Uptake of perfluorinated alkyl acids by crops: results from a field study†
Received
25th April 2021
, Accepted 5th July 2021
First published on 14th July 2021
Abstract
Four crops with different edible plant parts (radish, lettuce, pea and maize) were grown in outdoor lysimeters on soil spiked with 13 perfluorinated alkyl acids (PFAAs) at 4 different levels. PFAA concentrations were measured in soil, soil pore water, and different plant parts at harvest. Edible part/soil concentration factors ranged over seven orders of magnitude and decreased strongly with increasing PFAA chain length, by a factor of 10 for each additional fluorinated carbon (nCF) for pea. Three processes were responsible for most of the variability. The first was sorption to soil; calculating whole plant concentration factors on the basis of concentration in pore water instead of soil reduced the variability from five orders of magnitude to two. Second, the journey of the PFAAs with the transpiration stream to the leaves was hindered by retention in the roots driven by sorption; root retention factors increased by a factor 1.7 for each nCF. Third, transfer of PFAAs from the leaves to the fruit via the phloem flow was also hindered – presumably by sorption; fruit/leaf concentration factors decreased by a factor 2.5 for each nCF. A simple mathematical model based on the above principles described the measured concentrations in roots, leaves, fruits and radish bulbs within a factor 4 in most cases. This indicates that the great diversity in PFAA transfer from soil to crops can be largely described with simple concepts for four markedly different species.
Environmental significance
Due to their persistence and hydrophilicity, many PFAAs have a strong potential to be taken up from soil into plants and to accumulate in foliage. There they can harm the plant and enter the food web, contributing to exposure of higher organisms including humans. Here we show that sorption to soil is the major process modulating plant uptake of PFAAs. Furthermore, we show that accumulation in the plant occurs primarily where the water is lost (in the leaves), while transport from there to fruits is inversely correlated with the tendency of the PFAA to sorb (chain length). Finally, we present a model that provides a simple framework for understanding and assessing PFAA accumulation in plants.
|
Introduction
In addition to having been detected ubiquitously in several environmental compartments including water,1,2 biota3 and the atmosphere,4 perfluorinated alkyl acids (PFAAs) have also been found in human blood serum and breast milk.5–8 Because of their known and suspected toxic effects,9–11 it is important to understand the pathways of human exposure to minimize the risk for exposure and possible adverse health effects. The European Food Safety Authority therefore established tolerable daily intakes (TDIs) for perfluorooctanoic acid (PFOA), and perfluoroctane sulfonic acid (PFOS) in response to concerns about these chemicals.12 They recently revised these TDIs and established a new and much lower tolerable weekly intake rate of 4.4 ng per kg bw per week for the extended group of PFOA, PFNA, PFHxS and PFOS.13 Food has been identified as the main source of human exposure,14–19 and crops are one possible vector for PFAAs into the food supply. PFAAs are taken up by crops when grown in soil that has been contaminated, for instance via water reuse irrigation or biosolids application,20,21 and there are two known cases where agricultural sites have been widely contaminated with PFAAs in Germany.22,23 The aim of the presented work is to further our understanding of how PFAAs are transferred from soils to crops.
Current knowledge of plant uptake of PFAAs has been summarized in several recent reviews.24–26 In early research on this subject, Stahl et al.20 and Lechner et al.27 showed that the concentration of PFOA and PFOS in the vegetative parts of several crops was linearly proportional to the concentration in the soil they were grown in. Yoo et al. found that the foliage/soil concentration factors of C6–C14 perfluoroalkyl carboxylic acids (PFCAs) in grass decreased logarithmically with increasing chain length, while the foliage/soil pore water concentration factors increased with chain length.28 This evidence for a pronounced influence of soil-pore water partitioning on uptake was supported by Blaine et al., who found that uptake by crops varied between soils.29 In another field experiment Blaine and coworkers studied the distribution of C4–C10 PFAAs in four crops. They found that root/soil concentration factors (RCFS) varied little or not at all with chain length, but in agreement with Yoo et al. they found that both the shoot/soil concentration factors and the fruit/soil concentration factors decreased with increasing chain length, the first by 0.11–0.36
log units per CF2 group, the second by 0.54–0.58
log units. They incorporated these relationships into a simple conceptual model of PFAA uptake, attributing the lower accumulation of longer chained PFAAs in fruit compared to roots and shoot to an increased number of biological barriers that must be crossed (e.g. the cambium during loading into the phloem for transport to the fruit, and the Casparian strip separating root epidermis and cortex from root vascular tissue).30 Wen et al., on the other hand, postulated that transport within the plant was mediated by the sorption of PFAAs to plant tissue and explored correlations between shoot/soil and root/soil concentration factors and the lipid and protein content of the respective tissue.31
Further mechanistic insight into plant uptake of PFAAs can be obtained by studying hydroponic systems without the influence of soil. Transport of organic chemicals from the root zone to foliage is governed by the transpiration stream concentration factor (TSCF, the quotient of the concentration in the xylem flow and that in the nutrient solution) and the quantity of water transpired.32–34 In hydroponic experiments the TSCFs for C4–C11 PFCAs were similar in tomato, cabbage and zucchini and only weakly influenced by chain length, varying by less than a factor of 2.5 within a given species.35 In a hydroponic study with grass, the foliage-solution accumulation factors also varied by less than a factor 2.5 for C4–C10 PFAAs.36 This suggests that the combined effects of biological barriers hindering PFAA uptake into the root and sorption to root vascular tissue retarding transport to foliage are similar across chain lengths and between species. Lettuce, on the other hand, showed a decidedly different, V-shaped, chain length dependency, with TSCF values for perfluorododecanoic acid (PFDoDA) that were 6 times greater than for perfluoroheptanoic acid (PFHpA) suggesting the presence of a chain length specific barrier to root uptake.37 Salinity and temperature were shown to be positively correlated with PFAA uptake rate, whereby this was attributed to the influence of these variables on transpiration rate.38 Concerning accumulation in roots, root uptake factors from soil calculated on the basis of concentrations in pore water were up to two orders of magnitude lower than root uptake factors for the same crop (lettuce) in a hydroponic exposure. This was attributed to lower sorption to the roots surface as a result of competition from other molecules in the pore water and indicates that hydroponic experiments are of limited value for studying accumulation in roots.39 It has also been suggested that differences in root development between hydroponic and soil growing conditions lead to differences in PFAA accumulation.40
Despite this wealth of research, there is still limited understanding of the dominant factors controlling uptake of PFAAs from soil into edible plant parts under real-world exposure conditions. To contribute to progressing knowledge in the area, we grew four crops in outdoor lysimeters containing soil spiked with PFAAs. Radish, lettuce, pea and maize were chosen because their edible parts come from different parts of the plant (roots vs. leaves vs. pulses vs. cereal grain). A broad range of PFAAs was studied: 11 PFCAs (C4–C14) and 2 perfluoroalkane sulfonates (C4 and C8). Five lysimeters were used for each crop, four of which were spiked at different PFAA levels plus one non-spiked control. The different plant parts (roots, stems, leaves, etc.) were analyzed separately. Uptake factors based on PFAA concentrations in soil and pore water were calculated and compared across chain length and species.
Materials and methods
Chemical reagents and lab materials
The names, abbreviations and molecular formulas of the test chemicals, their suppliers and purities, and the 13C-labeled internal standards used for their quantification can be found in Tables S1 and S2 of the ESI.† All standards had a purity >95%.
Materials used for extraction and clean-up of the samples included Florisil SPE cartridges (1000 mg, 6 mL) from Applied Separations (Allentown, PA, USA); Acrodisc LC13 GHP Pall 0.2 μm filters from Pall Corporation (Port Washington, NY, USA); 50 and 15 mL polypropylene (PP) tubes with screw caps from Sarstedt (Nümbrecht, Germany); and Supelclean ENVI-Carb 120/140 from Supelco (Bellefonte, PA, USA). Tetrabutylammoniumhydrogensulfate and sodium hydrogencarbonate were purchased from Merck (Darmstadt, Germany). Sodium carbonate and ammonium hydroxide ACS reagent were from Sigma Aldrich; 2.0 and 0.3 mL PP vials were purchased from VWR International (Amsterdam, Netherlands). Centrifugation filter tubes (50 mL, 0.2 μm nylon filter) were obtained from Grace (Breda, Netherlands). For solvents see Table S1.†
Field experiment
The field experiment was conducted at the Fraunhofer Institute for Molecular Biology and Applied Ecology in Schmallenberg, Germany (51.15N, 8.29E) between June and October 2011. Each plant species was grown in 5 lysimeters, one containing soil with background concentrations of PFAAs (non-spiked), and 4 spiked with a mixture of PFAAs in which each PFAA had a nominal concentration in soil of 0.1 mg per kg dw, 1 mg per kg dw, 5 mg per kg dw and 10 mg per kg dw, respectively. For comparison, the PFOA and PFOS concentrations measured in contaminated agricultural soil in Arnsberg (51.41N, 8.05E), ∼30 km from Schmallenberg, were ∼1 mg per kg dw.22 The lysimeters had a surface area of 1 m2 and a total depth of 60 cm and were each filled with ∼450 kg sand (30–60 cm depth) and ∼450 kg of loamy sand (0–30 cm depth; 71% sand, 24% silt, 5% clay, pH 5.67, organic carbon content 0.93%). This resembled a typical soil in northwestern Germany. The soil used for the upper layer is available as a reference soil (Refesol 01-A) from Fraunhofer IME (www.refesol.de/boden01a.shtml). The lysimeters were outdoors and unprotected (see Fig. S1†). Precipitation was measured with a rain gauge located close to the lysimeters (Table S3†).
The spiking of the soil was described in a previous paper.41 Briefly, a stock solution was prepared containing equal concentrations of all PFAAs. This stock solution was spiked into 2 kg of soil which was homogenized and then mixed with approx. 90 kg of soil in a concrete mixer to achieve the desired concentration. This was repeated 5 times for each layer in each lysimeter. Samples were taken from each batch and combined to determine the final PFAA concentration in the soil of each lysimeter.
The lysimeters were planted with onion, carrot, radish, lettuce, pea, bean or maize (Table 1). Each crop was planted in one lysimeter of each spiked soil level. Onion, carrot and radish were planted together as were pea and bean, while lettuce and maize were the only crops in their respective lysimeters. Within one week of preparing the spiked soil, 6 bean seeds, 20 radish seeds, 20 onion seeds, 20 carrot seeds and 6 pea seeds plus 9 maize seedlings and 20 lettuce seedlings (pre-grown in uncontaminated soil) were planted in the respective lysimeters (on June 21, 2011). The lysimeters were watered after planting, and kept humid by natural precipitation and additional watering if needed (see Table S3† for water inputs).
Table 1 Summary of the field experiment
Crop |
Soil concentration (nominal, mg kg−1) |
Plant compartments sampled |
B = the background contamination present in the test soil.
The results for lettuce have already been published in a comparison of PFAA uptake under hydroponic and soil growing conditions.39
|
Onion (Allium cepa) |
Ba, 0.1, 1, 5, 10 |
Did not germinate |
Carrot (Daucus carota) |
Ba, 0.1, 1, 5, 10 |
Did not germinate |
Radish (Rapahnus sativus) |
Ba, 0.1, 1, 5, 10 |
Roots, bulb, foliage |
Lettuceb (Lactuca sativa) |
Ba, 0.1, 1, 5, 10 |
Roots, foliage |
Pea (Pisum sativum) |
Ba, 0.1, 1, 5, 10 |
Roots, stem, twigs, leaves, pods, peas |
Bean (Phaseolus vulgaris) |
Ba, 0.1, 1, 5, 10 |
Did not germinate |
Maize (Zea mays) |
Ba, 0.1, 1, 5, 10 |
Roots, stem, leaves, hull leaves, cobs, kernels |
Onion, carrot and bean did not germinate in either the exposed or the control lysimeters. The other plants were harvested at maturity (see schedule in Table S4†). At harvest soil samples were also taken. The soil samples were collected with a corer for lettuce, pea and maize. The soil core was separated into the upper (30 cm loamy sand) and lower (30 cm sand) layers, and the soil was packed in freezer bags and stored at −20 °C until analysis. It was not possible to sample the lower soil layer in some of the lysimeters. Consequently, only the results for the upper soil layer are presented here. For radish only the top 1–2 cm of the soil were sampled, because at the time of radish harvest it was still hoped that the onions and carrots seeded in the same lysimeters would germinate. The whole plant was harvested and divided into plant parts as detailed in Table 1. All plant parts were packed in polyethylene freezer bags and stored at −20 °C until analysis.
Extraction
Root and radish bulb samples were gently washed (no brushes were used) under running demineralized water to remove adherent soil and afterwards dried superficially by patting with kitchen towels. No cleaning of the other plant samples was necessary. The material was homogenized with a household blender (Braun Multiquick MX 2050). For the extraction of the PFAAs from the samples, the method by Hansen et al.42 was used with modifications proposed by Vestergren et al.,43 as described in our previous work.35,37 Briefly, 10 g of the homogenate were weighed in a 50 mL PP tube, spiked with isotope-labeled surrogate standards and mixed with 5 mL of 0.4 M NaOH solution. The samples were then left in the fridge over night to allow the internal standards to distribute in the slurry. Next, the samples were mixed with 4 mL of 0.5 M tetrabutylammonium hydrogen sulfate solution and 5 mL of a carbonate buffer (0.25 M Na2CO3/NaHCO3) and extracted with 10 mL methyl tert-butyl ether (MTBE). After centrifugation for 10 minutes at 3000 rpm and room temperature the MTBE phase was transferred to a new 50 mL PP tube and the extraction with MTBE was repeated two times. The extracts were combined and concentrated to approximately 2 mL using a Rapidvap (Labconco Corp., Kansas City, MO, USA). After a clean-up step using Florisil SPE-cartridges to remove non-polar matrix, the final extract was evaporated to 1 mL using a Rapidvap. If the extract was still strongly colored then an additional clean-up step following the Powley method with ENVI-Carb was added.44
For the analysis of PFAAs in soil, the soil was dried in an oven at 40 °C until no further weight loss was recorded. After homogenization, 1 g of soil was weighed in a 15 mL PP tube and spiked with internal standards. The soil was then extracted with 10 mL MeOH by vortex mixing for 1 minute and sonication for 10 minutes. The supernatant after centrifugation (10 min, 3000 rpm) was transferred to a new 15 mL PP tube and concentrated in the Rapidvap. The extraction was repeated twice with 5 mL MeOH. The extracts were combined and concentrated in the Rapidvap to a final volume of 1 mL.
For pore water analysis, 20 g of the soil was put in a 50 mL centrifugation filter tube with a 0.2 μm nylon filter. After 20 minutes of centrifugation at 2000 rpm, 0.5 mL of pore water was transferred to a vial. The internal standards and MeOH were added to achieve a final volume of 1 mL.
All final extracts were passed through an Acrodisc LC 13 GHP Pall nylon filter into 2 mL PP vials and stored at 4 °C until analysis.
Analysis
For PFAA analysis an HPLC system (LC-20AD XR pump, SIL-20A autosampler and SCL-10A VP system controller, Shimadzu, Kyoto, Japan) coupled with a tandem mass spectrometer (4000 QTrap, Applied Biosystems, Toronto, Canada) was used. A pre-column (Pathfinder 300 PS-C18 column, ID 4.6 mm; length 50 mm; 3 μm particle diameter; Shimadzu, Duisburg, Germany) prior to the injection valve was used to remove potential background contamination.
The analytes were separated on an ACE 3 C18-300 column (ID 2.1 mm; length 150 mm; 3 μm particle diameter; Advanced Chromatography Technologies, Aberdeen, Scotland) maintained at 30 °C with a mobile phase gradient consisting of two eluents A (40
:
60 MeOH
:
H2O, v/v) and B (95
:
5 MeOH
:
H2O; v/v), both with 2 mM ammonium acetate. The gradient used for separation and the mass transitions as well as other mass spectrometer settings can be found in our previous papers.35,37,39 The mass spectrometer was equipped with an electrospray ionization interface operating in the negative ionization mode, and it was run in the scheduled MRM-mode.
The purified extracts were diluted 1
:
1 with UPLC grade water prior to analysis to match the injection conditions of the HPLC. As pore water samples already had a water
:
methanol ratio of 1
:
1, no further dilution was performed for these samples. A volume of 20 μL was injected.
Raw data were processed with the Analyst 1.5 software package (Applied Biosystems).
Quality assurance and control
Each sample was extracted in duplicate and each extract was injected in duplicate. The relative standard deviation of the concentrations derived from these four injections was <10% for all analytes in all samples.
Concentrations were quantified using a twelve-point calibration, with R2 > 0.99 for all analytes; no weighting was applied. Further information on quality assurance and quality control is provided in our previous studies.35,37
Recoveries were determined by comparison with a matrix free solution spiked with internal standard immediately prior to injection. Average recoveries of the internal standards in the samples were between 22% (PFBA) and 112% (PFDoDA) (see Table S5†). Despite the low recovery of PFBA, the use of an isotope labeled PFBA surrogate standard provided for satisfactory data quality as evidenced by good method repeatability.
Limits of quantification (LoQs) (Table S6†) were calculated on the basis of the lowest validated calibration standard (signal![[thin space (1/6-em)]](https://www.rsc.org/images/entities/char_2009.gif)
noise ratio ≥10). They were derived from the amount injected back calculated to an extract volume of 1 mL and divided by the average extracted sample quantity. A method blank (beginning with simulated extraction without matrix) was prepared with every batch of samples; these blanks showed no quantifiable contamination. Solvent blanks were injected every ten injections to check for contamination of the LC system and for memory effects, but no contamination or memory effects were observed during the study.
All PFAA concentrations from the non-spiked lysimeters (in plant parts as well as in soil or pore water) were subtracted from the concentrations in the spiked lysimeters. This corrected for any impact of atmospheric deposition or other sources besides soil on PFAA levels in the plants. Resulting concentrations below the LoQ were neglected.
Since PFOS is the only compound for which branched isomers were included in the standards used for the calibration curve, branched isomers could only be quantified for PFOS. All reported PFOS concentrations are sum concentrations of non-branched and branched isomers.
Results and discussion
Radish and lettuce plants grown in the highest exposure level soil were markedly smaller at the time of harvest than those growing in the lower exposure levels, suggesting that the PFAAs had phytotoxic effects (see Fig. S2† and Felizeter et al.39). Results for radish and lettuce from the highest exposure level were therefore not used in the data interpretation. Pea and maize plants showed no visible signs of phytotoxicity.
PFAA fate in soil
After spiking, the concentrations in soil were generally within the intended concentration range (Table S7†). At harvest, concentrations in soil of the shortest chain PFAAs, the C4–C6 PFCAs and PFBS, were almost reduced to background concentrations through the whole soil column (<3% of the initial mass remaining, Table S8†). A large fraction of the short chain PFAAs was recovered in the drainage water, illustrating the transient nature of surface soil contamination with these substances.41 From this perspective, lysimeter studies can provide a more realistic simulation of plant uptake than laboratory hydroponic or pot experiments with constant exposure concentrations. In contrast to the shortest chain PFAAs, 80–90% of the spiked PFDoDA, PFTrDA and PFTeDA was found in the soil at the harvest date. The mid-chain length compounds (PFOA, PFNA and PFOS) generally had the highest concentrations in soil pore water (Table S9†).
The behavior of PFAAs in the lysimeter soil was analyzed in another paper.41 We showed that leaching was the dominant process for the loss of the short-chain PFAAs from the soil. Furthermore, leaching occurred at a faster rate than expected from calculated KD values, and this accelerated leaching was greater when the initial spiked soil concentration level was higher. We attributed the accelerated leaching to interactions between the PFAAs related to competition for sorption sites in the soil. Analysis of the leachate collected from the lysimeters suggested that leaching occurred primarily at the beginning of the experiment. Additionally, lower rainfall towards the end of the experiment resulted in more stable conditions. The evidence indicates that soil concentrations were more stable towards the end of the experiment.
Concentration factors
To evaluate the plant uptake of the PFAAs, concentration factors were calculated for the different plant tissues on the basis of the sampled exposure media, soil and pore water (ng g−1 ww plant tissue/ng g−1 dw soil, or ng g−1 ww plant tissue/ng mL−1 pore water, respectively). Soil and pore water concentrations were only available for the start and the end of the experiment. We used the soil and pore water concentrations at the end of the experiment (i.e. date of harvest) for the calculation of concentration factors because the bulk of transpiration and hence pore water uptake occurred during the latter part of the growth period. Soil concentrations were judged to have been more stable during this period, but it is nevertheless possible that the concentration factors were overestimated for the shorter chained PFAAs for which the concentration in soil decreased over the experiment (see above). As the soil sampled from the radish lysimeters was from the top 1–2 cm only (see above) and not representative for the root zone, the concentration in these samples was not used. The PFAA concentrations in surface soil at harvest were comparable between crops for most PFAAs, except for the concentrations of the shorter chained PFAAs which tended to decrease over time. Since lettuce was the first crop sampled after radish, the concentrations in soil from the lettuce lysimeters were used to evaluate the radish results.
Effect of exposure concentration
The PFAA concentrations in the different tissues for each of the different plants and exposure concentrations are reported in Tables S10–S13.† In the literature, the uptake of PFAAs by plants has generally been observed to be linearly correlated with exposure concentration.20,27,29,35,37,45,46 However, in hydroponic studies we observed lower root concentration factors for C7–C14 PFCAs and PFOS at 10 ng mL−1 compared to lower exposure concentrations.35,37 Although we had higher pore water concentrations in the present study (Table S9†), there was no consistent pattern of lower root concentration factors at higher exposure levels for these PFAAs (Tables S14 and S15†). This indicates that the non-linear root – pore water isotherms observed under hydroponic conditions cannot be extrapolated to soil systems. We therefore averaged the concentration factors from all exposure levels for our evaluation. The geometric mean was used because concentration factors are logarithmically distributed. Concentration factors for each exposure level can be found in Tables S14–S19.† It was not possible to retrieve any pore water from the pea lysimeters at the time of harvest, so concentrations in pore water from the lettuce lysimeters were used for calculating pore water-based concentration factors for pea.
Differences in the log concentration factor were statistically assessed using the t-test (two tailed, two sample unequal variance, significance threshold p < 0.05) in Excel (Microsoft). However, due to the limited number of data points (max. 4 per species and compound) the explanatory power of the statistical analysis is limited.
Accumulation in edible plant parts
Of primary interest for human exposure is accumulation in edible plant parts. Edible plant part concentration factors referenced to soil (ECFS) were calculated for radish bulbs, lettuce leaves, peas and maize kernels (Fig. 1, Table S16†). Very large variability was observed between the different PFAAs, with ECFS ranging by as much as seven orders of magnitude for a given edible part. In addition, there were large differences between different edible parts for a given PFAA, in some cases in excess of three orders of magnitude. Within this great variability there was also evidence of systematic structure. In particular, ECFS tended to decrease with PFAA chain length.
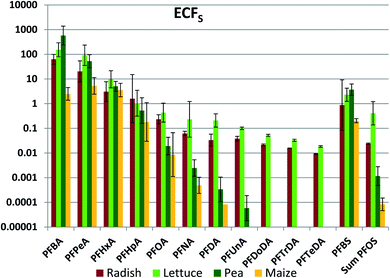 |
| Fig. 1 Edible part concentration factor based on concentration in soil (ECFS, kg soil dry weight per kg edible part fresh weight). The average and standard deviation from experiments conducted at different exposure levels are shown, assuming a log-normal distribution. In cases with no error bars, ECFS could be determined in just one exposure level. | |
In order to explore the factors determining PFAA transfer to edible plant parts, we use a mechanistically-based framework. In this framework, uptake occurs from soil into the root, and this is the rate limiting step for accumulation in the plant. From there the transpiration stream transports the PFAAs through the roots and the stem to the leaves, where they accumulate due to evaporation of the transpired water. Transport into plant fruits originates in the leaves, where PFAAs enter the phloem and flow to fruit tissues. In alignment with this framework, we first examine the PFAA uptake into the whole plant. We then consider what fraction of this uptake is retained in the roots and the stem. Finally, we study the relationship between concentrations in fruits and leaves before assembling a mathematical model to describe the observations.
Whole plant concentration factors
Whole plant concentrations CP were calculated by summing the PFAA quantities in the different plant parts and dividing by the total plant biomass. CP was used to determine whole plant concentration factor referenced to soil (PCFS, Fig. 2, Table S17†). The between species variability in PCFS was low; significant differences between species exceeding a factor two were observed only nine times: PFBA (between lettuce and pea), PFHxA (pea/corn), PFNA (radish/corn), PFDoDA (radish/all others) and PFBS (pea/all others) (Fig. 2). This is a quite remarkable finding. We had expected PCFS to depend on plant variables such as the cumulative amount of water transpired per unit plant biomass as well as species-specific differences in the barrier limiting PFAA transport across the root endodermis into the transpiration stream. Fig. 2 suggests that these factors did not differ greatly between the plants studied here.
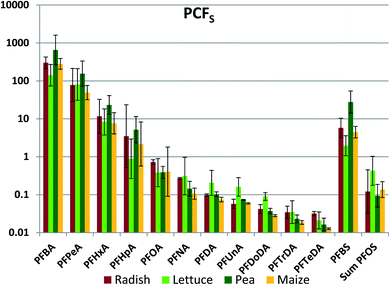 |
| Fig. 2 Whole plant concentration factor based on concentration in soil (PCFS, kg soil dry weight per kg plant fresh weight). The average and standard deviation from experiments conducted at different exposure levels are shown, assuming a log-normal distribution. | |
The inter-chemical variability in PCFS was large, ranging up to 4.5 orders of magnitude, but nevertheless considerably less than the inter-chemical variability in ECFS (Fig. 2). There was a very consistent trend of decreasing PCFS with increasing chain length for the PFCAs. PCFS for the PFSAs corresponded to PCFS for the PFCA with the same or a slightly longer perfluorinated chain; PCFS for PFBS was similar to PFHxA, while PCFS for PFOS was similar to PFNA/PFDA. Increasing chain length corresponds to increasing tendency of the PFAAs to sorb to the soil solids.41 This suggests that the strong inverse relationship between PCFS and chain length may have been a result of PFAA sequestration to soil solids reducing availability for uptake.
The whole plant concentration factor referenced to pore water (PCFPW) eliminates the influence of sorption to soil solids on the bioaccumulation metric. PCFPW shows a much lower inter-chemical variability than PCFS (Fig. 3, Table S18†). PCFPW ranged over 1.5–2 orders of magnitude for a given species compared to 4 orders of magnitude for PCFS. The variability between species was once again small, with significant differences observed for only five pairs: PFNA (lettuce/corn and pea/corn), PFBS (lettuce/pea and pea/corn) and PFOS (pea/corn). There was a pronounced chain length trend in mean PCFPW, with similar PCFPW for short and long chain lengths and decreasing PCFPW towards intermediate chain lengths with a minimum in the vicinity of PFOA. The trends were overlain by pronounced PCFPW variability between the exposure levels, so that there were few significant differences between PFAAs for radish and lettuce. However, for pea and maize the concentrations of PFOA (maize only) and PFOA (pea and maize) were significantly lower than most of the other PFAAs (Table S19†). The trends suggest that the uptake of the intermediate chain length PFAAs across the root endodermis is restricted compared to the other PFAAs. However, PCFPW for the shorter chain PFAA may be overestimated due to the uncertainty in the concentrations in pore water (see above), and PCFPW for the longer chain PFAAs may contain a contribution from sorption to the root surface or contamination of foliage with soil particles (see below). Similar to PCFS, PCFPW for the PFSAs corresponded to PCFPW for the PFCA with a one CF2 unit longer perfluoroalkyl chain; PFBS was similar to PFHxA, while PFOS was similar to PFDA. The strong reduction in variability of PCFPW (Fig. 3) compared to PCFS (Fig. 2) demonstrates the dominant role that sorption to soil plays in regulating plant uptake of PFAAs.
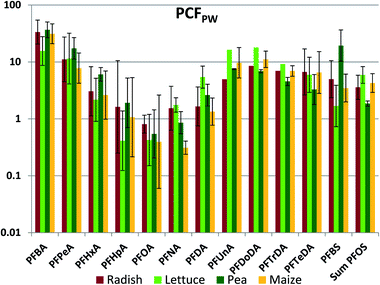 |
| Fig. 3 Whole plant concentration factor based on concentration in pore water (PCFPW, L pore water per kg plant fresh weight). The average and standard deviation from experiments conducted at different exposure levels are shown, assuming a log-normal distribution. | |
One significant departure from the species similarity in PCFPW is seen for PFHpA, which was 4–5 times lower in lettuce than in the other four species. PCFPW was also lower in lettuce for PFHxA, PFOA and PFBS. This is consistent with measured transpiration stream concentration factors, which showed a pronounced V-shaped dependence with chain-length with a minimum at PFHpA that was more pronounced for lettuce than for other plant species.35,37 A second significant departure is seen for PFNA in maize. In maize, the minimum for PCFPW was shifted to the right from PFOA to PFNA.
Retention in roots
Once having entered the roots from the soil, some portion of the chemical will be retained in the roots and some portion may be transported to the aerial plant parts with the transpiration stream. We assessed this using a root retention factor (RRF), defined as the fraction of the chemical mass in the whole plant at harvest that was present in the roots. RRF ranged from 99.5% (PFDoDA in maize) to 1% (PFBA in maize) (Fig. 4 (normal scale) and Fig. S3† (log scale), Table S20†). For a given PFAA the RRF was not significantly different between species for 50 out of 78 sample pairs (Table S21†), indicating that plant specific properties did not have a dominant influence on root retention. No species had an RRF that was greater than another species for all PFAAs. For a given plant species, RRF generally increased with chain length up to nCF = 10, after which it remained approximately constant. This observation is consistent with the increase in sorption tendency of PFAAs with increasing nCF;41 the longer chained PFAA sorb more strongly to root tissue after they are taken up, resulting in a smaller fraction being available for further transport with the transpiration stream. Once again, the RRFs for the PFSAs corresponded to the RRF for the PFCA with the same or a slightly longer perfluorinated chain.
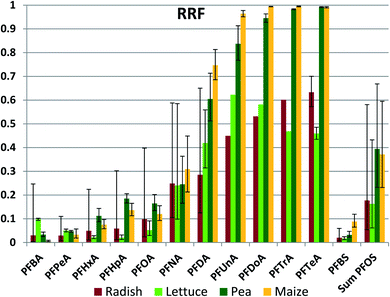 |
| Fig. 4 Root Retention Factor (RRF), equal to the PFAA mass in the roots as a fraction of the PFAA mass in the whole plant. The average and standard deviation from experiments conducted at different exposure levels are shown, assuming a log-normal distribution. There are no error bars when n was ≤2. | |
There are several notable exceptions to these trends. One is that RRF of the longer chained PFAAs (nCF ≥ 10) is consistently lower for radish and lettuce than for pea and maize, in most cases significantly (Fig. 4, Table S21†). The latter two plants have RRFs of 99% for the longest chained PFAAs, while for radish and lettuce it is only 50–60%. Radish and lettuce also have a large fraction of the longest chained PFAAs in the leaves. This may be due to contamination of the leaves with soil particles by processes such as rain splash. A leaf/soil concentration factor of 0.1–0.25 kg dry soil per kg leaf dry weight (roughly equivalent to 0.01–0.025 kg dry soil per kg leaf wet weight) in leaves growing close to the soil has been observed for other organic contaminants that are not taken up appreciably via the roots.47 Hence the lower RRFs for these plants may not reflect a lower root retention, but instead be an artifact arising from contributions of another uptake pathway to plant contamination.
A second exception is the significantly lower RRF for PFHxA, PFHpA, PFOA and PFBS in lettuce compared to pea and maize. As noted above, PCFPW of these substances was also lower in lettuce compared to the other species, presumably due to more restricted uptake across the root endodermis. However, we could identify no mechanistic link that would explain a positive relationship between uptake efficiency across the root epidermis and retention in root tissue. At this time we can offer no explanation for the weaker retention of these particular PFAAs in lettuce roots.
PFBA builds a third exception to the general trends in RRF. The differences in RRF between species exceed an order of magnitude. This may be due to the temporal variability in PFBA exposure. Due to its low KD, PFBA was rapidly leached out of the soil. In addition, concentrations in pore water would be expected to increase as soil dries out since PFBA in pore water is not buffered by a sorbed fraction. Levels in roots will tend to reflect more recent exposure while levels in foliage represent cumulative exposure, so consequently a dynamic exposure situation can result in changing RRF values over time.
Retention in stems
For pea and corn it was also possible to evaluate retention in stems. A stem retention factor (SRF) was calculated as the fraction of the PFAA mass in the above-ground plant parts that was present in the stem. Like the RRF, SRF increased with PFAA chain length, reflecting the increasing tendency of the PFAAs to sorb to plant tissue with increasing chain length (Fig. S4†). For the shorter chain PFAAs (nCF ≤7) there was little variation in SRF with chain length and there was a marked difference between the two species (∼0.15 in pea and 0.05 in maize). However, the stem played a minor role in the storage of these PFAAs in pea and maize.
Distribution between leaves and edible plant parts
The bulk of the PFAAs in aerial plant parts was stored in the leaves (see Fig. S5†). This was expected, as PFAAs would be expected to accumulate at the location where water is lost from the plant. We anticipate that the leaves are the primary source of PFAAs in fruits because the molecular building blocks for the fruit are synthesized largely in leaves and then transported via the phloem to the fruit.48 Edible plant part/leaf concentration factors (ELCFs) were calculated as the quotient of PFAA concentration in the edible plant part (radish bulbs, peas and maize kernels) and in the respective leaf or foliage sample (Fig. 5, Table S22†). ELCFs for pea and maize were similar, with only PFBA and PFBS being significantly different (lower for maize). For these crops the ELCF generally decreased with increasing nCF. Interestingly, the plant parts enclosing peas and maize displayed a different accumulation pattern. The maize husk/leaf and maize cob/leaf concentration factors were relatively independent of nCF, with log values of −1.47 ± 0.16 and −1.96 ± 0.32, respectively, and pea pod/leaf concentration factors were independent of nCF for nCF ≥ 6 (Fig. S6 and S7†). Concentration factors calculated from PFAA measurements in corn leaves, husks, cobs and kernels made by Liu et al. were similar to our results with the exception of PFOS, which shows a unique behavior in their study (Fig. S8†). Liu et al. also studied soybean, and the pod/leaf and soybean/leaf concentration factors in their study were similar to our observations for pea pods and peas (Fig. S9†).49 Blaine et al. measured PFAAs in pea shoots (stem + leaves) and fruit, and the derived pea pod/leaf concentration factors agreed quite well with ours (Fig. S9†).30
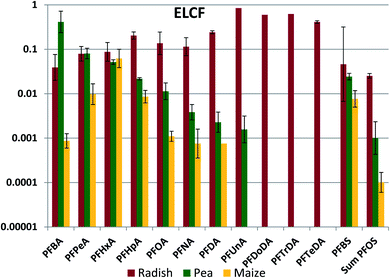 |
| Fig. 5 Edible part to leaf concentration factor (ELCF, kg leaf fresh weight per kg edible part fresh weight). The average and standard deviation from experiments conducted at different exposure levels are shown, assuming a log-normal distribution. | |
The lack of chain length dependence of the concentration factors for husks, cobs and pods suggest that the primary source of PFAAs to these tissues may be the same as the primary source to the leaves, namely the transpiration stream. The low values of the concentration factors (generally 0.01–0.04) could suggest much lower cumulative transpiration flow to these tissues than to the leaves. The pronounced chain length dependence for the peas, beans and kernels suggest that they have a different primary PFAA source. The chain length differentiation may arise during loading of the phloem. If there is no barrier preventing equilibration of PFAAs between the phloem contents and the freely dissolved fraction in the plant part where the phloem is loaded, then the phloem contents would contain a PFAA pattern that represents the freely dissolved fraction in the plant part, modified by any sorption occurring to solids in the phloem contents. If the sorption capacity of the phloem contents is lower than for the leaf as a whole, then the longer chained PFAAs will be more weakly represented in the phloem.
A pronounced chain length dependence of fruit/leaf concentration factors has been observed for other plants. For instance, we measured a log–linear relationship between the tomato fruit/leaf concentration factor and chain length in a hydroponic study, the concentration factor decreasing by three orders of magnitude from PFPeA to PFUnA, and similar trends were observed for tomato in two soil-based studies (see Fig. S10†).30,35,50 However, not all crops show this pronounced chain length selection in PFAA transfer to edible plant parts. Little chain length dependence was observed in wheat grain/leaf (straw) concentration factors,51 suggesting that other phenomena are governing transfer of PFAAs to grains.
In contrast to the other edible plant parts, the ELCF for radish showed no pronounced chain length dependence, varying around 0.1 for nCF of 3–8 (Fig. 5). Similar values of ELCF were calculated from the measurements of Blaine et al.30 and Liu et al.,49 with no chain length dependence for nCF of 3–8 (Fig. S11†). Radish bulbs are in between the roots and the foliage of the radish plant, so it is likely that PFAAs also reached the bulbs via the transpiration stream (xylem) from the roots. The fraction of the total plant residue that was present in the bulb was similar for the different PFAAs, ranging between 0.16 and 0.40 with the exception of PFBA (0.11) and PFOS (0.06).
A quantitative model of PFAA uptake into crops
The experimental results assembled here, with concentrations of 13 PFAAs in different tissues of four crop species exposed at four different levels, provided a large, internally consistent data set with which to explore the influence of different parameters on PFAA uptake in plants. We used this data to develop a simple quantitative empirical model of PFAA accumulation in roots, leaves, fruits and radish bulbs, taking into consideration the limitations in the data arising from the experimental design and information available in the literature.
Uptake in the whole plant.
PFAA uptake into the plant is treated as the sum or two processes, uptake from soil via the roots and uptake from soil directly to foliage. The former is estimated using a function of PCFPWversus nCF based on Fig. 3, with constant PCFPW for shorter and longer chain lengths and a V-shaped minimum centered at nCF = 7 (Table 2). Here and in the remainder of the model, an extra unit is added to nCF of the PFSAs to account for their stronger sorption compared to the PFCA of equivalent nCF. Uptake from soil directly to foliage is estimated using a soil-to-leaf concentration factor (SLCF) of 0.02 kg dry soil per kg leaf wet weight and applied only to plants growing close to the soil surface (here radish and lettuce).where NP is the quantity of PFAA in the plant (mol), NPR is the quantity of PFAA in the plant due to uptake via roots (mol), NPL is the quantity of PFAA in the plant due to uptake via leaves (mol), mP is the mass of the plant (kg wet weight), mL is the mass of the leaves (kg wet weight), CPW is the PFAA concentration in pore water (mol L−1) and CS in the PFAA concentration in soil (mol kg−1 dry soil).
Table 2 Whole plant/pore water concentration factors PCFPW (L kg−1 wet weight) as a function of perfluorinated chain length nCF used in the model. When modeling PFSAs, one unit was added to nCF
n
CF
|
PCFPW |
3 |
6 |
4 |
6 |
5 |
4.1 |
6 |
2.7 |
7 |
0.9 |
8 |
1.6 |
9 |
2 |
10 |
6 |
11 |
6 |
12 |
6 |
13 |
6 |
Concentration in roots.
The PFAA concentration in roots CR (mol kg−1 wet weight) is calculated according to: |  | (4) |
where mR is the mass of the roots (kg wet weight) and RRF is defined by: | log RRF = 0.21nCF − 2.24 (maxRRF = 1) | (5) |
where this equation was determined from the data in Fig. S3† neglecting PFBA in all species and PFHxA, PFHpA and PFOA in lettuce.
Concentration in leaves.
The PFAA concentration in leaves CL (mol kg−1 wet weight) is calculated according to: | 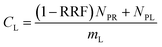 | (6) |
where mL is the mass of the leaf (kg wet weight). This approach assumes that all of the residues not retained in the roots are retained in the leaves. This is clearly a simplification, but most of the residues were retained in the leaves for almost all substances (Fig. S4†). Hence, we judge this to be a reasonable approach to estimate CL. Furthermore, the model cannot predict CL for nCF > 10 since RRF = 1. However, the concentrations of these long-chained PFAAs in leaves are very low and unlikely to be of relevance for exposure assessment.
Concentration in fruit.
The PFAA concentration in fruit CF (mol kg−1 wet weight) is calculated according to:where FLCF, the fruit/leaf concentration factor (kg wet weight per kg wet weight), is defined using a relationship derived from Fig. 5: | log FLCF = −0.38nCF + 0.33 | (8) |
Concentrations in bulbs.
The PFAA concentration in bulbs CB (mol kg−1 wet weight) was derived from the radish bulb data and is calculated according to: |  | (9) |
where mB is the mass of the bulb (kg wet weight). This model assumes that 24% of the PFAA taken up from the soil is stored in the bulb.
Model evaluation.
The ability of this simple model to describe the experimental observations was tested by calculating CR, CL, CF and CB from measured CPW and CS using the above equations and comparing the modeled values with the measured values for each PFAA at each exposure level in each species. Good agreement was obtained, with most of the modeled concentrations lying within a factor 3 (CL and CB) or factor 4 (CR and CF) of the measured concentrations (Fig. 6). The simple model explains a very large portion of the variability in plant/soil concentration factors, which covered up to 7 orders of magnitude (Fig. 1). This indicates that the basic structure of the model, uptake into the roots followed by transport via the transpiration stream and accumulation in the leaves, captures the central features of PFAA behavior. Furthermore, the processes described in the model give an indication of the key factors controlling PFAA transfer from soil to plant parts. These are: (i) decreasing bioavailability in soil with increasing chain length (captured by using the concentration in pore water instead of soil as the driver for uptake); (ii) increasing retention of PFAAs in the roots (and hence decreasing transfer to the leaves) with increasing chain length (eqn (5)); (iii) decreasing transfer from leaves to fruits with increasing chain length (eqn (8)). Each of these three processes is governed by the sorption properties of the PFAAs. Since they act sequentially on the transfer of PFAAs from soil to fruit, there is a multiplicative effect that leads to the very strong influence of chain length on ECFS seen in Fig. 1.
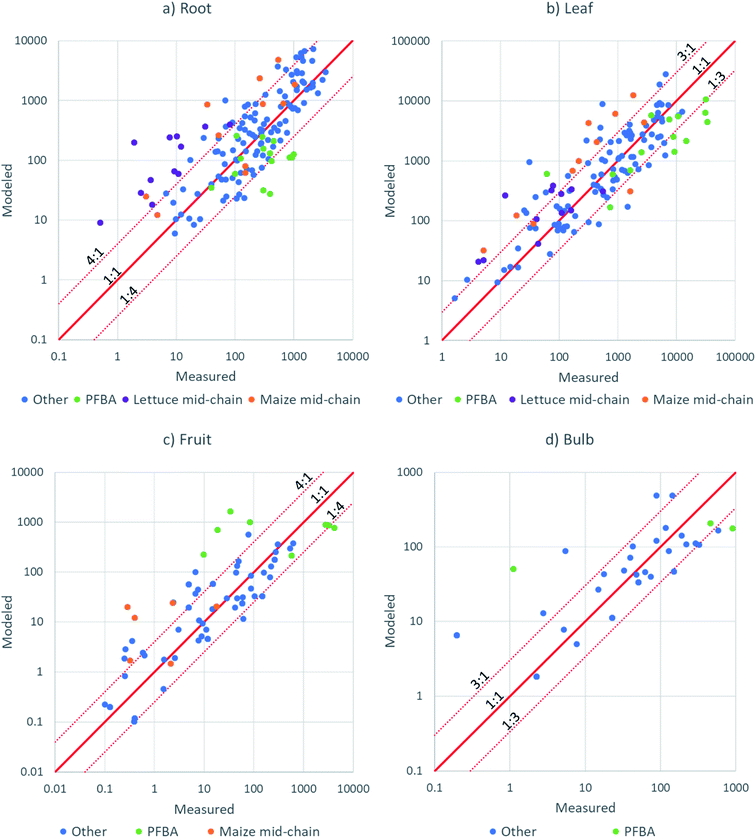 |
| Fig. 6
X–Y plots of modeled versus measured PFAA concentration in: (a) roots; (b) leaves; (c) fruit; (d) radish bulb. | |
An independent evaluation of fruit–leaf model (eqn (8)) was performed by forecasting the concentrations in tomatoes and soybeans from concentration in tomato and soybean leaves using data from studies in the literature.35,49 The agreement was better than a factor 4 for most of the 33 data points (Fig. S11†), but still poorer than the agreements for fruits from this study which were modeled from the PFAA concentration in pore water (Fig. 6c). We recall that there was no chain length dependence in wheat grain/leaf transfer factors51 and hence the model cannot be expected to perform well for all fruits. Measurement of the leaf to fruit transfer of PFAAs for a broader spectrum of plant species is required.
The nature of the data points showing poor model-measurement agreement provides insight into which features of PFAA accumulation behavior are not captured well by the model. A considerable number of the poorly fitting data were for PFHxA, PFHpA, PFOA or PFBS in lettuce or for PFOA, PFNA or PFDA in maize (Fig. 6). In these cases, the poor agreement can be attributed to the shift in the minimum for PCFPW from PFOA to PFHpA in lettuce and from PFOA to PFNA in maize (Fig. 3). For lettuce the deviation is amplified by lower RRF for the same substances (Fig. 4). In our model we have assumed that the dip in PCFPW for mid-chain length PFAAs is the same for all species. This is clearly an oversimplification. Better understanding of this dip in PCFPW and its variability between species is one key for improving our ability to describe PFAA accumulation in plants.
PFBA also generally showed poorer agreement than the other PFAAs (Fig. 6). This may be due to the particularly high uncertainty in exposure for this substance. Most of the other data points outside of the dotted lines were not grouped according to chemical or species, but rather reflected the experimental variability. We believe that the major source of experimental variability was the uncertainty in the concentrations in pore water during the growth period. In future work, more effort should be devoted to measuring the exposure over time.
Perspectives
In general, we found the highest concentrations in the leaves and the roots of the plants and the lowest in the fruit, regardless of the species. Hence leafy and root vegetables pose the highest risk for dietary exposure followed by fruit-bearing crops. This was also concluded by other studies, e.g. Blaine et al.30 The concentrations in the leaves depend on the concentrations in the pore water, which reflect the bioavailable fraction in the soil.
When confronted with an agricultural soil with elevated PFAA concentrations, the type of crop posing the greatest risk for exposure is dependent on which PFAAs are present in the soil. If long chained PFAAs are present, root vegetables like potatoes, carrots or radishes or crops for which the edible part can be in direct contact with the soil pose the highest risk for exposure, while the fruits of fruit-bearing crops will remain largely unaffected. If only short chain PFAAs are present in the soil then the differences between crops are smaller (see Fig. 1) and it may be difficult to identify a crop that will have lower concentrations in the edible part. In this case crops that are used for animal feed may be preferential, as a significant portion of the PFAA will be removed from the human food chain due to incomplete absorption or metabolism in the livestock.52
The chain length dependence of soil to crop transfer is particularly relevant in the context of recent and ongoing changes in PFAA production. Manufacturers switched their production from C8-compounds towards shorter chain compounds53 due to phase-out actions driven by adverse health effects and bioaccumulation in humans and wildlife.54,55 From a bioaccumulation point of view this change is questionable as ECFS values are much higher for C4 than for C8 PFAAs (up to 30
000 times higher for pea, see Fig. 1). The shorter residence time of short chain PFAAs in soil means that crops will not be impacted for as long a period of time. However, the higher mobility of the short chain PFAAs in soil will lead to groundwater becoming contaminated faster and with higher concentrations. Farmers also often use groundwater from their own fields for irrigation. In such cases a contamination cycle will result, prolonging the contamination of the respective fields.
To address concerns arising from the presence of PFAAs in agricultural soil, it is first necessary to determine the PFAA concentrations in porewater. The concentrations in crops can then be predicted using models. The simple models presented here are a first step in this direction, but more sophisticated models should be developed. More extensive empirical observations from controlled field studies are required to this end, whereby particular care needs to be paid to accounting for variable exposure concentrations of more mobile PFAS. Finally, while this study has shown the strong influence of chain length on PFAA behavior, many replacement PFAS are not PFCAs or PFSAs but instead contain other structural modifications that may also influence their fate in soil and uptake from soil into edible plant parts.
Funding
This study was part of the EU project PERFOOD (KBBE-227525), and the financial support of the European Union is gratefully acknowledged.
Conflicts of interest
There are no conflicts of interest to declare.
Acknowledgements
We thank the staff of Fraunhofer IME for their help setting up the field experiments and for taking care of the plants during the growth phase. We thank three anonymous reviewers for helpful suggestions.
References
-
C. Eschauzier, P. de Voogt, H.-J. Brauch and F. Lange, in Polyfluorinated Chemicals and Transformation Products, ed. T. P. Knepper and F. T. Lange, Springer, Berlin/Heidelberg, 2012, vol. 17, pp. 73–102 Search PubMed.
- L. Ahrens, Polyfluoroalkyl compounds in the aquatic environment: a review of their occurrence and fate, J. Environ. Monit., 2011, 13, 20–31 RSC.
- J. P. Giesy and K. Kannan, Global distribution of perfluorooctane sulfonate in wildlife, Environ. Sci. Technol., 2001, 35, 1339–1342 CrossRef CAS PubMed.
- A. Dreyer, I. Weinberg, C. Temme and R. Ebinghaus, Polyfluorinated Compounds in the Atmosphere of the Atlantic and Southern Oceans: Evidence for a Global Distribution, Environ. Sci. Technol., 2009, 43, 6507–6514 CrossRef CAS PubMed.
- A. Barbarossa, R. Masetti, T. Gazzotti, D. Zama, A. Astolfi, B. Veyrand, A. Pession and G. Pagliuca, Perfluoroalkyl substances in human milk: a first survey in Italy, Environ. Int., 2013, 51, 27–30 CrossRef CAS PubMed.
- A. Karrman, J. L. Domingo, X. Llebaria, M. Nadal, E. Bigas, B. van Bavel and G. Lindstrom, Biomonitoring perfluorinated compounds in Catalonia, Spain: concentrations and trends in human liver and milk samples, Environ. Sci. Pollut. Res., 2010, 17, 750–758 CrossRef.
- J.-P. Antignac, B. Veyrand, H. Kadar, P. Marchand, A. Oleko, B. Le Bizec and S. Vandentorren, Occurrence of perfluorinated alkylated substances in breast milk of French women and relation with socio-demographical and clinical parameters: results of the ELFE pilot study, Chemosphere, 2013, 91, 802–808 CrossRef CAS PubMed.
- W. Volkel, O. Genzel-Boroviczeny, H. Demmelmair, C. Gebauer, B. Koletzko, D. Twardella, U. Raab and H. Fromme, Perfluorooctane sulphonate (PFOS) and perfluorooctanoic acid (PFOA) in human breast milk: results of a pilot study, Int. J. Hyg. Environ. Health, 2008, 211, 440–446 CrossRef CAS.
- C. Lau, K. Anitole, C. Hodes, D. Lai, A. Pfahles-Hutchens and J. Seed, Perfluoroalkyl acids: a review of monitoring and toxicological findings, Toxicol. Sci., 2007, 99, 366–394 CrossRef CAS.
- S. Saikat, I. Kreis, B. Davies, S. Bridgman and R. Kamanyire, The impact of PFOS on health in the general population: a review, Environ. Sci.: Processes Impacts, 2013, 15, 329–335 RSC.
- J. L. Domingo, Health risks of dietary exposure to perfluorinated compounds, Environ. Int., 2012, 40, 187–195 CrossRef CAS PubMed.
- N. Johansson, D. Benford, A. Carere, r. J. De Boe, E. Dellatte, P. De Voogt, A. Di Domenico, C. W. Heppner, G. Schoeters and D. Schrenk, EFSA's risk assessment on PFOS and PFOA in the food, Toxicol. Lett., 2009, 189, S42 CrossRef.
- EFSA, Risk to human health related to the presence of perfluoroalkyl substances in food, EFSA J., 2020, 18, 6223 Search PubMed.
- W. D'Hollander, P. de Voogt, W. De Coen and L. Bervoets, Perfluorinated Substances in Human Food and Other Sources of Human Exposure, Rev. Environ. Contam. Toxicol., 2010, 208, 179–215 CrossRef PubMed.
- I. Ericson, R. Marti-Cid, M. Nadal, B. Van Bavel, G. Lindstrom and J. L. Domingo, Human exposure to perfluorinated chemicals through the diet: intake of perfluorinated compounds in foods from the Catalan (Spain) Market, J. Agric. Food Chem., 2008, 56, 1787–1794 CrossRef CAS PubMed.
- E. Dellatte, G. Brambilla, S. P. De Filippis, A. di Domenico, J. Pulkrabova, C. Eschauzier, S. Klenow, G. Heinemeyer and P. de Voogt, Occurrence of selected perfluorinated alkyl acids in lunch meals served at school canteens in Italy and their relevance for children's intake, Food Addit. Contam., Part A, 2013, 30, 1590–1597 CrossRef CAS PubMed.
- H. Fromme, S. A. Tittlemier, W. Volkel, M. Wilhelm and D. Twardella, Perfluorinated compounds – exposure assessment for the general population in western countries, Int. J. Hyg. Environ. Health, 2009, 212, 239–270 CrossRef CAS PubMed.
- D. Herzke, S. Huber, L. Bervoets, W. D'Hollander, J. Hajslova, J. Pulkrabova, G. Brambilla, S. Filippis, S. Klenow, G. Heinemeyer and P. Voogt, Perfluorinated alkylated substances in vegetables collected in four European countries; occurrence and human exposure estimations, Environ. Sci. Pollut. Res., 2013, 1–10, DOI:10.1007/s11356-013-1777-8.
- J. B. Brown, J. M. Conder, J. A. Arblaster and C. P. Higgins, Assessing human health risks from per- and polyfluoroalkyl substance (PFAS)-impacted vegetable consumption: a tiered modeling approach, Environ. Sci. Technol., 2020, 54, 15202–15214 CrossRef CAS PubMed.
- T. Stahl, J. Heyn, H. Thiele, J. Huther, K. Failing, S. Georgii and H. Brunn, Carryover of Perfluorooctanoic Acid (PFOA) and Perfluorooctane Sulfonate (PFOS) from Soil to Plants, Arch. Environ. Contam. Toxicol., 2009, 57, 289–298 CrossRef CAS PubMed.
- H. Zhao, Y. Guan, G. Zhang, Z. Zhang, F. Tan, X. Quan and J. P. Chen, Uptake of perfluorooctane sulfonate (PFOS) by wheat (Triticum aestivum L.) plant, Chemosphere, 2013, 91, 139–144 CrossRef CAS PubMed.
- M. Wilhelm, M. Kraft, K. Rauchfuss and J. Holzer, Assessment and management of the first German case of a contamination with perfluorinated compounds (PFC) in the region Sauerland, North Rhine-Westphalia, J. Toxicol. Environ. Health, Part A, 2008, 71, 725–733 CrossRef CAS PubMed.
-
Regierungspräsidium Karsruhe, Stabsstelle PFC, PFC-Problematik: Zwischenbilanz und Ausblick, 2018, https://rp.baden-wuerttemberg.de/rpk/Abt5/Ref541/PFC/Documents/pfc_buergerinfo_1808.pdf Search PubMed.
- R. Ghisi, T. Vamerali and S. Manzetti, Accumulation of perfluorinated alkyl substances (PFAS) in agricultural plants: a review, Environ. Res., 2019, 169, 326–341 CrossRef CAS PubMed.
- X. Jiao, Q. Shi and J. Gan, Uptake, accumulation and metabolism of PFASs in plants and health perspectives: a critical review, Crit. Rev. Environ. Sci. Technol., 2020 DOI:10.1080/10643389.2020.1809219.
- W. Wang, G. Rhodes, J. Ge, X. Yu and H. Li, Uptake and accumulation of per- and polyfluoroalkyl substances in plants, Chemosphere, 2020, 261, 127584 CrossRef CAS PubMed.
- M. Lechner and H. Knapp, Carryover of Perfluorooctanoic Acid (PFOA) and Perfluorooctane Sulfonate (PFOS) from Soil to Plant and Distribution to the Different Plant Compartments Studied in Cultures of Carrots (Daucus carota ssp. Sativus), Potatoes (Solanum tuberosum), and Cucumbers (Cucumis sativus), J. Agric. Food Chem., 2011, 59, 11011–11018 CrossRef CAS PubMed.
- H. Yoo, J. W. Washington, T. M. Jenkins and J. J. Ellington, Quantitative Determination of Perfluorochemicals and Fluorotelomer Alcohols in Plants from Biosolid-Amended Fields using LC/MS/MS and GC/MS, Environ. Sci. Technol., 2011, 45, 7985–7990 CrossRef CAS PubMed.
- A. C. Blaine, C. D. Rich, L. S. Hundal, C. Lau, M. A. Mills, K. M. Harris and C. P. Higgins, Uptake of Perfluoroalkyl Acids into Edible Crops via Land Applied Biosolids: Field and Greenhouse Studies, Environ. Sci. Technol., 2013, 47, 14062–14069 CrossRef CAS PubMed.
- A. C. Blaine, C. D. Rich, E. M. Sedlacko, L. S. Hundal, K. Kumar, C. Lau, M. A. Mills, K. M. Harris and C. P. Higgins, Perfluoroalkyl Acid Distribution in Various Plant Compartments of Edible Crops Grown in Biosolids-Amended soils, Environ. Sci. Technol., 2014, 48, 7858–7865 CrossRef CAS PubMed.
- B. Wen, Y. Wu, H. Zhang, Y. Liu, X. Hu, H. Huang and S. Zhang, The roles of protein and lipid in the accumulation and distribution of perfluorooctane sulfonate (PFOS) and perfluorooctanoate (PFOA) in plants grown in biosolids-amended soils, Environ. Pollut., 2016, 216, 682–688 CrossRef CAS PubMed.
- L. K. Dodgen, A. Ueda, X. Wu, D. R. Parker and J. Gan, Effect of transpiration on plant accumulation and translocation of PPCP/EDCs, Environ. Pollut., 2015, 198, 144–153 CrossRef CAS PubMed.
- D. Q. Zhang, M. Wang, Q. He, X. Niu and Y. Liang, Distribution of perfluoroalkyl substances (PFASs) in aquatic plant-based systems: from soil adsorption and plant uptake to effects on microbial community, Environ. Pollut., 2020, 257, 113575 CrossRef CAS PubMed.
- G. G. Briggs, R. H. Bromilow and A. A. Evans, Relationships between lipophilicity and root uptake and translocation of non-ionized chemicals by barley, Pestic. Sci., 1982, 13, 495–504 CrossRef CAS.
- S. Felizeter, M. S. McLachlan and P. De Voogt, Root uptake and translocation of perfluorinated alkyl acids by three hydroponically grown crops, J. Agric. Food Chem., 2014, 62, 3334–3342 CrossRef CAS PubMed.
- A. I. Valcárcel, E. Molero, M. C. Escorial, M. C. Chueca and J. L. Tadeo, Uptake of perfluorinated compounds by plants grown in nutrient solution, Sci. Total Environ., 2014, 472, 20–26 CrossRef PubMed.
- S. Felizeter, M. S. McLachlan and P. de Voogt, Uptake of Perfluorinated Alkyl Acids by Hydroponically Grown Lettuce (Lactuca sativa), Environ. Sci. Technol., 2012, 46, 11735–11743 CrossRef CAS PubMed.
- H. Zhao, B. Qu, Y. Guan, J. Jiang and X. Chen, Influence of salinity and temperature on uptake of perfluorinated carboxylic acids (PFCAs) by hydroponically grown wheat (Triticum aestivum L.), SpringerPlus, 2016, 5, 541 CrossRef PubMed.
- S. Felizeter, H. Jürling, M. Kotthoff, P. De Voogt and M. S. McLachlan, Influence of soil on the uptake of perfluorinated alkyl acids by lettuce: a comparison between a hydroponic and a field study, Chemosphere, 2020, 260, 127608 CrossRef CAS PubMed.
- A. Gredelj, C. Nicoletto, S. Polesello, C. Ferrario, S. Valsecchi, R. Lava, A. Barausse, F. Zanon, L. Palmeri, L. Guidolin and M. Bonato, Uptake and translocation of perfluoroalkyl acids (PFAAs) in hydroponically grown red chicory (Cichorium intybus L.): growth and developmental toxicity, comparison with growth in soil and bioavailability implications, Sci. Total Environ., 2020, 720, 137333 CrossRef CAS PubMed.
- M. S. McLachlan, S. Felizeter, M. Klein, M. Kotthoff and P. Voogt, Fate of a perfluoroalkyl acid mixture in an agricultural soil studied in lysimeters, Chemosphere, 2019, 223, 180–187 CrossRef CAS PubMed.
- K. Hansen, L. A. Clemen, M. Ellefson and H. Johnson, Compound-Specific, Quantitative Characterization of Organic Fluorochemicals in Biological Matrices, Environ. Sci. Technol., 2001, 35, 766–770 CrossRef CAS PubMed.
- R. Vestergren, S. Ullah, I. T. Cousins and U. Berger, A matrix effect-free method for reliable quantification of perfluoroalkyl carboxylic acids and perfluoroalkane sulfonic acids at low parts per trillion levels in dietary samples, J. Chromatogr. A, 2012, 1237, 64–71 CrossRef CAS PubMed.
- C. R. Powley, S. W. George, T. W. Ryan and R. C. Buck, Matrix effect-free analytical methods for determination of perfluorinated carboxylic acids in environmental matrixes, Anal. Chem., 2005, 77, 6353–6358 CrossRef CAS PubMed.
- J. Krippner, H. Brunn, S. Falk, S. Georgii, S. Schubert and T. Stahl, Effects of chain length and pH on the uptake and distribution of perfluoroalkyl substances in maize (Zea mays), Chemosphere, 2014, 94, 85–90 CrossRef CAS PubMed.
- A. C. Blaine, C. D. Rich, E. M. Sedlacko, K. C. Hyland, C. Stushnoff, E. R. V. Dickenson and C. P. Higgins, Perfluoroalkyl Acid Uptake in Lettuce (Lactuca sativa) and Strawberry (Fragaria ananassa) Irrigated with Reclaimed Water, Environ. Sci. Technol., 2014, 48, 14361–14368 CrossRef CAS PubMed.
- B. Prinz, G. H. M. Krause and L. Radermacher, Criteria for the evaluation of dioxins in the environment, Chemosphere, 1991, 23, 1743–1761 CrossRef CAS.
-
S. Das and S. S. Bhattacharya, Plant essential nutrients, nutrient composition in soil, and activity of plant metabolites, in Plant secondary metabolites – their roles in stress ecophysiology, ed. M. W. Siddiqui and V. Bansal, Apple Academic Press, Oakville (Canada), 2017 Search PubMed.
- Z. Liu, Z. Lu, X. Song, K. Jones, A. J. Sweetman, A. C. Johnson, M. Zhang, X. Lu and C. Su, Multiple crop bioaccumulation and human exposure of perfluoroalkyl substances around a mega fluorochemical industrial park, China: implications for planting optimization and food safety, Environ. Int., 2019, 127, 671–684 CrossRef CAS PubMed.
- I. Narvarro, A. de la Torre, P. Sanz, M. A. Porcel, J. Pro, G. Carbonell and M. Martínez, Uptake of perfluoroalkyl substances and halogenated flame retardants by crop plants grown in biosolids-amended soils, Environ. Res., 2017, 152, 199–206 CrossRef PubMed.
- B. Wen, L. Li, H. Zhang, Y. Ma, X.-Q. Shan and S. Zhang, Field study on the uptake and translocation of perfluoroalkyl acids (PFAAs) by wheat (Triticum aestivum L.) grown in biosolids-amended soils, Environ. Pollut., 2014, 184, 547–554 CrossRef CAS PubMed.
- R. Vestergren, F. Orata, U. Berger and I. T. Cousins, Bioaccumulation of perfluoroalkyl acids in dairy cows in a naturally contaminated environment, Environ. Sci. Pollut. Res., 2013, 20, 7959–7969 CrossRef CAS PubMed.
- A. Ahearn, A regrettable substitute: the story of GenX, Environ. Health Perspect., 2019 Search PubMed , https://ehp.niehs.nih.gov/doi/10.1289/EHP5134.
- P. Grandjean and R. Clapp, Changing Interpretation of Human Health Risks from Perfluorinated Compounds, Public Health Rep., 2014, 129, 482–485 CrossRef PubMed.
- M. Houde, A. O. De Silva, D. C. G. Muir and R. J. Letcher, Monitoring of Perfluorinated Compounds in Aquatic Biota: An Updated Review PFCs in Aquatic Biota, Environ. Sci. Technol., 2011, 45, 7962–7973 CrossRef CAS PubMed.
Footnotes |
† Electronic supplementary information (ESI) available: Additional graphics and tables as mentioned. See DOI: 10.1039/d1em00166c |
‡ Current address: Eurofins Dr. Specht International GmbH, Hamburg, Germany. |
§ Current address: Hamm-Lippstadt University of Applied Sciences, Department 2, Hamm, Germany. |
|
This journal is © The Royal Society of Chemistry 2021 |
Click here to see how this site uses Cookies. View our privacy policy here.