DOI:
10.1039/D1GC00603G
(Paper)
Green Chem., 2021,
23, 3295-3311
Harnessing polarity and viscosity to identify green binary solvent mixtures as viable alternatives to DMF in solid-phase peptide synthesis†
Received
16th February 2021
, Accepted 31st March 2021
First published on 7th April 2021
Abstract
Solid-phase peptide synthesis (SPPS) enables routine synthesis of virtually any type of peptide sequence and is the preferred method for peptide synthesis in academia and the pharmaceutical industry alike. Still, SPPS typically requires significant amounts of hazardous solvents and thus suffers from a negative environmental footprint. Such drawbacks have spurred numerous initiatives for solvent substitution, reduction and recycling, and a handful solvents have recently been proposed as potential green alternatives to N,N-dimethylformamide (DMF). In this report, we recognise solvent viscosity and polarity in combination as key physicochemical parameters for SPPS and identify green binary solvent mixtures of dimethyl sulfoxide (DMSO) and 1,3-dioxolane or 2-methyl tetrahydrofuran that closely resemble DMF. In a series of reagent dissolution, resin swelling, peptide coupling and Fmoc-removal experiments we show that combining solvents offers unprecedented opportunities to predict and fine-tune the overall solvent properties for different aspects of SPPS. Lastly, the identified green binary solvent mixtures were employed for the synthesis of a range of challenging model peptides and peptide therapeutics on meaningful scale, demonstrating that binary solvent mixtures are viable green alternatives to DMF in SPPS.
Introduction
Peptides and proteins play a fundamental role in the design of life and are increasingly important as active pharmaceutical ingredients (APIs) in a wide range of therapeutic areas.1 Traditionally hampered by poor oral bioavailability and short plasma half-life, significant advances in peptide modification and conjugation, as well as formulation, has led to a surge in therapeutic peptide development, and as of 2017 over 150 peptide drugs were in active clinical trials.2–4 The landmark technological breakthrough of solid-phase peptide synthesis (SPPS), first described by Merrifield in 1963,5 has transformed the field and is today the preferred method for peptide synthesis in academic research and large-scale pharmaceutical development alike.6,7 The principle of SPPS is the step-wise assembly of peptides using amino acids anchored to a solid support, usually from C- to N-terminus of the peptide, through a series of coupling, deprotection and wash cycles. Importantly, SPPS is scalable and enables the synthesis of virtually any type of peptide sequence (typically <50 amino acids). However, despite significant advances within most aspects of the process, e.g. protection groups,8 coupling reagents and reaction conditions,9 SPPS remains an overall wasteful endeavour. Coupling reagents and protected amino acid reagents are typically used in excess to push each reaction to completion in reasonable reaction times, and significant amounts of solvents are used for peptide washing, isolation and purification. Tellingly, the manufacture of peptide APIs generates several tons of waste per kilogram API, where solvents constitute the main component.10–12 In addition, the solvents commonly used such as N,N-dimethylformamide (DMF), dichloromethane (DCM) and N-methyl-2-pyrrolidone (NMP) are hazardous (DMF13 and NMP14 are reprotoxic and DCM15 is suspected carcinogenic) and are heading for restriction under the European Chemicals Agency's (ECHA) Registration, Evaluation, Authorisation and Restriction of Chemicals (REACH).16,17 In the search for alternatives, solvent classifications based on a holistic assessment of environment, health, safety, recyclability and CO2 footprint parameters etc. have in recent years been compiled into helpful selection guides for green solvent replacement.18–20 However, the conclusions from these guides often depend on the in-house experience and capabilities of the authoring teams, and may not be widely applicable to specific projects or technologies. In the search for a DMF substitute in large-scale SPPS, we have therefore decided to focus on environmental and safety aspects based on the ECHA-REACH status, and herein we define green solvents from this perspective.21 Significant work has been done by teams in both academia and industry over the last decade in making peptide synthesis greener,22–25 with recent focus on solvent substitution, reduction and recycling in SPPS. More benign solvents investigated for use in various stages of the SPPS process include acetonitrile (MeCN),26,27 2-methyl tetrahydrofuran (2-Me-THF),28–31 ethyl acetate (EtOAc),27,29,31 anisole,27 dimethyl carbonate (DMC),27,31N-butylpyrrolidone (NBP),11,31,32 γ-valerolactone (GVL),31,33–37 cyclopentyl methyl ether (CPME),30,31 4-methyl tetrahydropyran (MTHP),31 and N-formylmorpholine (NFM).34 While these reports collectively show that it is possible to replace DMF for certain applications, solvent specific drawbacks such as the propensity for GVL to induce ring-opening acylation of glycine residues,35,36 or the high viscosity of NBP,11,31,32 indicate limits for finding a neat solvent that fully matches the performance of DMF in SPPS. Consequently, binary solvent mixtures have been proposed as an alternative. SPPS using mixtures of dimethyl or diethyl carbonate with Cyrene™, sulfolane or anisole,38 and mixtures of propylene carbonate (PC) with EtOAc or tetramethyl oxolane (TMO),39 have been reported. Importantly for large-scale use, mixtures of EtOAc with MeCN, NBP or DMSO have been demonstrated to offer cost-efficient alternatives to DMF with the potential for solvent recycling,27,40 albeit recycling solvents in API production is often avoided in the pharmaceutical industry due to regulatory concerns. Moreover, recycling specific solvents such as DMSO by distillation is associated with safety concerns,41 and hence for a green solvent or solvent mixture to be impactful across industry and academia there are a number of technical and regulatory requirements that need to be fulfilled, which has been thoroughly discussed in recent reviews.11,24,25,42 Furthermore, it is becoming increasingly clear that intrinsic solvent properties such as polarity, are of utmost importance for SPPS process performance.11,38,39 In this project, we set out to identify environmentally benign solvents or solvent mixtures amenable to large-scale SPPS of therapeutic-grade peptides. We hypothesised that determining key physical parameters would allow us to identify alternatives to DMF. Herein, we redefine the preferred physicochemical SPPS solvent space using solvent polarity and viscosity parameters and identify a focussed set of viable green binary solvent mixtures used for the synthesis of challenging peptide sequences, as well as therapeutically relevant peptides.
Results and discussion
At the outset of the project we identified a list of desirable traits that should be inherent to a new green SPPS process solvent under standard conditions at ambient temperature (vide infra). If these qualities are met, technical challenges in the pilot plant environment can be minimised and product output and manufacturing time kept at an acceptable level.24,25,42
• Solvent melting point ≤ 10 °C
• Solvent viscosity < 4 mPa s
• Reagents and by-products solubility ≥ 0.25 M
• Starting resin swelling 4–7 mL g−1
• Coupling time ≤ 1 hour
• Fmoc-removal time ≤ 30 min
To identify green solvent candidates for SPPS we conducted a comprehensive analysis of a wide range of polar aprotic process solvents, assessing the solvent's chemical and physical properties and compliance with REACH authorisation,21 candidate list (SVHC, Substances of Very High Concern),16 PACT (The Public Activities Coordination Tool),43 and data for endocrine disruptors and CMR (Carcinogenic, Mutagenic or Toxic for Reproduction). We selected 19 solvents with an acceptable environmental profile (herein defined as green solvents) that could be used neat or as mixtures (Table 1) and proceeded with an evaluation against the criteria outlined above, starting with investigating reagent solubility, solvent stability and resin swelling.
Table 1 Solvents selected for SPPS assessment based on REACH data
Solvent |
Abbreviation |
Structure |
Melting pointa (°C) |
Viscositya (mPa s) |
The solvent melting point and viscosity data was collated from chemical databases such as ECHA,21 SciFinder,44 Reaxys,45 and material safety data sheets (MSDS) from chemical vendors.
1,3-Dioxolane is under REACH evaluation as some suppliers identify the substance as toxic to reproduction. However, under the current harmonised classification and labelling 1,3-dioxolane is considered unproblematic for use and in compliance with REACH authorisation.46
|
Acetonitrile |
MeCN |
|
−46 |
0.35 |
t-Amylmethylether |
TAME |
|
−20 |
0.54 |
n-Butylacetate |
— |
|
−78 |
0.73 |
N-Butylpyrrolidinone |
NBP |
|
<−75 |
4.0 |
Cyrene™ |
— |
|
−20 |
14.5 |
Diethyl carbonate |
DEC |
|
−43 |
0.84 |
Dimethyl carbonate |
DMC |
|
−3 |
0.59 |
Dimethylisosorbide |
DMI/DMIE |
|
−84 |
6.80 |
Dimethyl sulfoxide |
DMSO |
|
19 |
2.14 |
1,3-Dioxolaneb |
DOL |
|
−90 |
0.59 |
Ethyl acetate |
EtOAc |
|
−84 |
0.45 |
N-Formylmorpholine |
NFM |
|
23 |
7.87 |
2-Methyl tetrahydrofuran |
2-Me-THF |
|
−20 |
0.58 |
4-Methyl tetrahydropyran |
4-Me-THP |
|
−50 |
0.78 |
Nitromethane |
— |
|
−28 |
0.65 |
Propylene carbonate |
PC |
|
−49 |
2.76 |
Tetrahydropyran |
THP |
|
−49 |
0.81 |
2,5,7,10-Tetraoxaundecane |
TOU |
|
−65 |
1.52 |
γ-Valerolactone |
GVL |
|
−31 |
2.18 |
Solubility of amino acid derivatives and SPPS-reagents
Four amino acid (AA) derivatives (Fmoc-Arg(Pbf)-OH, Fmoc-Gln(Trt)-OH, Fmoc-Gly-OH and Fmoc-Phe-OH) known to be difficult to solubilise at high concentration were selected for the solubility study. Inspired by recent reports employing binary solvent mixtures for SPPS,38–40 we initially included four binary solvent mixtures of selected green solvents (EtOAc in combination with Cyrene™, DMSO, NBP or NFM) in addition to the 19 neat solvents and DMF as reference. The solvents were first screened for their ability to dissolve the AA derivatives at a high concentration (0.40 M), and if failing this, the target concentration was lowered to 0.25 M (Table 2). In total, 13 out of 19 neat solvents were unable to fully dissolve all four AA derivatives at a concentration of ≥0.25 M. Although it is ultimately the solubility of the activated AA derivative that determines the effective AA concentration in the reaction mixture, poor solubility of the starting AA derivative introduces unwelcome variability and indicates poor process utility for these solvents in SPPS. While a binary mixture of two poor solvents (i.e. Cyrene™ and EtOAc) resulted in poor solubility, mixing EtOAc with the good solubilisers NBP, DMSO and NFM resulted in an acceptable solubility for the four AA derivatives.
Table 2 Solubility of amino acid (AA) derivatives in DMF, neat green solvents and selected binary mixtures
The solvent (10 mL) was added to the AA derivative (4 mmol) and the mixture was stirred at 25 °C for 30 min. Subsequent visual evaluation was qualitatively reported as soluble (S; green colour), or insoluble (I; red colour). AA derivatives insoluble at 0.40 M were further assessed for their solubility at 0.25 M (S; yellow colour).
The solubility of Fmoc-Phe-OH was generally only assessed provided the three other AA derivatives were soluble. ND = not determined.
|
|
Based on these results, six neat solvents and three binary solvent mixtures were next assessed for their ability to dissolve commonly employed coupling reagents. Unlike the previous experiment with AA derivatives it was ideal but not essential that all coupling reagents were soluble at the target concentration of 0.40 M. Furthermore, the solubility of the by-product diisopropylurea (DIU), resulting from diisopropylcarbodiimide (DIC) mediated couplings was also assessed (Table 3). Due to process safety considerations regarding coupling reagents containing the benzotriazole motif,47–49 such reagents are generally avoided in large-scale synthesis of peptides, and consequently these reagents were not included in the solubility study. The success criterion was to achieve dissolution of the most commonly employed coupling reagents in our facilities (OxymaPure and DIC). All solvents were in fact capable of dissolving OxymaPure and DIC, while DIU was poorly soluble or insoluble in all solvents except NBP and DMSO. From a process chemistry perspective, DIU could accumulate during SPPS and clog filters and tubes of SPPS reactors, unless large volumes of solvent are employed for washing. The use of isopropyl alcohol (IPA) has been reported for resin wash after coupling and Fmoc-removal,29,40,50 and we verified the solubility of DIU in IPA at 0.40 M (Table 3). With the indication that washing volumes could be significantly reduced by incorporating IPA washes for solubilisation of DIU by-products, IPA washes were incorporated in all SPPS experiments reported herein.
Table 3 Solubility of coupling reagents and diisopropylurea (DIU)
The solvent (10 mL) was added to the compound (4 mmol) and the mixture was stirred at 25 °C for 30 min. Subsequent visual inspection was qualitatively reported as soluble (S; green colour), or insoluble (I; red colour). Compounds insoluble at 0.40 M were further assessed for their solubility at 0.25 M (S; yellow colour).
DIU solubility was assessed at 0.10, 0.20, 0.30 and 0.40 M.
For isopropyl alcohol (IPA) only the solubility of DIU was determined. ND = not determined.
|
|
Assessment of solvent stability
Any solvent comes with its own challenges and risks for cross reaction and side-product formation, and for certain neat green solvents used in SPPS, specific drawbacks can be pronounced (e.g. ring-opening acylation of glycine residues with GVL,35,36 and Arg-lactamisation as a consequence of the high viscosity of NBP11,32). To test the stability of the solvents examined in the present work a selection of solvents were subjected to stressed conditions in the presence of a peptide resin with a free amino group. Neat EtOAc did not perform well in the solubility experiments but was in scope as part of binary mixtures and was thus included, as was GVL as a control. The solvent stability was assessed both without additive and under acidic and basic SPPS conditions using HOBt and N,N-diisopropylethylamine (DIPEA) respectively. In order to thoroughly test the system, HOBt was used here as a less stable acidic additive compared to OxymaPure.48 The peptidyl H-Gly-Ala-Phe-Phe-Ala-tricyclic amide resin was incubated in a given solvent at 40 °C for seven days, then washed, cleaved and the resulting crude peptide was analysed by HPLC (Fig. 1). In line with previous reports,35,36 significant ring-opening acylation of the N-terminal Gly was observed in GVL (giving diastereomeric acylated by-products). More surprisingly, the peptide N-terminus was also found to react with DMF and EtOAc. An N-formylated side-product, due to the partial degradation of DMF,51 was observed exclusively under acidic conditions (HOBt), while a minor percentage of N-acetylation was observed in EtOAc under all conditions. Moreover, despite a number of washing steps after cleavage, some NBP was carried over to the obtained crude peptide as seen in the HPLC profile, illustrating how the high viscosity of NBP affects the efficiency of resin washing negatively. Besides this, the remaining tested solvents (DMSO, DOL, THP and 2-Me-THF) showed overall good stability (Fig. 1).
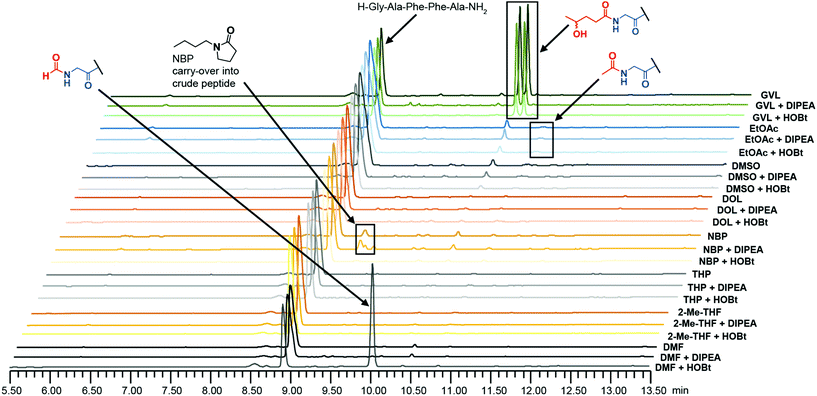 |
| Fig. 1 Assessment of the stability of selected green solvents. The H-Gly-Ala-Phe-Phe-Ala-NH2 peptide (cleaved from tricyclic amide resin) was analysed by HPLC (220 nm) after being incubated in the indicated solvent at 40 °C for seven days, then washed and cleaved. Identified side-products and impurities are highlighted. | |
Resin swelling
The success of peptide synthesis on solid support is heavily governed by the resin matrix to which the peptide chain is anchored.52 Polystyrene (PS) resins are extensively utilised as solid supports in SPPS, especially in industrial settings, due to robust supply chains, low cost and high chemical stability.53 The resin swelling is of particular importance, as optimum swelling ensures sufficient diffusion of reagents within the solid support to access the reaction sites. Consequently, in pursuit of finding green solvent alternatives to DMF in SPPS, it is imperative to know the resin swelling propensities in such solvents. To this end, several research groups have recently reported resin swelling experiments and SPPS using various alternative green solvents and their binary mixtures.11,22,38,54–57 In particular, Magtaan et al. demonstrated correlation between volumetric and optical microscopy-based methods on a range of resins using green solvents to aid the selection of suitable resin-solvent combinations.57 Moreover, Ran et al. used the Hansen solubility parameter in practice (HSPiP) software for predicting the resin swelling capacity in various green solvents and reported that binary mixtures of green solvents not only exceeded the performance of their individual component but also could be judiciously fine-tuned to mimic the swelling properties of DMF.39 Since the swelling of resin is affected by its microenvironment and growing peptide chain,58–60 we decided to test the swelling propensity of solvents and their binary mixtures for both starting as well as peptide-functionalised resins using the volumetric method.61 Six neat solvents and three binary mixtures were employed for swelling a series of starting PS and ChemMatrix resins, and benchmarked against DMF (Table 4). In general, the excessive swelling of ChemMatrix resins excludes them for practical use in large-scale SPPS (ESI Table S1†). Five neat solvents (NBP, DMSO, DOL, NFM and THP) gave swelling outside the preferred range from 4–7 mL g−1 for different starting resins, indicating that they are not generally applicable to green SPPS. In contrast, binary mixtures of three of these solvents with EtOAc resulted in the desired swelling for all resins (Table 4), again demonstrating the possibility of fine-tuning solvent performance by using binary mixtures. Due to the poor swelling of several resins, neat NFM was not further explored.
Table 4 Swelling of starting resins in neat solvents and solvent mixtures reported in mL g−1
The solvent was added to the designated resin (1.0 g) to give a final volume of 10 mL, and after 3 h incubation the resin volume was measured. Green: 4–7 mL g−1 (sufficient swelling); orange: < 4 mL g−1 (insufficient swelling); blue: > 7 mL g−1 (excessive swelling).
Resin loading (mmol g−1) and particle mesh size: 0.42 (100–200 mesh).
0.74 (100–200 mesh).
0.70 (75–150 mesh).
0.25 (100–200 mesh).
0.23 (100–200 mesh). Green: 4–7 mL g−1 (sufficient swelling); orange: < 4 mL g−1 (insufficient swelling); blue > 7 mL g−1 (excessive swelling). |
|
In addition to the starting resin swelling experiments, we aimed to investigate the dynamic swelling response during peptide synthesis in different solvents. Understanding the response characteristics of the resin i.e., the ability of the resin to gradually swell to its maximum capacity in the solvent used, is of substantial importance with regard to repeated solvent treatment of peptide resins during SPPS cycles. Octreotide peptidyl resin (8 AAs) as well as the corresponding starting resin (Fmoc-L-Thr(ol)-2-chlorotrityl resin (CTR), 1 AA) were subjected to a series of sequential solvent treatments. As an example, the gradual swelling of the resin in DMF or 2-Me-THF and its gradual shrinkage in IPA is illustrated in Fig. 2 (data for other solvents and binary solvent mixtures is provided in ESI Fig. S1A–D†).
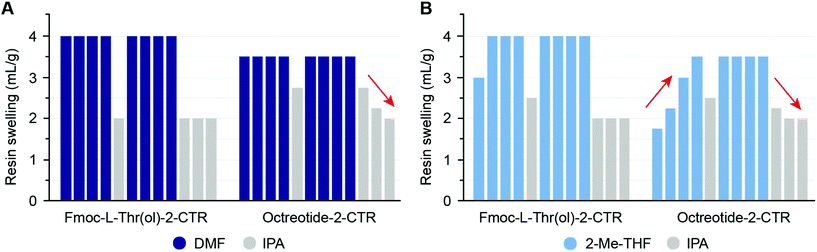 |
| Fig. 2 Swelling response characteristics of Octreotide peptidyl resin (Octreotide-2-CTR, 0.37 mmol g−1 loading) and its starting resin (Fmoc-L-Thr(ol)-2-CTR, 0.84 mmol g−1 loading) in DMF (A) and 2-Me-THF (B). The designated resin (1.0 g) was sequentially treated with solvent (10 mL) as indicated by the order of the bars from left to right (full experimental details in the ESI†). The red arrows highlight the resin response characteristics. | |
As anticipated the Octreotide peptidyl resin showed reduced swelling compared to its starting resin (Fmoc-L-Thr(ol)-2-CTR) in both DMF and 2-Me-THF. A gradual increase in swelling (moderate response characteristics) of the Octreotide peptidyl resin was observed in 2-Me-THF, while DMF gave homogeneous swelling (good response characteristics). In total, four different PS resins (Wang and 2-chlorotrityl linker) loaded with peptides of varying length, i.e. Octreotide (8 AAs), Bivalirudin (20 AAs), Glucagon (29 AAs) and Aprotinin (58 AAs) were subjected to the sequential solvent-IPA treatment in neat green solvents and binary solvent mixtures. No general trend of resin swelling and response characteristics was observed in neither neat solvent nor binary mixtures, illustrating that several parameters could influence the swelling of peptidyl resins, e.g. resin loading, cross-link density, mesh size, molecular and supramolecular properties of resin-loaded peptide, as well as the type of side-chain protection groups.54 Nevertheless, the peptidyl resin generally showed reduced swelling compared to its corresponding starting resin and this phenomenon became more pronounced with increasing peptide length. The swelling propensity and response characteristics of the shortest and longest tested resin-bound peptides (8-mer Octreotide-2-CTR and 58-mer Aprotinin-Wang respectively) in selected neat solvents and binary mixtures is illustrated in Fig. 3 (swelling data for all peptidyl resins is available in ESI Fig. S1A–D†). Furthermore, while the swelling of the starting resins vary between the solvents, these differences decrease with increasing peptide length. Thus, for the Glucagon-Wang resin (29 AAs) and Aprotinin-Wang resin (58 AAs) the swelling is similar in all solvents and solvent mixtures, illustrating how the growing peptide chain exerts increasing influence on the overall resin swelling (ESI Fig. S1C and D†). Overall, the swelling capacity of the neat solvents 2-Me-THF, DOL, DMSO, and the binary mixtures DMSO/EtOAc (2
:
8) and NBP/EtOAc (2
:
8) were comparable to DMF. NBP/DOL (2
:
8) was included here to illustrate that, in similarity with neat solvents, the swelling capacity of binary solvent mixtures can be dependent on peptidyl-resin type. We observed that NBP was slow to drain, and a substantial amount of resin beads were found lingering on the inner wall of the reactor for both NBP and DMSO, presumably due to the high viscosities of the solvents. These solvents also showed a slow response rate of swelling, again believed to be an effect of high viscosity and slow diffusion.
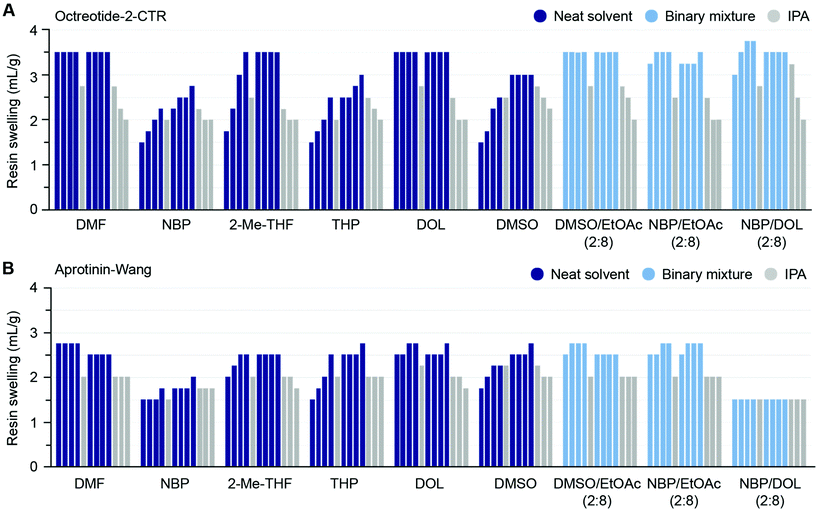 |
| Fig. 3 Swelling in mL g−1 of (A) Octreotide-2-CTR (8-mer, 0.37 mmol g−1 loading) and (B) Aprotinin-Wang resin (58-mer, 0.073 mmol g−1 loading) in selected neat solvent (dark blue), binary solvent mixtures (light blue), and IPA (grey). The order of the bars from left to right indicates the sequence of solvent treatment. | |
From the results of solubility and swelling experiments we concluded that solvent polarity appeared to govern dissolution of amino acid derivatives and coupling reagents, while resin swelling was additionally influenced by the solvent viscosity. DMF clearly strikes a good balance between these properties, and while no individual neat solvent could fully match the performance of DMF in all these experiments, it was evident that the binary solvent mixtures provide opportunities for fine-tuning of solvent properties. We thus hypothesised that by determining the preferred polarity and viscosity space for SPPS solvents, governed by the physicochemical properties of DMF, we should be able to identify alternative solvents or binary solvent mixtures that display similar characteristics and therefore perform on par with DMF in SPPS.
Assessing neat green solvents and binary solvent mixtures by determining viscosity and polarity
We started by determining the viscosities of the selected neat green solvents and a range of their binary mixtures at 20 °C, and found that it was indeed possible to modulate the viscosity of the binary mixtures to closer resemble the value of DMF (0.83 ± 0.05 mPa s) by varying the ratio of the individual components. For example, highly viscous solvents such as DMSO and NBP (2.08 and 3.30 mPa s respectively) could be rendered less viscous by mixing in increasing amounts of solvents with lower viscosity such as 2-Me-THF, DOL or EtOAc (Fig. 4A and ESI Table S2†). The facile tailoring of the viscosity of the binary solvent mixtures was encouraging since it has a direct impact on the resin swelling (via diffusion and draining) and thereby the success of SPPS. We proceeded with investigating solvent polarity since the ability of DMF to function as a universal solvent in SPPS has been linked to its optimal polarity.11,38,39 To this end, we used the Reichardt polarity index, which is a UV/Vis spectrophotometric determination of the molar transitional energy of a solvatochromatic zwitterionic pyridinium N-phenolate betaine dye dissolved in the designated solvent.62,63 The polarity was measured for a wide range of green binary solvent mixtures and the relevant neat solvents. NBP and DMSO are the neat solvents that most resemble DMF in polarity, and by combining different ratios of these with solvents of lower polarity (e.g. EtOAc, DOL and 2-Me-THF) it was possible to obtain solvent mixtures with overall polarity that closely matched DMF (Fig. 4B and ESI Tables S3, S4†). To fully leverage the physicochemical data, we plotted the solvent viscosity over polarity and identified a small subset of solvent combinations and ratios that closely matched DMF in both characteristics (Fig. 4C). The data suggests that based on solvent viscosity or polarity data alone, neat solvents that resemble DMF (e.g. viscosity of 2-Me-THF or polarity of NBP) may seem attractive as substitutes in SPPS. However, in combination it is evident that DMF populates a significantly different physicochemical space that is for example more closely mimicked by a mixture of 2-Me-THF and NBP than by either of the neat solvents. The relatively high polarity of DMF was especially challenging to match and therefore the solvent combinations that most closely mimicked DMF were mixtures of highly polar DMSO with EtOAc, DOL and 2-Me-THF (Fig. 4C). With this insight in mind, we proceeded with evaluating Fmoc-removal and peptide coupling reactions.
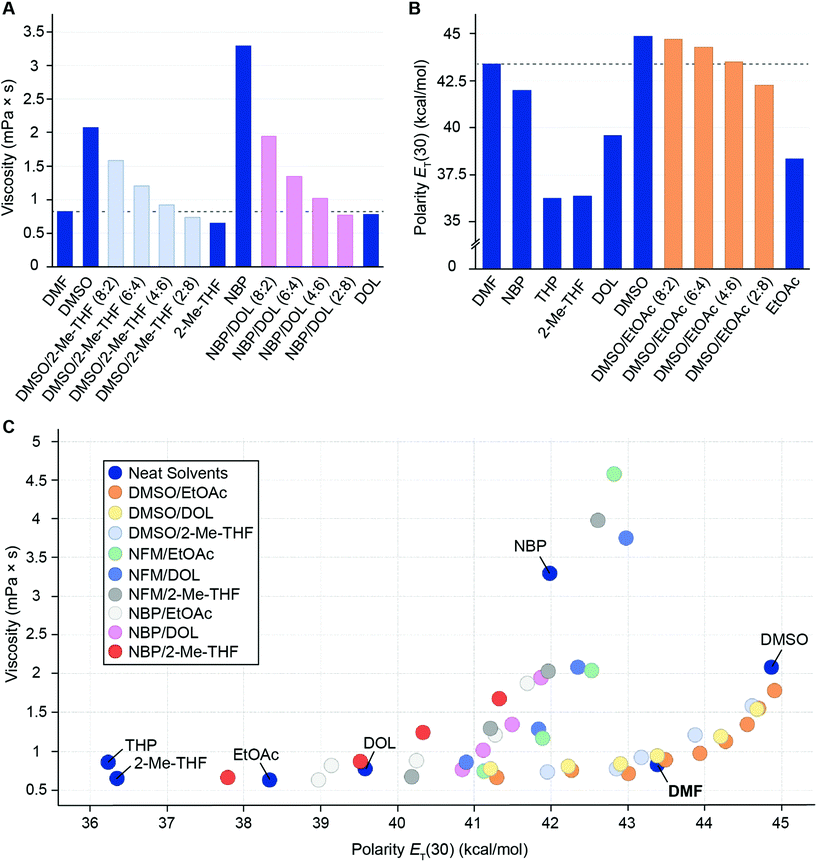 |
| Fig. 4 (A) Measured viscosity (mPa s) of selected neat green solvents (DMSO, 2-Me-THF, NBP and DOL) and their binary mixtures, benchmarked against DMF (dashed line). (B) Measured solvent polarity (ET(30)) (kcal mol−1) of selected neat green solvents (DMSO, EtOAc, 2-Me-THF, THP, NBP and DOL) and binary mixtures of DMSO and EtOAc using the Reichardt polarity index,62,63 benchmarked against DMF (dashed line). (C) Viscosity (mPa s)–Reichardt polarity (kcal mol−1) plot for the relevant neat green solvents (indicated by name) and binary mixtures. With increasing amounts of viscous or polar solvent (e.g. NBP or DMSO) in the binary mixture, the overall viscosity and polarity of the mixture increases, causing an upward (viscosity) or rightward (polarity) shift in the plot. | |
Peptide bond formation and Fmoc-removal kinetics in neat solvents and binary mixtures
Peptide bond formation (coupling) efficiency in various solvents was assessed by monitoring the incorporation of Fmoc-Gly-OH on a resin-bound peptide (H-Pro-Phe-Ala-CTR) over time using DIC and OxymaPure as coupling reagents. We screened a focused number of neat green solvents and binary solvent mixtures at a fixed 2
:
8 or 4
:
6 ratio. In general, there was an inverse correlation between solvent polarity and coupling efficiency, i.e. the coupling efficiency was higher in solvents of lower polarity. The neat solvents THP, 2-Me-THF and DOL, gave faster incorporation of Fmoc-Gly-OH compared to DMF, while NBP and DMSO gave moderate to slow coupling with incomplete incorporation of the AA derivatives after 60 min (Fig. 5A and ESI Table S5A†). Similarly, binary solvent mixtures with high content of lower polarity solvents (e.g. NFM/DOL, NBP/DOL and NBP/2-Me-THF) showed fast coupling kinetics, while the coupling kinetics were reduced in mixtures with high polarities (e.g. DMSO/EtOAc and DMSO/2-Me-THF), generally being more similar to DMF (Fig. 5B and ESI Tables S5A–C†). In line with previous observations for reagent solubility, solvent viscosity and polarity, we concluded that neat solvents with slow coupling kinetics can be mixed with better performing solvents to yield solvent mixtures that result in good coupling efficiency. For example, DMSO or NBP can be mixed with 2-Me-THF or DOL to give mixtures that perform on par with or better than DMF for peptide bond formation. In particular, DOL appears to be an attractive component for binary solvent mixtures to promote efficient coupling reactions in SPPS.
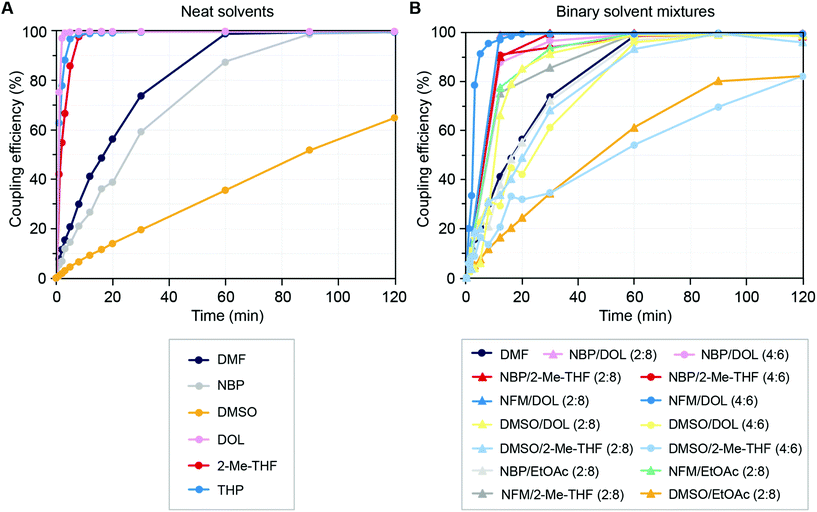 |
| Fig. 5 Kinetics for the coupling of Fmoc-Gly-OH onto H-Pro-Phe-Ala-CTR using DIC/OxymaPure in (A) neat solvents and (B) selected binary solvent mixtures, benchmarked against DMF. The conversion of tri- to tetrapeptide was quantified by HPLC analysis after Fmoc-removal, resin wash and peptide cleavage. The coupling reaction was defined as fast to moderate for >99% incorporation of Fmoc-Gly-OH within 60 to 90 min, respectively, while reactions with <98% conversion after 90 min were considered slow. | |
Following the coupling kinetics experiments we went on to assess the efficiency of Fmoc-removal in the selected neat solvents and binary mixtures. A tetrapeptide (Fmoc-Ala-Phe-Phe-Ala-tricyclic amide linker resin) was treated with a 20% (v/v) solution of piperidine in the designated solvent. At specific time intervals, the supernatant solution was spectroscopically analysed for the in situ formed dibenzofulvene-piperidine adduct which directly correlates to the extent of Fmoc-removal. Of the neat solvents, only DMSO gave Fmoc-removal with similar efficiency to DMF with >95% reaction completion within 10 min (Fig. 6A and ESI Table S6A†). Interestingly, the relatively polar NBP performed only slightly better than the non-polar solvents THP and 2-Me-THF, all giving poor (<40%) Fmoc-removal within 90 min. Consequently, the binary mixtures of NBP with 2-Me-THF also gave poor Fmoc-removal, while mixing NBP with DOL or EtOAc significantly increased the rate of Fmoc-removal (Fig. 6B and ESI Tables S6A–C†). In general, the data shows that Fmoc-removal is strongly favoured in polar solvents or solvent mixtures, with efficient Fmoc-removal achieved in most solvent combinations including DMSO (e.g. with EtOAc, DOL and 2-Me-THF) at a DMSO concentration of ≥40% in the binary mixture. This observation is in accordance with the E1cB mechanism of Fmoc-removal with the negatively charged intermediate being better stabilised in polar solvents, and it further underscores the importance of solvent polarity in SPPS.
 |
| Fig. 6 Fmoc-removal kinetics for Fmoc-Ala-Phe-Phe-Ala-tricyclic amide linker resin in (A) neat solvents and (B) selected binary solvent mixtures, benchmarked against DMF. The percentage of in situ formed dibenzofulvene–piperidine adduct was quantified by UV spectroscopy at 289.8 nm. | |
Collectively, the results from reagent solubility and resin swelling experiments together with the reaction kinetics study illustrate how solvent viscosity and polarity affect different aspects of SPPS, and in consequence that these critical physicochemical properties need to be thoroughly optimised for efficient use of alternative solvents in SPPS. DMF intrinsically balances these properties in a way that makes it a very useful solvent in SPPS, with efficient reagent solubility and resin swelling properties, efficient Fmoc-removal and sufficient peptide coupling kinetics. While no neat green solvent could match DMF in this fashion, it was indeed possible to modulate both viscosity and polarity by using binary solvent mixtures, and obtain new combinations with properties that matched DMF. Motivated by these results and determined to test the hypothesis that polarity and viscosity both play important roles in SPPS, we decided to proceed with the synthesis of model peptides.
Solvent polarity and viscosity of binary solvent mixtures govern peptide purity in SPPS
Based on the coupling and Fmoc-removal study we decided to focus on a specific range for solvent polarity (Reichardt polarity index was set at 39–45 kcal mol−1) and viscosity (0.5–1.1 mPa s) going forward. In this range we identified 20 green binary solvent mixtures and one neat solvent (DOL), all giving appropriate resin swelling and acceptable reagent dissolution. At first, we selected the peptide Ac-Leu-Val-Ala-Tyr-Ala-Gly-NH2 (Rink amide PS resin) as a simple model incorporating side chains unlikely to undergo side-reactions during SPPS. The manual synthesis of this model peptide was then carried out in a majority of the selected solvents. However, four solvent mixtures (NBP/EtOAc (2
:
8), NFM/EtOAc (2
:
8), DMSO/DOL (1
:
9), and DMSO/2-Me-THF (2
:
8)) were excluded along the way based on the overall synthesis trend suggesting that they would not be useful. The results showed that in general the solvents with the most similar viscosity-polarity profile to that of DMF gave crude peptide with the highest purities (orange box, Fig. 7A), whereas solvent combinations further from DMF gave lower peptide purities (Fig. 7A and ESI Table S7†). It was however challenging to make a distinct discrimination between the solvents due to the simplicity of the model peptide and the overall high purities. For better evaluation of polarity–viscosity dependency of binary solvent mixtures, the more challenging sequence Aib-enkephalin (Ac-Tyr-Aib-Aib-Phe-Leu-OH) was selected, which is a commonly employed target in SPPS method development.26,29,48 Although a short peptide containing only five AAs, the presence of two sterically hindered aminoisobutyric acids (Aib) increases the difficulty of the sequence. Six promising binary mixtures of DMSO in combination with DOL (3
:
7 and 4
:
6), 2-Me-THF (3
:
7 and 4
:
6), and EtOAc (4
:
6 and 5
:
5) with a polarity–viscosity profile close to DMF were used for the synthesis along with DMF as a reference. The results showed that most of the tested solvent combinations gave similar or slightly lower peptide purity than DMF (35–46% compared to 48% for DMF), while DMSO/2-Me-THF (3
:
7) gave higher purity than DMF (53%). Encouraged by this finding, we decided to move forward with the ratio of each binary solvent mixture that physicochemically most closely resembled DMF (DMSO/2-Me-THF (3
:
7), DMSO/EtOAc (4
:
6), and DMSO/DOL (3
:
7)) for the synthesis of a panel of challenging model peptides to fully assess the utility of these green binary solvent mixtures in SPPS.
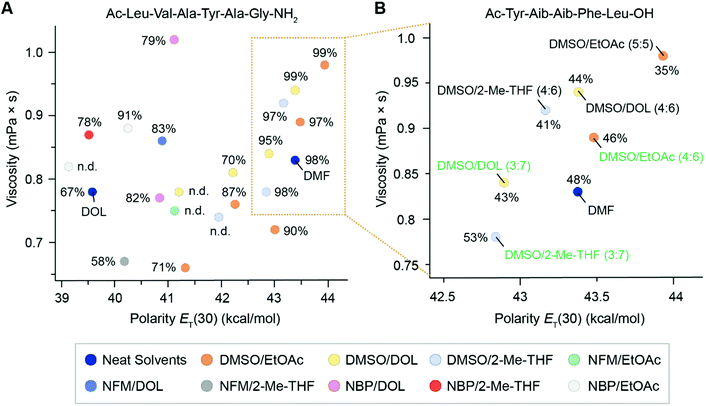 |
| Fig. 7 (A) Solvent viscosity–polarity plot showing crude peptide purities (%) for the manual synthesis (1.5 mmol scale) of the model peptide Ac-Leu-Val-Ala-Tyr-Ala-Gly-NH2 using DIC/Oxyma (4/3 equiv.) coupling reagents in selected binary solvent mixtures, DOL and DMF as reference. The purities were determined by the area under the curve (AUC) using HPLC analysis at 214 nm. The full table of solvent mixtures and purities are listed in ESI Tables S7 and S8.† A cluster of six high-performing solvent mixtures resembling DMF are highlighted in the orange box. (B) Expanded solvent viscosity–polarity plot showing crude peptide purities (%) for the manual synthesis (0.4 mmol scale) of the model peptide Ac-Tyr-Aib-Aib-Phe-Leu-OH using DIC/Oxyma (4/3 equiv.) coupling reagents in six selected solvent mixtures and DMF as reference. The purities were determined by the area under the curve (AUC) using HPLC analysis at 214 nm. Three well-performing solvent combinations and ratios selected for further investigation are indicated in green. | |
Green SPPS of challenging model peptides using binary solvent mixtures
At this point, three green solvent combinations and ratios that performed on par with DMF for the synthesis of short model peptides of limited complexity had been identified. To fully assess their general utility for SPPS we undertook automated synthesis of Aib-enkephalin and three additional peptides with increased complexity:
• Aib-enkephalin (Ac-Y-Aib-Aib-FL-NH2)
• Jung-Redemann (H-WFTTLISTIM-NH2)
• Thymosin α1 (Ac-SDAAVDTSSEITTKDLKEKKEVVEEAEN-NH2)
• Dasiglucagon amide (H-HSQGTFTSDYSKYLD-Aib-ARAEEFVKWLEST-NH2)
The Jung-Redemann and Thymosin α1 peptides are both challenging sequences,64–66 and Dasiglucagon (with COOH at the C-terminus) is a glucagon analogue in clinical trials for treatment of severe hypoglycaemia,67 thus representing a relevant model peptide therapeutic. All four peptides were synthesised in duplicate on a Symphony X automated peptide synthesiser using Rink amide PS resin on 0.15 mmol scale in the different solvents. Due to the small scale and fixed volumes for reagent addition in the automated setup, we used an increased amount of DIC coupling reagent (4 + 4 equiv.) compared to the manual synthesis. Both the purity of the target peptide and the resin weight gain were measured in order to evaluate the extent of any unintentional peptide cleavage from the resin during synthesis. For the synthesis of Aib-enkephalin the purities were generally higher than what was observed for the manual synthesis (vide supra), which is likely explained by the increased equivalents of coupling reagent and extended coupling times in the automated synthesis. Regardless, DMSO/DOL (3
:
7) was again inferior to DMF, while the peptide purity in DMSO/2-Me-THF (3
:
7) was higher than in DMF (Table 5). For the synthesis of the remaining peptides (Jung-Redemann, Thymosin α1 and Dasiglucagon amide) all three solvent mixtures generally gave somewhat lower purities than those obtained in DMF (Table 5). Despite some sequence dependence for the peptide purity when using binary solvent mixtures, we were encouraged by the overall performance of the mixtures investigated. In particular, DMSO/DOL (3
:
7) and DMSO/2-Me-THF (3
:
7) performed well for most targets and were further investigated through scaled-up peptide synthesis.
Table 5 Purities and resin weight gain of four model peptides synthesised on the automated Symphony X peptide synthesisera
Solvent |
Aib-Enkephalin (5-mer) |
Jung-Redemann (10-mer) |
Thymosin α1 (28-mer) |
Dasiglucagon amide (29-mer) |
Purityb (%) |
Resin weight gain (%) |
Purityb (%) |
Resin weight gain (%) |
Purityc (%) |
Resin weight gain (%) |
Purityc (%) |
Resin weight gain (%) |
All syntheses were carried out in duplicate or triplicate on Rink Amide PS resin (0.74 mmol g−1 loading), 0.15 mmol scale and the average resin weight gain and peptide purity is reported.
Purity (in %) determined from AUC using HPLC analysis at 210 nm.
Purity (%) determined from AUC using HPLC analysis at 214 nm.
|
DMF |
69 |
99 |
57 |
87 |
52 |
85 |
59 |
89 |
DMSO/EtOAc (4 : 6) |
44 |
105 |
52 |
90 |
41 |
81 |
49 |
85 |
DMSO/2-Me-THF (3 : 7) |
75 |
115 |
53 |
90 |
47 |
85 |
50 |
87 |
DMSO/DOL (3 : 7) |
59 |
73 |
52 |
64 |
42 |
73 |
53 |
75 |
Scaled-up green SPPS of Dasiglucagon amide and Bivalirudin
To be a fully viable alternative to DMF in peptide synthesis and production it is critical that any new binary solvent mixture is applicable to peptide synthesis on production scale. To this end, we scaled-up the synthesis of two peptide therapeutics, Dasiglucagon (29-mer, vide supra) and the anti-coagulant Bivalirudin (20-mer),68 in selected binary solvent mixtures with DMF as reference. The Dasiglucagon syntheses were performed using an automated peptide synthesiser (CSBio CS536XT) on 5 mmol scale on Rink Amide PS resin, using DIC coupling reagent (3 + 1 equiv.) and 3 equiv. of both Oxyma and the AA derivatives. Unlike the previous small-scale syntheses on the Symphony X instrument that employed a large excess of reagents, the stoichiometry on the CSBio CS536XT instrument mimics production conditions more accurately and consequently we anticipated that a lower purity and yield might be realised. DMF and DMSO/2-Me-THF (3
:
7) gave similar resin weight gain (75% and 76% respectively), while DMSO/DOL (3
:
7) gave a somewhat lower resin weight gain (69%). In terms of peptide purity, DMF gave a slightly lower purity (53%) to what was observed on smaller scale (Table 5), while the green solvents unfortunately yielded a significantly lower purity than previously observed on smaller scale; 41% in DMSO/2-Me-THF (3
:
7) and 38% in DMSO/DOL (3
:
7) (Fig. 8). To elucidate the source of the discrepancy in purity in DMSO/2-Me-THF (3
:
7) and DMSO/DOL (3
:
7), we compared the purity profiles for the three syntheses and identified the major impurities. To ensure that the observed differences were not instrument-related the Dasiglucagon synthesis in DMSO/DOL (3
:
7) was repeated on the Symphony X at 0.45 mmol scale utilising the stoichiometry applied on the CSBio CS536XT system. As anticipated the result was repeated indicating that the difference in purity and crude yield was not related to the instrument or synthesis scale. Consequently, we believed that the root cause for the difference between the small-scale Symphony X syntheses and the medium-scale CSBio CS536XT syntheses was the difference in the amounts of reagents used (in total 4/8/4 vs. 3/4/3 equiv. of AA derivative/DIC/Oxyma respectively), which suggested that the problem could be attributed to a few challenging coupling reactions. In-depth analysis of the purity profile indeed identified the main impurities as a number of deletion sequences related to incomplete incorporation of e.g. [Arg18], [Glu20/21] and [Asp15]. To verify that such deletions can be limited by optimisation of selected couplings, we repeated the synthesis of Dasiglucagon on the Symphony X using 3/4/3 equiv. of AA derivative/DIC/Oxyma in DMSO/DOL (3
:
7) with double couplings of [His1], [Asp15], [Arg18], and [Glu20]. As expected, using double couplings for the indicated amino acids led to an increase in the observed purity for Dasiglucagon from 38% to 46%, which was more on par with the purity obtained in DMF.
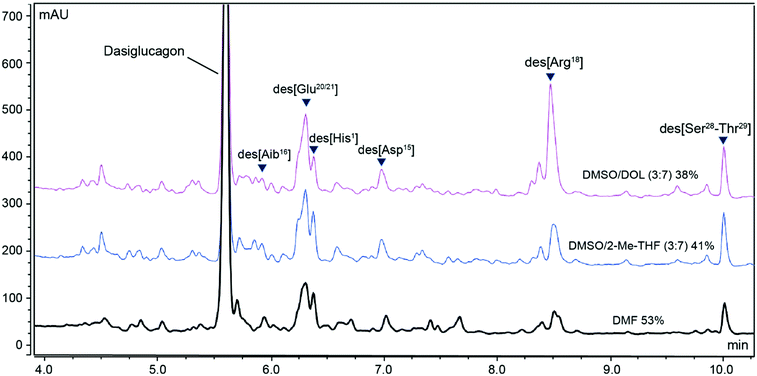 |
| Fig. 8 HPLC purity profiles (210 nm) of Dasiglucagon amide (H-HSQGTFTSDYSKYLD-Aib-ARAEEFVKWLEST-NH2) synthesised in selected green binary solvent mixtures and DMF. Major impurities arising from deletion sequences are high-lighted and the overall crude peptide purity (%) is indicated. | |
In addition to Dasiglucagon, the 20-mer peptide Bivalirudin (H-fPRPGGGGNGDFEEIPEEYL-OH) was synthesized on 7.5 mmol scale using an automated synthesiser (Sonata) in DMSO/DOL (3
:
7) and DMSO/2-Me-THF (3
:
7). As references, we chose to include not only DMF but also NBP/DOL (4
:
6) and NFM/DOL (2
:
8). These two solvent mixtures were selected as negative controls since both display polarity–viscosity profiles that we argue are detrimental in SPPS. Both mixtures lie outside the preferred polarity range (with polarities similar to each other), while NFM/DOL (2
:
8) has a similar viscosity to that of DMF, and NBP/DOL (4
:
6) a significantly higher viscosity (ESI Table S2†). In order to gain further insight about the scope and limitations of the approach, the synthesis was carried out on preloaded H-Leu-2-CTR using an equimolar AA derivative/DIC/Oxyma coupling protocol with only two equivalents of reagents compared to resin loading, which was lower than in previously described syntheses. After each coupling step, a capping step was performed routinely to facilitate the identification of difficult coupling steps. The crude purities of Bivalirudin were highest in DMSO/DOL (3
:
7) and DMF and slightly lower in DMSO/2-Me-THF (3
:
7) (Fig. 9). As expected, the NFM/DOL (2
:
8) and NBP/DOL (4
:
6) control experiments gave purities well below the other solvents, thus strengthening the hypothesis that the solvent polarity and viscosity profile is indicative of its performance in SPPS. The SPPS yield (based on resin weight gain) was comparable (≥86%) in both DMF and DMSO/DOL (3
:
7), whereas DMSO/2-Me-THF (3
:
7) resulted in a lower resin weight gain (approximately 73%). To gain further insight into the performance of the binary solvent mixtures we made an in-depth comparison of the purity and impurity profiles of Bivalirudin from the selected solvents and DMF (Table 6). Of the identified impurities, a truncated fragment (Ac-[Glu17-Leu20]-OH) formed early during the synthesis in DMSO/2-Me-THF (3
:
7) likely explains the lower SPPS yield and purity in this solvent, while a longer truncated fragment (Ac-[Pro4-Leu20]-OH) constituted the most significant impurity in DMSO/DOL (3
:
7) (Table 6). Based on the analysis from the Bivalirudin syntheses it is evident that the choice of solvent affects the purity profile of the designated target peptide. While truncated and deletion sequences can be minimised with optimisation of challenging couplings and Fmoc-removal (vide supra), further investigation into the prevalence of SPPS-specific side-reactions such as Arg-lactamisation, diketopiperazine (DKP) and aspartimide formation is warranted to further establish the scope and limitations for binary solvent mixtures in SPPS (see accompanying manuscript).69
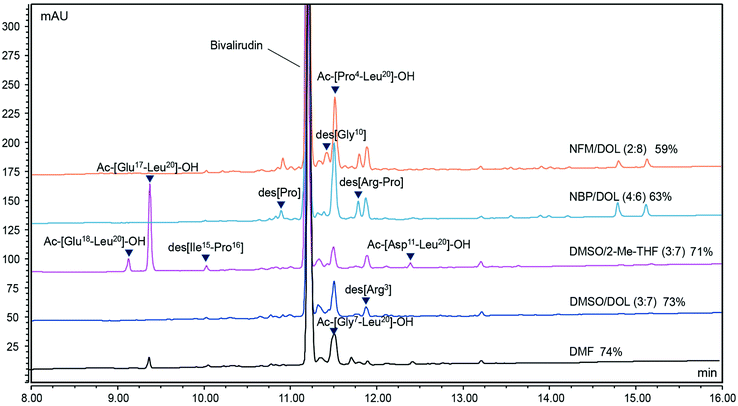 |
| Fig. 9 HPLC purity profiles (220 nm) of Bivalirudin (H-fPRPGGGGNGDFEEIPEEYL-OH) synthesised in selected green binary solvent mixtures and DMF. The crude peptide purity (%) in each solvent is indicated. | |
Table 6 Impurity profiles (LC-MS) of Bivalirudin synthesised in selected green binary solvent mixtures, benchmarked against DMF
|
Peptide prevalencea (%) |
Peptide description |
DMF |
DMSO/DOL (3 : 7) |
DMSO/2-Me-THF (3 : 7) |
NBP/DOL (4 : 6) |
NFM/DOL (2 : 8) |
The peptide prevalence and sample composition was determined by AUC using LC-MS analysis (220 nm).
Yield based on resin weight gain.
|
Bivalirudin crude purity (%) |
73.8 |
72.7 |
70.7 |
62.7 |
58.6 |
Ac-[Pro4-Leu20]-OH |
5.1 |
6.3 |
4.0 |
12.7 |
11.5 |
Ac-[Gly7-Leu20]-OH |
2.9 |
0 |
0 |
0 |
0 |
Ac-[Glu17-Leu20]-OH |
1.1 |
0 |
8.3 |
0 |
0 |
Ac-[Glu18-Leu20]-OH |
0 |
0 |
1.35 |
0 |
0 |
Ac-[Asp11-Leu20]-OH |
0.6 |
0.3 |
0.6 |
0.4 |
0.2 |
Ac-[Asn9-Leu20]-OH |
1.3 |
0.4 |
0.5 |
0.5 |
0.8 |
des[Gly–Gly] |
2.4 |
3.11 |
2.3 |
1.6 |
2.1 |
des[Gly10] |
0 |
1.01 |
0.6 |
1.3 |
3.5 |
des[Arg3] |
0.7 |
1.7 |
2.0 |
3.3 |
3.5 |
des[Arg-Pro] |
0.7 |
0.5 |
0.5 |
2.5 |
2.3 |
des[Ile15-Pro16] |
0.4 |
0.3 |
0.5 |
0.3 |
0.3 |
Other species |
10.9 |
13.7 |
8.7 |
14.5 |
17.4 |
SPPS yieldb (%) |
89 |
86 |
73 |
92 |
88 |
Conclusion
Over the past five years there has been a marked increase in the number of reports describing efforts towards replacing hazardous solvents such as DMF in SPPS. Identifying an alternative to DMF may seem like a straightforward task at a first glance, yet for a replacement to be viable and widely adopted across academic research institutions and the pharmaceutical industry there are a number of technical, regulatory and commercial challenges that need to be overcome. The SPPS process entails a range of individual steps i.e. reagent dissolution and stability, resin swelling, peptide coupling and Fmoc-removal that all contribute to the overall success of the synthesis. Thus, the physicochemical profile of the solvent needs to meet a set of technical requirements in order to be useful in SPPS, and here DMF strikes an excellent balance that has made it the gold standard. In this study we set out to identify environmentally benign solvents suitable for large-scale SPPS of peptide therapeutics at ambient temperature by assessing reagent solubility, resin swelling, amino acid coupling and Fmoc-removal efficiency in a collection of REACH compatible neat solvents and selected binary solvent mixtures. While the high utility of DMF in SPPS has been attributed to its optimal polarity, we acknowledged that solvent viscosity also plays an important role as it directly impacts the resin swelling, especially early on in the synthesis before the influence on the resin swelling by the peptide chain grows more pronounced. Revealingly, an assessment of solvent polarity and viscosity, as well as swelling and swelling response characteristics of starting- and peptidyl resins clearly showed that only binary solvent mixtures were able to match the properties of DMF, thereby being highly relevant for SPPS. Furthermore, it was possible to modify the overall character of the solvent by changing the ratio of the binary mixture. Consequently, for coupling (promoted by non-polar solvents) and Fmoc-removal reactions (promoted by polar solvents) the use of binary solvent mixtures allowed for fine-tuning of the solvent properties in a way otherwise impossible to achieve with neat solvents. Viscosity and polarity are together therefore key physicochemical parameters that can be used to predict the utility of new binary solvent mixtures in SPPS. The viscosity and polarity of DMF and a wide range of green binary solvent mixtures were used to guide the synthesis of the short model peptides Ac-LVAYAG-NH2 and Aib-enkephalin. In this way, the preferred physicochemical space for SPPS could be identified and ultimately two binary mixtures, DMSO/DOL (3
:
7) and DMSO/2-Me-THF (3
:
7) generally performed well compared to DMF for the synthesis of challenging model peptides such as the Jung-Redemann peptide (10-mer) and Dasiglucagon amide (29-mer). The sequence dependence observed with regards to the peptide purities obtained in different solvents could potentially mean longer development times for peptide API synthesis, but also offers opportunities for using solvent selection as part of that development process. In addition, solvent recycling represents an important aspect of developing greener chemical processes, which has not been addressed in the present study. Solvent recycling in the manufacture of APIs is associated with technical and regulatory challenges, making the use of recycled solvents for API synthesis cumbersome. However, the feasibility and cost-efficiency for recycling components of binary solvent mixtures in SPPS of peptides has previously been demonstrated.40 The results obtained herein demonstrate that binary solvent mixtures already constitute an important resource in peptide chemistry, further underlined by the successful automated SPPS of the peptide Dasiglucagon amide on 5 mmol scale and the peptide therapeutic Bivalirudin on 7.5 mmol scale. Further work placing emphasis on varying the composition of the binary mixtures to control common side-reactions is presented in an accompanying report,69 and taken together this work shows that binary solvent mixtures are indeed viable alternatives to DMF for the synthesis of therapeutically relevant peptides, paving the way towards greener peptide synthesis.
Conflicts of interest
The authors declare no conflicts of interest.
Acknowledgements
We thank Dr Sebastian Brandes, Dr Per Vedsø and Dr Rune Severinsen from Novo Nordisk for providing the necessary resources and support to realise the work presented herein. Dr Rolf H. Taaning, Dr Søren Bertelsen, Dr Brian Rasmussen and Dr Michael Raunkjær from Novo Nordisk are gratefully acknowledged for fruitful discussion during the start-up of the project that helped set the scope and direction. Marcela Castro is thanked for assistance with the design and creation of the graphical abstract. Dr Vincent Martin was funded by the Novo Nordisk R&D STAR Fellowship Programme. We acknowledge Dr Stefan Eissler from Bachem AG for diligently reviewing the manuscript internally. We thank and acknowledge the members of the research committee of Bachem AG for providing all necessary resources to accomplish the work presented here. Finally, we want to thank the unknown reviewers for their time reviewing this manuscript.
References
- A. Henninot, J. C. Collins and J. M. Nuss, J. Med. Chem., 2018, 61, 1382–1414 CrossRef CAS PubMed.
- J. L. Lau and M. K. Dunn, Bioorg. Med. Chem., 2018, 26, 2700–2707 CrossRef CAS PubMed.
- K. Fosgerau and T. Hoffmann, Drug Discovery Today, 2015, 20, 122–128 CrossRef CAS PubMed.
- D. J. Drucker, Nat. Rev. Drug Discovery, 2020, 19, 277–289 CrossRef CAS PubMed.
- R. B. Merrifield, J. Am. Chem. Soc., 1963, 85, 2149–2154 CrossRef CAS.
- A. A. Zompra, A. S. Galanis, O. Werbitzky and F. Albericio, Future Med. Chem., 2009, 1, 361–377 CrossRef CAS PubMed.
- T. Bruckdorfer, O. Marder and F. Albericio, Curr. Pharm. Biotechnol., 2004, 5, 29–43 CAS.
- A. Isidro-Llobet, M. Alvarez and F. Albericio, Chem. Rev., 2009, 109, 2455–2504 CrossRef CAS.
- D. M. M. Jaradat, Amino Acids, 2018, 50, 39–68 CrossRef CAS PubMed.
- M. E. Kopach, Curr. Opin. Green Sustainable Chem., 2018, 11, 54–57 CrossRef.
- J. Lopez, S. Pletscher, A. Aemissegger, C. Bucher and F. Gallou, Org. Process Res. Dev., 2018, 22, 494–503 CrossRef CAS.
- B. L. Bray, Nat. Rev. Drug Discovery, 2003, 2, 587–593 CrossRef CAS PubMed.
-
The European Chemicals Agency (ECHA), Substance Infocard for N,N-dimethylformamide (DMF): https://echa.europa.eu/de/substance-information/-/substanceinfo/100.000.617.
-
The European Chemicals Agency (ECHA), Substance Infocard for N-methylpyrrolidone (NMP): https://echa.europa.eu/de/substance-information/-/substanceinfo/100.011.662.
-
The European Chemicals Agency (ECHA), Substance Infocard for Dichloromethane (DCM): https://echa.europa.eu/substance-information/-/substanceinfo/100.000.763.
-
The European Chemicals Agency (ECHA), Candidate List of substances of very high concern for Authorisation. http://echa.europa.eu/candidate-list-table.
- L. Bergkamp and N. Herbatschek, Rev. Eur. Comp. Int. Environ. Law, 2014, 23, 221–245 CrossRef.
- D. Prat, O. Pardigon, H.-W. Flemming, S. Letestu, V. Ducandas, P. Isnard, E. Guntrum, T. Senac, S. Ruisseau, P. Cruciani and P. Hosek, Org. Process Res. Dev., 2013, 17, 1517–1525 CrossRef CAS.
- D. Prat, A. Wells, J. Hayler, H. Sneddon, C. R. McElroy, S. Abou-Shehada and P. J. Dunn, Green Chem., 2016, 18, 288–296 RSC.
- C. M. Alder, J. D. Hayler, R. K. Henderson, A. M. Redman, L. Shukla, L. E. Shuster and H. F. Sneddon, Green Chem., 2016, 18, 3879–3890 RSC.
-
The European Chemicals Agency (ECHA), Registration, Evaluation, Authorisation and Restriction of Chemicals (REACH), https://echa.europa.eu/legislation.
- F. Albericio, O. Al Musaimi and B. G. de la Torre, Green Chem., 2020, 22, 996–1018 RSC.
- Y. E. Jad, A. Kumar, A. El-Faham, B. G. de la Torre and F. Albericio, ACS Sustainable Chem. Eng., 2019, 7, 3671–3683 CrossRef CAS.
- A. Isidro-Llobet, M. N. Kenworthy, S. Mukherjee, M. E. Kopach, K. Wegner, F. Gallou, A. G. Smith and F. Roschangar, J. Org. Chem., 2019, 84, 4615–4628 CrossRef CAS PubMed.
- V. Martin, P. H. G. Egelund, H. Johansson, S. Thordal Le Quement, F. Wojcik and D. S. Pedersen, RSC Adv., 2020, 10, 42457–42492 RSC.
- Y. E. Jad, G. A. Acosta, S. N. Khattab, B. G. de la Torre, T. Govender, H. G. Kruger, A. El-Faham and F. Albericio, Org. Biomol. Chem., 2015, 13, 2393–2398 RSC.
- J. Pawlas, B. Antonic, M. Lundqvist, T. Svensson, J. Finnman and J. H. Rasmussen, Green Chem., 2019, 21, 2594–2600 RSC.
- O. Al Musaimi, Y. E. Jad, A. Kumar, A. El-Faham, J. M. Collins, A. Basso, B. G. de la Torre and F. Albericio, Org. Process Res. Dev., 2018, 22, 1809–1816 CrossRef CAS.
- Y. E. Jad, G. A. Acosta, T. Govender, H. G. Kruger, A. El-Faham, B. G. de la Torre and F. Albericio, ACS Sustainable Chem. Eng., 2016, 4, 6809–6814 CrossRef CAS.
- Y. E. Jad, G. A. Acosta, S. N. Khattab, B. G. de la Torre, T. Govender, H. G. Kruger, A. El-Faham and F. Albericio, Amino Acids, 2016, 48, 419–426 CrossRef CAS PubMed.
- K. Wegner, D. Barnes, K. Manzor, A. Jardine and D. Moran, Green Chem. Lett. Rev., 2021, 14, 153–164 CrossRef CAS.
- B. G. de la Torre, A. Kumar, M. Alhassan, C. Bucher, F. Albericio and J. Lopez, Green Chem., 2020, 22, 3162–3169 RSC.
- O. Al Musaimi, A. El-Faham, A. Basso, B. G. de la Torre and F. Albericio, Tetrahedron Lett., 2019, 60, 151058 CrossRef CAS.
- A. Kumar, Y. E. Jad, A. El-Faham, B. G. de la Torre and F. Albericio, Tetrahedron Lett., 2017, 58, 2986–2988 CrossRef CAS.
- A. Kumar, A. Sharma, B. G. de la Torre and F. Albericio, Molecules, 2019, 24, 4004 CrossRef CAS PubMed.
- A. Kumar, A. Thompson-Adewumi, K. P. Nandhini, J. M. Collins, F. Albericio and B. G. de la Torre, Org. Process Res. Dev., 2019, 23, 1096–1100 CrossRef CAS.
- A. Kumar, Y. E. Jad, J. M. Collins, F. Albericio and B. G. de la Torre, ACS Sustainable Chem. Eng., 2018, 6, 8034–8039 CrossRef CAS.
- L. Ferrazzano, D. Corbisiero, G. Martelli, A. Tolomelli, A. Viola, A. Ricci and W. Cabri, ACS Sustainable Chem. Eng., 2019, 7, 12867–12877 CrossRef CAS.
- Y. Ran, F. Byrne, I. D. V. Ingram and M. North, Chem. – Eur. J., 2019, 25, 4951–4964 CrossRef CAS PubMed.
- J. Pawlas and J. H. Rasmussen, Green Chem., 2019, 21, 5990–5998 RSC.
- Y. Deguchi, M. Kono, Y. Koizumi, Y.-i. Izato and A. Miyake, Org. Process Res. Dev., 2020, 24, 1614–1620 CrossRef CAS.
-
E. R. Lax and T. Shah, in Peptide Therapeutics: Strategy and Tactics for Chemistry, Manufacturing, and Controls, The Royal Society of Chemistry, 2019, pp. 151–193 Search PubMed.
- The Public Activities Coordination Tool, https://echa.europa.eu/pact.
- SciFinder – Chemical Abstracts Service (American Chemical Society), https://scifinder.cas.org.
-
Reaxys, (Elsevier), http://www.reaxys.com Search PubMed.
-
The European Chemicals Agency (ECHA), Substance Infocard for 1,3-dioxolane (DOL): https://echa.europa.eu/substance-information/-/substanceinfo/100.010.422.
- J. B. Sperry, C. J. Minteer, J. Y. Tao, R. Johnson, R. Duzguner, M. Hawksworth, S. Oke, P. F. Richardson, R. Barnhart, D. R. Bill, R. A. Giusto and J. D. Weaver, Org. Process Res. Dev., 2018, 22, 1262–1275 CrossRef CAS.
- R. Subiros-Funosas, R. Prohens, R. Barbas, A. El-Faham and F. Albericio, Chem. – Eur. J., 2009, 15, 9394–9403 CrossRef CAS PubMed.
- K. D. Wehrstedt, P. A. Wandrey and D. Heitkamp, J. Hazard. Mater., 2005, 126, 1–7 CrossRef CAS PubMed.
- D. Samson, D. Rentsch, M. Minuth, T. Meier and G. Loidl, J. Pept. Sci., 2019, 25, e3193 CrossRef PubMed.
- J. K. Magtaan, M. Devocelle and F. Kelleher, J. Pept. Sci., 2019, 25, e3139 CrossRef PubMed.
- K. C. Pugh, E. J. York and J. M. Stewart, Int. J. Pept. Protein Res., 1992, 40, 208–213 CrossRef CAS PubMed.
- M. Delgado and K. D. Janda, Curr. Org. Chem., 2002, 6, 1031–1043 CrossRef CAS.
- C. Amadi-Kamalu, H. Clarke, M. McRobie, J. Mortimer, M. North, Y. Ran, A. Routledge, D. Sibbald, M. Tickias, K. Tse and H. Willway, ChemistryOpen, 2020, 9, 431–441 CrossRef CAS PubMed.
- S. Lawrenson, M. North, F. Peigneguy and A. Routledge, Green Chem., 2017, 19, 952–962 RSC.
- Y. E. Jad, T. Govender, H. G. Kruger, A. El-Faham, B. G. de la Torre and F. Albericio, Org. Process Res. Dev., 2017, 21, 365–369 CrossRef CAS.
- J. K. Magtaan, M. Devocelle and F. Kelleher, J. Pept. Sci., 2020, 26, e3250 CrossRef CAS.
- G. B. Fields and C. G. Fields, J. Am. Chem. Soc., 1991, 113, 4202–4207 CrossRef CAS.
- A. R. Vaino, D. B. Goodin and K. D. Janda, J. Comb. Chem., 2000, 2, 330–336 CrossRef CAS PubMed.
- I. L. Rodionov, I. A. Peshenko, L. K. Baidakova and V. T. Ivanov, Int. J. Pept. Res. Ther., 2007, 13, 161–171 CrossRef CAS.
- R. Santini, M. C. Griffith and M. Qi, Tetrahedron Lett., 1998, 39, 8951–8954 CrossRef CAS.
- K. Dimroth, C. Reichardt, T. Siepmann and F. Bohlmann, Justus Liebigs Ann. Chem., 1963, 661, 1–37 CrossRef CAS.
- C. Reichardt, Chem. Rev., 1994, 94, 2319–2358 CrossRef CAS.
- M. Paradis-Bas, J. Tulla-Puche and F. Albericio, Chem. Soc. Rev., 2016, 45, 631–654 RSC.
- L. A. Carpino, E. Krause, C. D. Sferdean, M. Schümann, H. Fabian, M. Bienert and M. Beyermann, Tetrahedron Lett., 2004, 45, 7519–7523 CrossRef CAS.
-
T. Redemann and G. Jung, in Peptides 1996 - Proceedings of the 24th European Peptide Symposium, ed. R. Ramage and R. Epton, Mayflower Scientific Ltd, Kingswinford, UK, 1998, pp. 749–750 Search PubMed.
- U. Hövelmann, B. V. Bysted, U. Mouritzen, F. Macchi, D. Lamers, B. Kronshage, D. V. Møller and T. Heise, Diabetes Care, 2018, 41, 531 CrossRef PubMed.
- T. E. Warkentin, A. Greinacher and A. Koster, Thromb. Haemostasis, 2008, 99, 830–839 CrossRef CAS PubMed.
- S. Jadhav, V. Martin, P. H. G. Egelund, H. Johansson Castro, T. Krüger, F. Richner, S. Thordal Le Quement, F. Albericio, F. Dettner, C. Lechner, R. Schönleber and D. S. Pedersen, Green Chem., 2021 10.1039/d1gc00604e.
Footnote |
† Electronic supplementary information (ESI) available. See DOI: 10.1039/d1gc00603g |
|
This journal is © The Royal Society of Chemistry 2021 |
Click here to see how this site uses Cookies. View our privacy policy here.