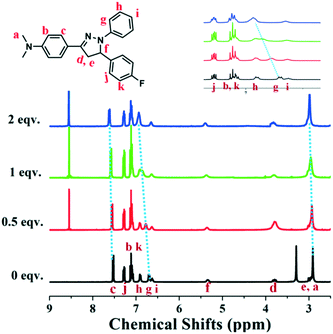 |
| Fig. 4 Partial 1H NMR titration spectra of NF upon the addition of PA (0, 0.5, 1, 2 equiv.) in DMSO-d6. | |
In contrast, after the addition of PA, 1H NMR spectra subtlety changed. With the continuous increase of PA, the protons of the N,N-dimethyl group gradually shifted downfield from 2.91 to 2.98 ppm (Δδ = 0.07) and protons labeled “c” were downfield shifted from 7.53 to 7.62 ppm (Δδ = 0.09). Meanwhile, protons labeled “g” was converted into obviously shifted to downfield from 6.70 to 6.94 ppm (Δδ = 0.24). These results made us assume that the interactions between NF and PA were weaker than protonation or the formation of a new chemical bond. The interaction might be the D–A interactions of electron deficiency PA and electron enriched NF. To further understand the interaction between the probe NF and PA, the fluorescence spectrum changes of the probe towards 10 equiv. trifluoroacetic acid (TFA) were evaluated. As shown in Fig. S7,† the fluorescence intensity of compound NF after adding TFA exhibited no emission quenching compared with that of PA, showing that it was indirectly suggested that there is no protonation effect between PA and NF molecules. These results demonstrated that there was no chemical reaction happened between PA and NF. However, the present PA could affect the electron cloud of NF by D–A interactions.
To explore the quenching mechanism of the compound NF towards PA, first, density functional theory (DFT) calculations were performed to investigate the frontier orbital energies of both NF and PA. In Fig. 5a, an estimation of the HOMO and LUMO energies of luminogen NF and PA via density functional theory (DFT) demonstrated that the LUMO of the electron deficient PA (−3.90 eV) is situated in between the HOMO and LUMO (−4.47 eV and −0.66 eV) of luminogen NF. Hence, the PET mechanism was expected from the LUMO of NF to PA, resulting in the fluorescence quenching. Second, the energy transfer might occur between the fluorophore and analyte, and if the fluorophore and the analyte were close to each other and the analyte absorption band effectively overlaps with the fluorophore emission band, the phenomenon is known as the Förster resonance energy transfer (FRET). In Fig. 5b, it was evident that there was a significant overlap between the absorption spectrum of PA and the emission spectrum of NF, which was observed to better understand the fluorescence quenching behavior of NF with PA. Hence, there was a strong possibility of FRET from π-electron-rich luminogen NF to electron-deficient PA, which can significantly enhance the quenching efficiency as well as sensitivity. These results are well in agreement with the fluorescence responses of NF to PA.
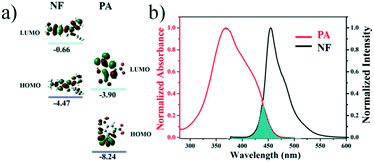 |
| Fig. 5 (a) HOMO and LUMO energy-level diagram of NF and PA; (b) the normalized UV-vis absorption spectra of PA and normalized fluorescence of NF. | |
Further, during the preparation and usage of nitroaromatics, the human body and other materials in the surroundings were contaminated with trace amounts of explosives. The detection of these traces of nitro explosives is major concern in the field of analytical and forensic sciences. In this context, we prepared test strips by dip-coating solutions of aggregates in THF on Whatman filter paper, followed by drying the strips under vacuum to test strips in different concentrations of PA. The fluorescence quenching of PA with various concentrations was performed in portable fluorescent test strips at 365 nm UV light, and the results indicated that the fluorescence quenching increased with the concentration of PA in range from 10−12 to 10−2 M (Fig. 6). These results validated the potential of the fluorescent NF as a fluorescent sensor for the local and instant detection of PA, and further efforts are still needed for this method and field.
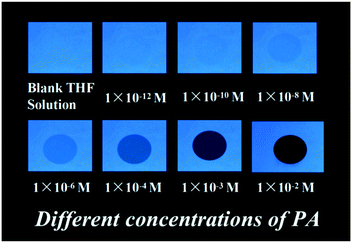 |
| Fig. 6 Photographs of fluorescence quenching of NF on test strips for detecting different concentrations of PA under 365 nm UV light. | |
3. Conclusion
We designed and synthesized a pyrazoline derivative NF displaying efficiently dual-state blue luminescence, which simultaneously surmounted the typical drawbacks of ACQgens and AIEgens. The fluorescence quantum yield of the blue-color luminogen reaches 58% and 43% in THF solution and solid states, respectively. The close-knit molecular structure, intramolecular steric hindrance, intermolecular interactions (C–H⋯π and C–H⋯F) and J-type aggregation accelerated the radiative transition to achieve highly dual-state emission of luminogen NF in solution and solid states. Another superb utilization of highly blue fluorescent NF had been executed as a fluorescence probe for detrimental explosive picric acid in an aqueous medium and on a test paper with the feature of high quenching efficiency. The quenching mechanism was attributed to the PET and FRET processes. This work stimulated and provided a basis for the further development of a novel small dual-state blue material, which enabled excellent optical performance for potential applications.
Conflicts of interest
The authors declare no conflicts of interest.
Acknowledgements
This work was supported by the National Natural Science Foundation of China (51673001, 51432001) and the Educational Commission of Anhui Province of China (KJ2019A0012).
Notes and references
- Z. X. Cai, N. Zhang, M. A. Awais, A. S. Filatov and L. P. Yu, Angew. Chem., Int. Ed., 2018, 57, 6442 CrossRef CAS.
- Y. X. Lei, W. B. Dai, Z. Q. Liu, S. Guo, Z. X. Cai, J. B. Shi, X. Y. Zheng, J. G. Zhi, B. Tong and Y. P. Dong, Mater. Chem. Front., 2019, 3, 284 RSC.
- P. Pallavi, V. Kumar, M. D. W. Hussain and A. Patra, ACS Appl. Mater. Interfaces, 2018, 10, 44696 CrossRef CAS.
- Q. Q. Shao, K. L. Liang, H. Ling, Y. G. Wang, Z. H. Yan, G. M. Xia and H. M. Wang, J. Mater. Chem. C, 2020, 8, 4549 RSC.
- Y. Q. Ma, Y. Y. Zhang, L. Kong and J. X. Yang, CrystEngComm, 2019, 21, 94 RSC.
- G. B. Zhang, Z. Li, F. J. Chen, D. T. Zhang, W. H. Ji, Z. P. Yang, Q. Wu, C. W. Zhang, L. Li and W. Huang, J. Innovative Opt. Health Sci., 2020, 03, 2050013 CrossRef.
- F. Tang, C. Wang, J. Wang, X. Wang and L. Li, ACS Appl. Mater. Interfaces, 2014, 6, 18337 CrossRef CAS.
- Z. Peng, Z. Wang, B. J. Shi, B. Tong and Y. P. Dong, Chin. J. Chem., 2016, 34, 1071 CrossRef CAS.
- H. Shi, H. Sun, H. Yang, S. Liu, G. Jenkins, W. Feng, F. Li, Q. Zhao, B. Liu and W. Huang, Adv. Funct. Mater., 2013, 23, 3268 CrossRef CAS.
- N. Goswami, Q. F. Yao, Z. T. Luo, J. G. Li, T. K. Chen and J. P. Xie, J. Phys. Chem. Lett., 2016, 7, 962 CrossRef CAS.
- M. Li, Y. Niu, X. Zhu, Q. Peng, H. Y. Lu, A. Xia and C. F. Chen, Chem. Commun., 2014, 50, 2993 RSC.
- D. Xi, Y. Z. Xu, R. H. Xu, Z. Wang, D. M. Liu, Q. F. Shen, L. Yue, D. F. Dang and L. J. Meng, Chem. – Eur. J., 2020, 26, 2741 CrossRef CAS.
- C. G. Wang, K. Wang, Q. Fu, J. Y. Zhang, D. G. Ma and Y. Wang, J. Mater. Chem. C, 2013, 1, 410 RSC.
- W. Li, D. Liu, F. Shen, D. Ma, Z. Wang, T. Feng, Y. Xu, B. Yang and Y. Ma, Adv. Funct. Mater., 2012, 22, 2797 CrossRef CAS.
- S. Jeong, M. K. Kim, S. H. Kim and J. I. Hong, Org. Electron., 2013, 14, 2497 CrossRef CAS.
- S. K. Sheet, B. Sen, S. K. Patra, M. Rabha, K. Aguan and S. Khatua, ACS Appl. Mater. Interfaces, 2018, 10, 14356 CrossRef CAS.
- H. Langhals, T. Potrawa, H. Noth and G. Linti, Angew. Chem., Int. Ed., 2003, 28, 478 CrossRef.
- M. Cai, Z. Gao, X. Zhou, X. Wang, S. Chen, Y. Zhao, Y. Qian, N. Shi, B. Mi, L. Xie and W. Huang, Phys. Chem. Chem. Phys., 2012, 14, 5289 RSC.
- G. Chen, W. Li, T. Zhou, Q. Peng, D. Zhai, H. Li, W. Yuan, Y. Zhang and B. Tang, Adv. Mater., 2015, 27, 4496 CrossRef CAS.
- X. J. Zheng, W. C. Zhu, C. Zhang, Y. Zhang, C. Zhong, H. Li, G. H. Xie, X. J. Wang and C. L. Yang, J. Am. Chem. Soc., 2019, 141, 4704 CrossRef CAS.
- Y. Y. Zhang, J. T. Pan, C. Y. Zhang, H. W. Wang, G. B. Zhang, L. Kong, Y. P. Tian and J. X. Yang, Dyes Pigm., 2015, 123, 257 CrossRef CAS.
- K. A. Haupa, A. Szukalski and J. Mysliwiec, J. Phys. Chem. A, 2018, 122, 7808 CrossRef CAS.
- A. Karuppusamy, R. Arulkumar, P. Kannana and P. Venuvanalingam, J. Photochem. Photobiol., A, 2019, 377, 247 CrossRef CAS.
- V. Bhalla, A. Gupta, M. Kumar, D. S. S. Rao and S. K. Prasad, ACS Appl. Mater. Interfaces, 2013, 5, 672 CrossRef CAS.
- A. P. Cando, D. Woitassek, G. Brunklausb and U. Scherf, Mater. Chem. Front., 2017, 1, 1118 RSC.
- V. Vij, V. Bhalla and M. Kumar, ACS Appl. Mater. Interfaces, 2013, 5, 5373 CrossRef CAS.
- Z. H. Fu, Y. W. Wang and Y. Peng, Chem. Commun., 2017, 53, 10524 RSC.
- S. Kaur, V. Bhalla, V. Vij and M. Kumar, J. Mater. Chem. C, 2014, 2, 3936 RSC.
- P. P. Maharjan, Q. L. Chen, L. J. Zhang, O. Adebanjo, N. Adhikari, S. Venkatesan, P. Adhikary, B. Vaagensmith and Q. Q. Qiao, Phys. Chem. Chem. Phys., 2013, 15, 6856 RSC.
- P. Galer, R. C. Korosec, M. Vidmar and B. Sket, J. Am. Chem. Soc., 2014, 136, 7383 CrossRef CAS.
- P. Sudhakar and T. P. Radhakrishnan, J. Mater. Chem. C, 2019, 7, 7083 RSC.
Footnote |
† Electronic supplementary information (ESI) available: NMR spectra, photophysical data, TG data, crystallographic tables. CCDC 1950610 (NF). For ESI and crystallographic data in CIF or other electronic format see DOI: 10.1039/d0ce01195a |
|
This journal is © The Royal Society of Chemistry 2021 |
Click here to see how this site uses Cookies. View our privacy policy here.