DOI:
10.1039/D0LC00963F
(Perspective)
Lab Chip, 2021,
21, 9-21
Accelerating innovation and commercialization through standardization of microfluidic-based medical devices
Received
23rd September 2020
, Accepted 20th November 2020
First published on 23rd November 2020
Abstract
Worldwide, the microfluidics industry has grown steadily over the last 5 years, with the market for microfluidic medical devices experiencing a compound growth rate of 22%. The number of submissions of microfluidic-based devices to regulatory agencies such as the U.S. Food & Drug Administration (FDA) has also steadily increased, creating a strong demand for the development of consistent and accessible tools for evaluating microfluidics-based devices. The microfluidics community has been slow, or even reluctant, to adopt standards and guidelines, which are needed for harmonization and for assisting academia, researchers, designers, and industry across all stages of product development. Appropriate assessments of device performance also remain a bottleneck for microfluidic devices. Standards reside at the core of mature supply chains generating economies of scale and forging a consistent pathway to match stakeholder expectations, thus creating a foundation for successful commercialization. This article provides a unique perspective on the need for the development of standards specific to the emerging biomedical field of microfluidics. Our aim is to facilitate innovation by encouraging the microfluidics community to work together to help bridge knowledge gaps and improve efficiency in getting high-quality microfluidic medical devices to market faster. We start by acknowledging the progress that has been made in various areas over the past decade. We then describe the existing gaps in the standardization of flow control, interconnections, component integration, manufacturing, assembly, packaging, reliability, performance of microfluidic elements and safety testing of microfluidic devices throughout the entire product life cycle.
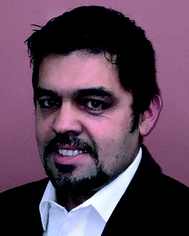 Darwin Reyes | Dr. Darwin Reyes is a biomedical engineer and project leader at the National Institute of Standards and Technology (NIST). He received a B.S. and Ph.D. in chemistry from the University of Puerto Rico, and an M.S. in Applied Biomedical Engineering from The Johns Hopkins University. After his PhD he worked with Prof. Andreas Manz at Imperial College as an NSF Postdoctoral Fellow. Dr. Reyes is currently working on the development of microfluidic systems with electronic manipulation and measurement elements for cell-based assays and microphysiological systems. He is also developing electronic flow sensors and protocols that could ultimately serve as standard methods for localized flow in microfluidic systems. |
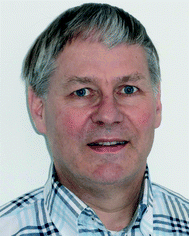 Henne van Heeren | Henne van Heeren is an industry analyst in the field of microfluidics and CEO of enabling MNT. Henne assisted several startups in the areas of technology commercialisation and industrialisation of micro and nanotechnologies. He has been active in microfluidics standardisation since 2010. |
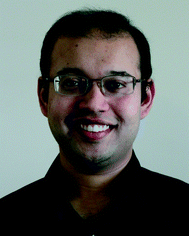 Suvajyoti Guha | Dr. Suvajyoti Guha has been working at the Center for Devices and Radiological Health (CDRH), U.S. Food and Drug Administration (US FDA) since 2012. He graduated from University of Maryland, College Park, with a PhD degree in Mechanical Engineering. His interests include microfluidics, aerosol sciences, pediatrics and laboratory research driven by patient input. He also is the team lead for the recently created Microfluidics Program within CDRH's Office of Science Engineering Laboratories. In that role his goal is to foster more collaboration with external organizations to facilitate innovation in microfluidics based medical devices. |
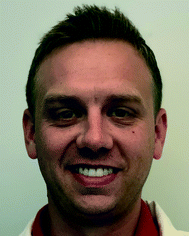 Luke Herbertson | Dr. Luke Herbertson has been a biomedical engineer in FDA's Center for Devices and Radiological Health since 2009, during which time he has directed in vitro fluid dynamics studies, developed standard test methods, and reviewed pre-market submissions of medical devices. He received his Ph.D. from the Pennsylvania State University for his work in hemodynamics and artificial heart valves. Dr. Herbertson has expertise in mechanical blood cell damage, blood–surface interactions, pressure-flow characterization, and pump performance, and he is the co-leader of the Microfluidics Program within the Office of Science and Engineering Laboratories. |
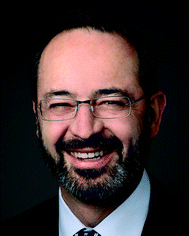 Alexios Paul Tzannis | Dr. Alexios Paul Tzannis has been working for IMT Microtechnologies for 14 years and is responsible for developing the Life Science and Diagnostics activities of IMT. He studied Electrical Engineer M.Sc. at the technical University of Denmark (DTU) in the field of technical Holography. He received his Ph.D. at the ETH Zurich for his work at the Paul Scherrer Institute in the field of resonant holographic Interferometry. Dr. Alexios Paul Tzannis has founded, hands-on experience in micro- and nanotechnology, MEMS, optics and optoelectronics, spectroscopy, and microfluidics. |
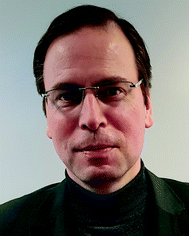 Jens Ducrée | Dr. Jens Ducrée is a full professor of microsystems at Dublin City University. His main research interests revolve about microfluidic Lab-on-a-Chip technologies for applications in the context of the life sciences. His team specifically looks into design-for-manufacture and virtual prototyping technologies in order to increase the reliability, performance and integration density of sample-to-answer automated solutions for decentralised bioanalytical testing. Dr. Ducrée also looks into upgrading science, research and technology development in academia and the corporate sector by efficiently tapping into the collective intelligence, skills and infrastructure of the global research community through the game-changing distributed ledger technology blockchain. |
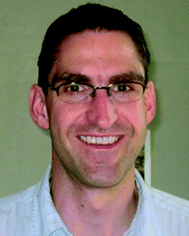 Hugo Bissig | Dr. Hugo Bissig has been working since 2007 at METAS in the laboratory of flow and hydrometry as a scientist. He is an expert in the development and construction of primary standards for measuring low liquid flow rates and is project leader of European research projects. He obtained his MSc in physics at the University of Fribourg in Switzerland and continued his education with a PhD in soft condensed matter physics at the same institution. |
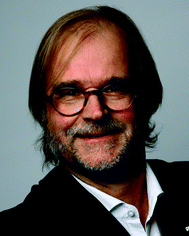 Holger Becker | Dr. Holger Becker, FRSC, is co-founder and Chief Scientific Officer of microfluidic ChipShop GmbH. After his PhD in Physics from University of Heidelberg on Surface-acoustic wave (SAW) sensors he worked as postdoc for Andreas Manz at Imperial College. He started his industrial career with Jenoptik Mikrotechnik in 1998. In 2002 he co-founded microfluidic ChipShop GmbH. Since then, he has been active in the field of polymer-based microfluidics, microfluidics fabrication and commercialization. He has served as co-chair of MicroTAS 2013 and on the Advisory Boards of the “Next Generation Diagnostics” and “Molecular Diagnostics Europe” conferences, and of “Lab-on-a-Chip”, and in the Editorial Boards of “Microelectronic Engineering” and “Micro and Nanosystems”. He has published more than 160 journal and conference papers with currently >6000 citations. |
Introduction
“There is plenty of room at the bottom” – it has been just over 60 years since physicist and visionary Richard Feynman articulated this outlook, foreseeing the importance of miniaturization. Since then, advances in microfabrication, especially in microelectronics, have led to seminal innovations such as computing chips embedded in smartphones and tablets. Miniaturized systems that typically handle fluids in the range of micro- to nanoliter volumes, which are referred to as microfluidic or lab-on-chip technologies, began to follow suit at first in the academic arena, and now through commercial applications. The academic work started in the early 1990's,1,2 and in the 30 years since, the microfluidics field has seen an ever-diversifying number of applications in healthcare—namely point-of-care diagnostics, genomics and drug development, as well as in various other life science disciplines. As the technology continues to mature, more companies invested in a wide range of applications are becoming part of the movement.
Other past emerging technologies, such as microelectronics, have successfully adopted standards that have enabled the growth of their respective industries. Although there have been recent attempts to promote the development of standards for microfluidics, we are only now starting to see the payoff of those efforts.3 The concept of standardization is seen by some in the field as a way to stifle innovation, but the opposite effect usually occurs. Standards enable clear communication between customers and suppliers, while providing reliable, harmonized, and validated measurement protocols. They improve and streamline manufacturing processes, reducing the cost of components. Enhancements to the network of processes could produce many microfluidic components and standard protocols that will allow researchers in both academia and industry to focus on scientific and technological advancements, saving valuable time and resources required for troubleshooting issues with the devices. Although the field of microfluidics is unique from others due to an especially wide variety of potential applications, there are certain technological aspects that should be considered for standardization.
One major aspect that has hindered growth in microfluidics is the diversity in the materials and fabrication processes, which are heavily dependent on whether the product is made in a research setting or in a mass production facility for commercialization. While academicians tend to be motivated by understanding the science and procuring research grants, many professors and research associates envision that their best ideas and inventions will someday be commercialized, perhaps through a spin-off company or by patent licensing. However, many proof-of-concept experiments in academia utilize materials that tend to be useful only for small scale device production. In addition to deciphering what material should be used to fabricate a new product, other challenges regarding commercialization need to be solved to determine the product viability. The following general questions should always be considered before embarking on product development: (1) what is the potential market value of the assay? (2) Is it a single point measurement or a multiplexed assay? (3) What is the public health need of this assay and what problem does it help to solve? (4) What outputs do the customers want and need? (5) Is the product a disruptive alternative to an existing solution? (6) What is the benefit to the customer or why would they switch to a new product? (7) What are the volumes of sample and consumable required, and what are the recurring costs, sale price, and long-term profit margins?
Some of these questions are strictly related to the development of a business plan for a product or company and outside the scope of this perspective, whereas other questions, such as (2), (4) and (7) are important to stakeholders with interest in streamlining their quality assurance process through standards and guidelines.
Once the commercialization path and the consumables have been defined, the next step involves establishing a production path, which has its own set of challenges. During this stage, the key assay workflow parameters are refined and implemented to create the most efficient production scenario. Fundamentally, the transduction mechanism of the assay dictates the design of the microfluidic device. One major challenge relevant to biomedical in vitro diagnostic applications and the life sciences is that a chip carrying the sample and reagents is often hard to clean and is typically designed to be disposable, which needs to meet stringent cost targets. In many cases, this economic constraint requires eventual mass production of the product by tool-based polymer replication schemes such as injection moulding, hot embossing, or film-based roll-to-roll. These well-established industrial replication processes often involve high upfront costs for process development and instrumentation.4 These costs are sometimes only recovered by achieving high production numbers, which extend well beyond the typical needs during product development and early-stage in-field testing. To accommodate frequent design improvement iterations, the development of single-use microfluidic chips is mostly based on prototyping and small-scale fabrication methods such as ultra-precision milling, lithography-derived patterning, or 3D-printing. This means that the scale-up of production is marked by a series of technological chasms with respect to the choice of materials as well as their structures, processing and assembly techniques. Each of these modifications may have an unintended and detrimental impact on the performance (e.g., fluidic and bioanalytical) and reliability of the device. The time and resources required to change from the materials and processes used during the prototyping period to scale up and be able to mass produce devices tend to be largely underestimated. For example, companies have provided anecdotal evidence that the transition from the materials and processes used for the proof-of-concept design to larger-scale production of the end-product can take several years. Others in the literature have referred to the development and optimization of non-scalable devices as unproductive due to the variety of artifacts that could be found in the different materials.5
The role and benefits of standards and guidelines
The challenges described in the previous section can be mitigated through development of standards in microfluidics. The topic of standardization in the field of microfluidics is rarely broached. This article provides a perspective on how to bridge some of the gaps that are currently hindering the potential growth of microfluidics and microfluidic-based technologies for healthcare purposes. While the discussion here pertains only to medical devices, it may apply to other areas that use microfluidic technologies as well. Furthermore, in the context of this article, we should make the distinction that we do not address product standards, which have been described as specified technical requirements to be fulfilled by a design.6 Instead, we are focusing on testing strategies that would aid product developers in advancing the field of microfluidics. The following are five major benefits of standards and guidelines:
1. Harmonization – harmonization avoids redundancy and conflicts between standard testing strategies developed independently by individual research and development labs. Overall, harmonization is relied heavily upon by industry and regulatory agencies for product evaluation, providing an invaluable tool to further product development and, ultimately, approval. The use of standards can help provide additional information about the device in the pre-clinical stage through cost-efficient bench testing or simulations, which can potentially reduce the need for, or supplement, animal studies and clinical data.
2. Safety and performance – standard test methods enable a direct comparison of safety and performance between similar products or established safety thresholds. They are often simple so as not to require specialized, costly equipment to conduct.
3. Early stage product development – standards help researchers in the early stage of product development better prepare for, and take into consideration, the entire product life cycle through a defined pathway to commercialization.
4. Development of a supply chain – standards for materials, manufacturing, and testing promote the establishment of industrial supply chains, which in turn, reduce the costs for everyone from the basic researcher to the manufacturer of the end product.
5. Acceleration of newcomers into the field – standards allow “non-expert” users to utilize microfluidic components as tools without the need to become proficient in understanding the mechanisms behind the system.
Why now?
A lack of standards can hinder product development. For instance, if there is no standard test method for a small business to follow, they may not have the resources to develop and validate their own test methods and are less likely to fulfil regulatory requirements. In this case, regulatory bodies like the U.S. Food and Drug Administration (FDA) can work with the company on an individual basis to develop a device-specific test plan. These alternatives are not only inefficient in terms of cost, resources, and the processes used, they also present challenges for the manufacturers and regulatory reviewers in interpreting the test results. The need for consistent, well-defined and streamlined approaches for evaluating similar device types is now becoming apparent in microfluidics-based medical devices with increased commercialization of microfluidics-based devices (Fig. 1) and in submissions to regulatory agencies such as the FDA (Fig. 2A). Diagnostics presently constitute most of microfluidic device submissions (Fig. 2B). While the market for microfluidic-based biomedical platforms continues to grow steadily, and several disruptive applications have been developed for point-of-care diagnostics and for initially gauging the efficacy of new drugs, little progress has been made to date in standardizing common aspects (e.g., flow and interconnections) of these microfluidic-based technologies. Thus, we feel that this an optimal time for the microfluidics community to embrace the standardization of common aspects of microfluidic devices. Such test methods will help to drive the momentum behind commercializing this emerging field and will make the submission process more consistent to regulatory agencies including the FDA and the European Medicines Agency, EMA.
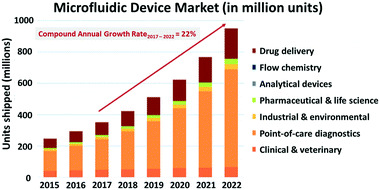 |
| Fig. 1 Representative plot showing the steady growth of the microfluidic devices market by the different application areas (shown with permission from Yole Développement). | |
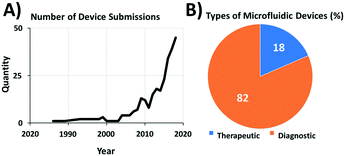 |
| Fig. 2 (A) Recent growth of medical device submissions per year to FDA that use microfluidic technologies. (B) Breakdown of percentages of therapeutic and diagnostic applications for these devices. | |
The following section summarizes the progress made in the last decade in microfluidics. Critical areas where standardization will provide clear benefits have been identified based on real-world feedback from stakeholders.
A preamble to standardization: current state of microfluidics
The need for standardization has long been recognized. The community has identified potential areas where standardization would be most beneficial to include interfaces or interconnections, materials for fluidic sealing and the corresponding bonding methods, materials present in the flow path, and achievable pressure considerations. These knowledge gaps formed the basis for creating the microfluidic standardization initiative by the SEMI (Semiconductor Equipment and Materials International) organization. Although four standards were published addressing high-pitch microfluidic interconnections,7 their impact remained limited because they were created with limited involvement from the microfluidics community. Additional basic guidelines that do not contain detailed specifications have also been published. Tantra et al.6 discussed alternative pathways for disseminating consensus approaches such as publicly available specifications and international working agreements. While they are not considered standard documents, they have advantages in that they can be published quickly and have the potential to reach a wider audience. To further develop and promote microfluidics standards, a non-profit organization has been founded8 and an ISO task force, ISO/CD 22916 Laboratory equipment — Interoperability of microfluidic devices9 has been formed. Others have expanded the initiative to similar technical fields such as for organ-on-chip technologies.10 Most of the standardization efforts to date have been to address reliability in the context of failure modes. Here we define a failure mode as the inability to perform as specified by the intended use of the device, leading to adverse events that may result in device failure or cause harm to a patient. Adverse events can either be (a) soft, or parametric, causing the system to shift from a desired set point, or (b) hard, or catastrophic, causing a change in the system's structure or topology or even total system failure.11 Catastrophic adverse events may cause incorrect results, device stoppage, or even patient death, whereas soft faults may be reversible depending on the fault mechanism, sensors, and mitigation strategies. Investigating and understanding failure modes is essential to improving devices and advancing the field through redesigned systems, material changes, and process modifications. Failure modes can be design- or fabrication-related (e.g., residual stress), operation-related (e.g., wear, creep) and environment-related (e.g., particles, vibration, temperature changes).12 The following subsections describe efforts that have been made to lay the groundwork for creating standardized methods and materials for advancing the use of microfluidics in biomedical applications.
Materials
Klapperich13 stated that one of the major issues with biomedical diagnostics has been a lack of standardization in material selection, process development, and tool design. There is an obvious disconnect between the popular materials and methods used in product development that offer flexibility in design and prototyping and those preferred by industry [e.g., COC – cyclic olefin copolymer, PMMA – poly(methyl methacrylate), polystyrene, glass] that are more suited for mass production processes like injection moulding or wafer level manufacturing. This is generally seen as a problem during scale up as device performance can be strongly influenced by the materials used. Unfortunately, little progress has been made in this area, except that academic researchers have access to new additive manufacturing tools, such as 3D-printing, that have the potential to bridge the gap between prototyping and mass production. Paper-based microfluidic devices have found application in point-of-care, environmental and food safety applications. Their primary biosensor detection scheme is based on capillary forces for the transport of the analyte, a detection scheme based on a catalytic cycle and a colorimetric detection. The mechanisms associated with paper-based microfluidics are different than the ones described in this paper and therefore paper-based microfluidic devices can be defined as a separate class of microfluidic devices.14,15
Common failure modes with materials include cracking and delamination. Thermally induced cracks (in glass) have been studied by Shim,16 and those results demonstrated detrimental outcomes close to joints at different pressures, and defects in manufacturing in various areas of the devices. In general, to check for the presence of cracks and delamination, one could follow MIL-STD-883C, a standard containing uniform methods, controls, and procedures for testing microelectronic devices suitable for use within military and aerospace electronic systems. However, the circumstances under which microfluidic devices might be stored, shipped, and used, can be quite different from conventional electronic devices. As cracks and delamination often lead to fluid leakage, leakage testing may be able to capture some aspects of this failure mode.
Chemical resistance and biocompatibility
As most microfluidic devices employ non-corrosive solutions at or around room temperature, chemical resistance is only of interest for a subset of biomedical applications. One exception is when high concentrations of organic solvents come in contact with certain polymer devices in micro reaction technology applications. Wilhelm17 proposed to test chemical resistance by flowing different solvents at a constant flow rate over a period of 500 minutes. This protocol has not been widely accepted by the community primarily because most microfluidic devices are intended to be used for shorter periods of time, and thus the utility of such an approach has not been well accepted. The ISO standard that describes the effects of product immersion in liquid chemicals18 would not seem to apply to most microfluidic devices. Conversely, the ISO 10993 standard on biocompatibility testing of medical devices should be applicable for most blood-contacting medical devices regardless of their size.19 The biological evaluation of all components in contact with the fluid in a biomedical microfluidic system can be conducted using principles established in ISO 10993 without the need to modify those well-established test methods.
Pumps and valves
Moving parts introduce their own specific failure modes, especially when there are fluid-contacting surfaces, as is the case for valves. Araci20 studied microfluidic biochips featuring microvalves based on a flexible membrane (control layer) on top of a microfluidic chip (flow layer) and identified the following general defects:
1. Blockage: blocked, disconnected or missing channels.
2. Leakage: defective areas on the channel wall can compromise independent microchannels.
3. Misalignment: flow and control layers are misaligned.
4. Degradation of valves: loss of flexibility or perforation of the membrane over time.
5. Dimensional errors: a mismatch of height-to-width ratio which may lead to a valve that does not function properly.
These defects were described in terms of leakage or blockage with versions of fault-tolerant architectures containing extra channels, valves, and other microfluidic elements for redundancy, simplicity, and statistical power. However, such redundancy is not always practical or accurate, and therefore the community is reluctant to embrace this approach.
Passive microfluidic devices
Flow rate is a critical measurement of a microfluidic system performance, but changes to flow can also indicate issues with the system like formation of bubbles or any other system failure (e.g., small leakage, fouling, blockage). There are protocols for ensuring the accurate measurement of flow,21–23 but these protocols are based on estimating the average flow rate through the system over time. These methods were developed to accurately measure steady flow rates down to a resolution of 5 nL min−1. However, the metrology institutes did not previously focus on accurate measurement methods for dynamically changing flows or step response times. Therefore, there are no current widely accepted methods for accurately measuring real-time fluctuations or delayed step responses. However, several projects are currently underway to address these real-time flow measurement issues.24–26
Electrodes
Electrodes are unique since they can, in principle, be self-tested. Lui et al.27 embedded a test option to track and correct for drifts in electronic measurements (e.g., impedance). Another effort from the same research group,28 proposed two options for assessing the degradation of electrodes in contact with fluidic or biological systems. One was a low frequency, impedance-based protocol to determine electrode structural degradation supported by physical measurements from electrode arrays used in drug testing (on cells); and the other was a mid-frequency oscillation test based on changes in the capacitance between a bio-fluid and the electrodes to determine contamination, degradation and fouling of the electrodes. However, no formal work towards the development of standard methods for electrical/electrochemical measurements for microfluidic applications has been undertaken. Work for developing standard methods will need to consider typical faults for electrodes, which include cracking or delamination from the substrate, degraded gate voltages, jammed particles in the channel, and leakage.29 Other failure modes that could negatively influence electrode performance include trapped bubbles, foreign particles or precipitation of particles during dilution or other mixing steps, bubbles caused by leakage or electrolysis, manufacturing and design tolerances, and operation outside the normal limits (i.e., a divergence between user requirements and the technical limitations of the design).
Modularity and interconnections
There are three approaches to creating microfluidic systems: 1) full integration of microfluidic functions, 2) creating a microfluidic system by assembling of components, and 3) connecting the different microfluidic subsystems with tubing. With regards to the last option, much work has been done in the area of interconnections. Becker et al.30 were the first to stress the use of standardized or universal interconnections for developing microfluidic devices. They stated that connections to the existing laboratory equipment like pipetting robots and handling stations could be a key aspect to the implementation and integration of new microfluidic devices. Therefore, they proposed the use of microtiter plate and microscope slide formats. Such an approach was expected to save on engineering costs and shorten device times to market. Klapperich13 also stated that there is a case for interconnection standards and dimensional standards to facilitate integration of microfluidic functions. Since then, several independent researchers in the field have mentioned the need for microfluidic connector standards. Becker,31 for instance, discussed the interconnections in more detail in 2010 and recommended mini-Luer interfaces as a potential solution, though he also pointed out certain disadvantages to using mini-Luers such as the large dead volumes. As an alternative, he proposed Nanoports or similar connections. Unfortunately, standard initiatives like Becker's never expanded beyond one or two companies. Van Heeren32 reemphasized the benefits of “plug and play” functionality in microfluidics enabled by standard multiport connectors. Modularization by direct assembly of microfluidic components was seen by Podczerviensky, et al. as a key for industrial manufacturing of microfluidic systems.33,34 One of the advantages of such a modular approach is the possibility of testing these modules in isolation on the bench with standard test protocols. The results can be used to quantify variations in the production process and improve product quality. However, the approach needs simple, robust and reliable connections, preferably with plug-and-play functionality, a feature that is not yet available. An alternative to the plug-and-play option could be a consensus, standardized port pitch and device dimensions that could be applicable for designing modular microfluidic systems (e.g., organ-on-chip applications).
Fast design of microfluidic components has been developed by the Cidar lab at Boston University using predefined modular components.35 From this group's efforts a 3DμF has been created as the first completely open source interactive microfluidic system designer that readily supports state-of-the-art design automation algorithms.36
Pressurization limit/burst pressure in modularity and interconnections
Christensen37 created microfluidic interconnections in a PDMS device by sticking a hollow needle through the PDMS. He tested these interconnections extensively with regards to burst pressure and flow restrictions in relation to needle twisting, connecting and reconnecting, and bonding methods. Thus, a framework was developed for producing and testing interconnections in PDMS.
Stepped increases in pressure have been suggested for leakage tests and for testing the reliability of interconnections.38 Paydar39 assumed that leakage of connectors occurs when the hydrodynamic pressure on the sidewall of the gasket overcomes the friction force between the connector parts and proposed two leakage tests:
1. Increasing the fluid pressure until a leak forms; repeating it several times with new devices to establish the expected burst pressure of the device.
2. Pressurizing the devices over an extended period of time at 2/3 of the expected burst pressure.
Gray40 also developed a fluid dynamic testing protocol to quantify the pressure that interconnections can withstand before leaking and to monitor the pressure drop versus flow rate through the device using a syringe pump and a pressure sensor.
Thermal cycling in modularity and interconnections
Simko41 stated that a major factor that affects the reliability of interconnections and seals is thermal cycling, as it impacts the durability of the plastic and elastomer sealing materials. The protocol Simko proposed for leak testing involved increasing the temperature over 30 minutes to 121 °C, and then maintaining that temperature for another 30 minutes. The device was then water quenched to room temperature and the cycle was repeated 250 times. The system dimensions were checked, and leaks were observed 17 times during those cycles. This procedure cannot be widely used because many polymer materials have working temperatures below the peak temperature of 121 °C. Furthermore, for any device containing biological materials, including antibodies and protein coatings, this approach will not work because of degradation of the biological components at elevated temperatures. If such a test is prescribed to a device that contains materials with different thermal expansion coefficients (e.g., a silicon sensor in a polymer part), the thermal exposure could lead to device failure due to dimensional changes and induced shear stress.
Pull out forces in modularity and interconnections
Pull out force is an excellent measure of how well the interconnections hold two chips together and a good indicator of fluidic pressurization limits. When a connection is based on a rigid material and tight fittings, it is extremely sensitive to tolerances, resulting in a wide range in pull-out forces for a variation of even a few microns. When fabricated using more compliant materials like PDMS, the devices are less sensitive in this respect. Interconnection strengths are measured by a linear coil actuator force tester to quantify and compare pull-out forces. This might be an alternative for pressure testing of devices. To simulate the effect of other forces on the connector, in-plane pull tests or pulling at an angle to the plane can also be used.42 Despite the many efforts to characterize the strength of the interconnections, the adoption of any one approach has largely been limited.
Long-term reagent storage in modularity and interconnections
One recurring reliability problem when storing devices involves the loss of medium over the shelf life of the product. As blisters are used for reagent storage in other life sciences areas, the microfluidics community may be able to rely on existing knowledge of blister packets to drive this area of standardization. Liquid storage reservoirs, like blisters, can be tested easily for reagent loss after storing for a prolonged time at elevated temperatures.42
Delamination studies, which examine material coming off fluid-contacting surfaces of the reservoir where a reagent was stored, were conducted by the company Schott in accordance with USP166043 using two different types of formulations: a 15% potassium chloride solution and a 10% sodium thiosulfate solution led to product recalls due to delamination. Established buffers or formulations that are used to develop drugs (e.g., ultra-pure water, citrate buffer, phosphate buffer, sodium bicarbonate buffer and EDTA [ethylenediaminetetraacetic acid]) were also tested.44 However, the relevance of this work for microfluidics applications remains largely undefined and specific to the application.
Areas benefitting from standards development
From review of the available literature above, it is evident that modularity and interconnectivity are the areas that have received considerable attention to date. Unfortunately, the standardization efforts so far have failed to demonstrate a cross-cutting influence in microfluidics. One of the reasons is that the facilitators did not enlist a wider group of organizations during the development of the respective standard test protocols. In the past, in other fields, we have seen an informal standard test protocol becoming widely adopted when a dominant innovator in the field supported the effort. Because there are many potential applications for microfluidic technologies, there is no dominant person, organization, or even device type to produce a big enough following to compel others to take up the standard. The most promising way forward is to bring together organizations in the beginning of the development process of a standard and work closely to ensure consensus from the initial steps of the process. This will allow for input from everyone to be considered and ensure that the requirements of different stakeholders are addressed for widespread use and applicability. This also means that the discussions should be open for all interested parties and the results be made freely and publicly available. Therefore, a series of workshops were organized to identify the key areas in microfluidics that would benefit most from standards development.38 Guidelines addressing different aspects of microfluidic standardization were offered to the community in the form of white papers.8
From 2014 to 2016, five surveys were disseminated among microfluidic experts to formulate a consensus strategy. In total, over 1000 responses from about 200 different organizations were received. Most of the responses were from companies, but research institutes and universities were also represented. The conclusions from the surveys are summarized as follows:
1. Reliability and ease of use are the main concerns for those designing and using microfluidic interconnections.
2. There are four major sources of reliability issues pertaining to microfluidic devices: (i) flow control, (ii) components like micropumps, (iii) microfluidic interconnections, and (iv) filters.
3. In terms of failure modes, flow-related issues like bubble formation, unanticipated changes in flow and pressure, and clogging were the most common problems encountered by the community.
4. There is a need for flow measurement accuracies in the range of 2% to 5%, which will require a closed-loop control to achieve very accurate flow measurements. The lack of availability of accurate and affordable flow sensors was therefore identified as a major limitation for the microfluidics community.
5. It is also important to monitor changes in flow rate and system responses to such changes.
6. Most users prefer to work with pressures below 2 bars and temperatures below 50 °C to reduce failure modes.
7. Integration of components is also a major challenge with respect to designing and manufacturing sensor systems.
8. The diversity of sensors, and the fact that they are often application-specific, makes it challenging to formulate industry-wide standard tests for sensors.
9. Concerns were raised on how to test the reliability of microfluidic connections, as well as the ability to test micropumps and microvalves, accurately.
Guided by the results from these surveys, a proposal was presented to the International Organization for Standardization (ISO) to start a working group with the objective of making microfluidic devices “plug-and-play” (i.e., simplified, more reliable, and cheaper to assemble). As shown in Table 1, a proposal for microfluidic interconnection standards and for operational classes based on temperature and pressure was accepted at an ISO organized workshop in 2016.3 By understanding past issues with microfluidic-based devices and listening to the concerns of stakeholders from the microfluidics community, we can begin to formulate a plan for standardization of microfluidics in the identified areas of need.
Key areas for future standards development
Based on feedback from the community during the microfluidics standards workshops, the following factors have been identified as key areas for standards or guidelines: generic testing protocols, interconnections & inter-compatibility, integration/modular approach, and flow control. These issues are discussed in more detail in this section.
Standardized common testing methods
Before any product is allowed on the market, it is imperative that the product is functional and safe. It should be verified that the product meets or exceeds the reliability and quality requirements of its intended application and exhibits features and characteristics that are within the specified tolerance range.45 This means that there is a need for practical reliability tests to compare competing products, but also to check for variations between products and to prevent faulty products from being made available to the public. Different aspects of a microfluidic device can be tested as a final, comprehensive unit or as individual components. On the lowest level are the material properties that are used to create the product. Examples of those properties are mechanical strength and optical transmission. On a second level, there are straightforward physical parameters like dimensions and wafer bond strength. To measure such characteristics, a plethora of instruments and protocols are available (e.g., optical profilometry, infrared transmission microscopy, double cantilever beam testing, micro chevron testing, bond testing). On the highest level are application specific tests. The protocols and instruments for such tests are often specific to the product or manufacturer. Among these different types of tests, the focus should be on those tests that have the potential to be applicable to a wide range of products. There are common, non-trivial issues plaguing the microfluidic community and some are related to specific components that can be tested individually (e.g., leakage testing). Such tests are particularly suited for microfluidic components, but they may also be applied to integrated microfluidic products. The goal is to ensure manufacturing quality and reliability of the products. A consistent approach to testing such components would help the community to overcome these problems.45 As Volpatti46 states, academics seldom cite reproducibility statistics and chip-to-chip and batch-to-batch variability for their proposed fabrication methods, since only a handful of working devices are typically produced. This is unfortunate since nearly all the publicly available technical information about microfluidics stems from academic research, and only a few commercial organizations make such information public. Standard test protocols may trigger publications about microfluidic quality issues from which industry and academia can learn and adapt.
Solutions for defining efficient non-destructive production test strategies, fault tolerant design features and integrated health monitoring are expected to be important contributions to facilitate new technologies.29 One industry that has substantial experience with this issue is the semiconductor industry, though similarities between the emerging microfluidics industry and the established semiconductor industry are practically non-existent.30 Most of the tools and techniques currently used for failure analysis are leveraged from the semiconductor industry and are not designed to be used with fluids.47 The microfluidics community faces the challenge of needing to define its own testing strategies, methods and reliability models. In general, simulations and fault predictions become increasingly complex when more domains or modules are added to the system. However, heterogenous systems need more research to assess the fault modes, as they are the most difficult cases of failure analysis.11 Challenges associated with defining tests to identify fault modes are difficult to address in microfluidic devices/systems.12 Some of these challenges include:
a. Size: the physics of materials are not always understood on these small scales, and surface-to-volume ratios are much higher compared to other products. Surface properties play an important role. Due to the small device sizes, large variations in temperature are not expected to play an important role, especially when most biomedical applications typically occur well below 100 °C.
b. Metrology: while many tools exist to measure surface profiles or other geometrical features of open structures at high resolution (e.g., the profile of a microfluidic channel), very limited techniques exist which can do the same with enclosed structures (e.g., microchannels in the device after bonding) and in a production line.48
c. Multiple domains: microfluidic, thermal, optical, electrical, etc.
d. Diversity of applications.
e. Diversity of media.
f. Surface interactions: the influence of the surface of the channels on the behaviour of the device.
g. Difficulty in parameterizing.
In electronics there are a limited number of building blocks or components (e.g., transistors, resistors) and each can be parametrized, facilitating design improvements while circumventing the need for trial and error. The number of different building blocks in microfluidics is much higher, and opportunities for parameterizing them are limited. While the performance can often be parameterized for components such as mixers, this is rather difficult for valves and pumps.30
According to van Heeren,32 generic reliability tests for microfluidic devices should be based on a classification scheme that includes meaningful temperature and pressure ranges. Such a classification scheme has been refined during discussions with stakeholders and is in the process of being converted into an official standard document.3 This scheme is presented in the following table (Table 1):
Table 1 Initial classification scheme for microfluidic devices; PT denotes pressure and temperature, respectively
Class type |
Maximum pressure (bar) |
Maximum temperature (°C) |
Minimum temperature (°C) |
PT 2/50 |
2 |
50 |
4 |
PT 2/75 |
2 |
75 |
4 |
PT 2/100 |
2 |
100 |
4 |
PT 7/50 |
7 |
50 |
4 |
PT 7/100 |
7 |
100 |
4 |
PT 30/50 |
30 |
50 |
4 |
Further classification might be needed based on parameters such as gases versus liquids, digital or continuous flow, viscosity, corrosive liquids, dynamic versus static fluid-contacting surfaces, and reusable or single use devices. Furthermore, there is a need for community-wide test protocols due to the many issues presented in Table 2.
Table 2 Types of testing that may be applicable for microfluidic-based medical devices
Type of testing |
Component or device feature |
Reliability / validation of components |
- Blisters, vials, reservoirs |
- Chip holders |
- Flow distributors |
- Flow sensors |
- Heat exchangers |
- Pumps |
- Valves |
- Electrodes |
- Optical sensors |
Characterization of flow pathway |
- Geometrical features |
- Bonding and assembly |
- Coatings |
- Fluidic or pneumatic tightness |
- Warpage |
- Surface roughness |
- Physicochemical properties |
Device processing |
- Accelerated aging |
- Storage |
-Transport and logistics |
- Manual handling |
- Operation |
Environmental |
- Atmospheric pressure |
- Local temperature |
- Humidity |
- Electromagnetic fields |
- Vibration |
- Shock |
Overall, there is a need for a microfluidic equivalent of MIL-STD-883C,48,49 which would entail uniform methods, controls, and procedures for testing microfluidic devices under conditions and constrains that are typical for the intended biomedical applications. A guideline for early development design and process failure mode and effects analysis (PFMEA) dedicated to microfluidics has not yet been created. There is a lack of scientific articles addressing the sensitivity of flow measurements, manufacturing processes, failure analyses, and root cause analyses to better understand reasons for failures in microfluidic medical devices.
Interconnections and inter-compatibility
Inter-compatibility involves the interfacing of different components within a device. The main reliability issue with microfluidic interconnections is device leakage. Testing for leakage with gases as a medium is described in detail in DIN EN 1779:199950 and is also discussed extensively by Schröder.51 Leak testing with gas is an efficient way of testing and is generally non-destructive. In microfluidics, however, leakage of liquids is more likely to be a concern, and liquids are not within the scope of this standard. There are already well established and commonly used consensus standardized protocols for leakage testing, including with liquids, for blood-contacting medical devices such as oxygenators,52 transfusion equipment53 and hemodialysis.54 While it may sound superfluous with so many test protocols in existence for other emerging technology domains, it is important to realize that driving liquid through a device with micro-scale dimensions can potentially introduce unique failure modes. There is a need for leakage test protocols specific to microfluidic products to address common issues anticipated in modular microsystems with interconnections and non-trivial geometries, subjected to potentially elevated pressures and dynamic fluid properties.
Leakage tests are generally conducted using either a syringe pump or a pressurized reservoir. When using a gas, leaks can be visualized by submerging the product under pressure in a fluid and then checking for bubbles or for pressure loss in the closed system. Liquid leakage can also be detected by adding a dye to the liquid. When submerged, the leak can be optically detected in real time. Since leaks can occur slowly and worsen over time, it is recommended to test for a longer period and/or increase the pressure stepwise beyond the worst-case operating point of the device.
Modularity and assembly
One of the advantages of a modular approach is the possibility of testing individual modules in isolation with standard test protocols. The results can be used to quantify variations in the production process and improve product quality. However, the approach needs simple, robust and reliable connections, preferably with plug-and-play functionality. To address an expanded view of module-to-module and module-to-world connectors, there is the need to consider the smallest achievable dimensions and resolutions, based on parameters such as manufacturability, reliability, and robustness. Furthermore, to enable a platform approach towards manufacturing and to support the device evaluation process, there is a need for a geometrically parameterized library of standard, inter-compatible components and subsystems for quick configurability.
One challenge with modularization is the issue of combining optics, electronics, and other components in industrial settings, particularly when stringent acceptance criteria are applied for high volume manufacturing, reliability and cost effectiveness. Since there are many microfluidic devices that require a combination of electrical and optical connections, there is a true need for a standard that would address this issue. For optical and electrical connections, existing standards can be used as a starting point. For instance, electronic packaging is highly mature and is already standardized by international standards associations such as IPC (Institute of Printed Circuits), ITRS (International Technology Roadmap for Semiconductors) and JEDEC (Joint Electron Device Engineering Council).
To be able to mass produce these complex systems in a cost-effective manner, the development of fluidic components using standardized formats is essential. Requirements should ensure: 1) leak-free microfluidic connections; 2) well defined microfluidic paths with negligible dead volumes, but with sufficient lengths to ensure laminar flows; 3) all linked materials in the fluid paths have to be compatible; and 4) the need to combine microfluidic, electrical and optical functionalities into a single system makes the microfluidic platform potentially more complex than an isolated electronic package.
Flow control
At the heart of any microfluidic system is its capability of moving small amounts of fluids in a controlled fashion. However, measuring these small volume flow rates in an accurate way is challenging. International metrology institutes have started to define methodologies for accurate measurement of flow within the range of 100 nL min−1 to 1 mL min−1.22,55,56 However, measuring flow rates below 100 nL min−1 remains a challenge, which is why several European groups as well as NIST have internally funded projects to address this issue by building up new metrological infrastructures.24,57
Other challenges remain regarding definitions, methods, and instrument calibrations for ensuring extreme tolerance levels. Therefore, standardized protocols, as well as the ability of a system to detect and induce dynamic flow changes, are needed by the microfluidics community. These tests would enable an objective, reproducible evaluation of flow control components (e.g., pumps, valves, tubing) along with stand-alone devices in real time. The target uncertainties of these metrological infrastructures should be determined by the measurement precision required by the microfluidic industry.
Some measurement methods already exist for application specific needs.23,56,58 Besides flow rates, other flow related characteristics like pressure drop across a chip or the maximal operational pressure in flow should also be characterized. New testing methods including measurement of the dead volume and piping volumes should be developed to satisfy accuracy requirements of the industry.
We believe that these common issues with microfluidics-based platforms can be collaboratively resolved by the community to facilitate the development of standard test methods with widespread applicability. The community can build on the existing individual efforts described in this paper to establish consensus standards that will benefit all stakeholders.
Summary and outlook
Since the emergence of the first microfluidic device in the form of a gas chromatograph in the 1970's,59 the field of microfluidics has grown and diversified by leaps and bounds. While initially used almost exclusively in academic research, microfluidics remained on the fringes of commercialization for decades. The diversity in sensing, actuation, materials, and fabrication in lab-on-chip applications has made the commercial expansion and growth of microfluidics quite challenging. However, the field appears to now be maturing, as shown by recent market and regulatory submissions trends. Multiple biomedical areas such as inertial microfluidics, droplet microfluidics and organs-on-chip look promising. One of the technologies that is revolutionizing the biological sciences is massive parallel sequencing—specifically next generation sequencing (NGS). Its success is based on full implementation of microfluidic solutions from sample preparation through sequencing itself. NGS has evolved into a multibillion-dollar market, and it is projected to reach $7.7B in sequencing consumables revenue by 2024.60
Standardization of microfluidics offers many benefits to academic researchers, regulatory bodies, and industry. For academicians, increased knowledge of validated procedures and materials suitable for high-volume production informs research, resulting in a faster transition from bench to mass production in industry. Further, there is an increasing need for researchers to show funding organizations that proof-of-principle products will have a sustainable future by indicating the number and type of tests required. Perhaps most importantly, reproducibility is a major concern in biomedical research,61 underscoring the importance of standards in academia and for all other stakeholders.
The benefits in standardization for regulatory agencies is three-fold: 1) standardization can streamline the regulatory decision-making process; 2) regulatory considerations can be understood by all stakeholders earlier in the product lifecycle through the pre-submission process; and 3) interested stakeholders can collaboratively participate in interlaboratory studies to advance standard test methods for the field. Standardization in the field of microfluidics is beneficial to industry in that it helps to make the development process more reliable, thus decreasing development time and manufacturing costs. Finally, standards help supply chains to generate economies of scale. International collaborations involving academia, industry, national metrology institutes, and regulatory agencies are necessary to develop comprehensive and meaningful standards with maximum benefits for all. There are many unknowns about the reliability of microfluidic devices and their unique failure modes. This lack of knowledge is a serious hurdle for the field of microfluidics, inhibiting further growth and development.
Microfluidics standardization is not just a matter for industry; it can also help researchers developing new concepts faster and with higher success rates. Also, industry needs researchers to identify and understand potential failure modes, as they are often related to fundamental problems of translating the assay into a functional microfluidic component.
The microfluidics community should join forces to improve the reliability of microfluidic devices and help to advance the utility of microfluidics for biomedical applications. We encourage organizing workshops to identify and develop consensus standards, along with industry-wide surveys on this topic and round robin interlaboratory testing. Standards should cater towards potential failure modes, and researchers should consider the impact of material and fabrication choices earlier in the product development life cycle. Whenever possible, existing standards from other well-established fields should be used in or adapted to the field of microfluidics. We hope this article will facilitate more activities in microfluidics standards development and will stimulate collaborations around this common goal.
Conflicts of interest
The authors have no conflicts to declare.
Acknowledgements
We acknowledge the many experts who answered the surveys and participated in the standardization workshops. We also acknowledge Dr. Rucha Natu for assisting in mining the FDA/CDRH internal database for microfluidics related medical device submissions. DRR acknowledges NIST-on-a-Chip Initiative funding.
References
- P. A. Auroux, D. Iossifidis, D. R. Reyes and A. Manz, Anal. Chem., 2002, 74, 2637–2652 CrossRef CAS.
- D. R. Reyes, D. Iossifidis, P. A. Auroux and A. Manz, Anal. Chem., 2002, 74, 2623–2636 CrossRef CAS.
-
ISO, International Standard ISO/IWA 23:2016 - Interoperability of microfluidic devices — Guidelines for pitch spacing dimensions and initial device classification, 2016 Search PubMed.
- J. Ducree, Micromachines, 2019, 10, 886 CrossRef.
- C. D. Chin, V. Linder and S. K. Sia, Lab Chip, 2012, 12, 2118–2134 RSC.
- R. Tantra, H. van Heeren and J. Jarman, J. Micro/Nanolithogr., MEMS, MOEMS, 2016, 15, 4 Search PubMed.
-
SEMI MS11 - Specification for Microfluidic Port and Pitch Dimensions, SEMI MS7 - Specification for Microfluidic Interfaces to Electronic Device Packages, SEMI MS6 - Guide for Design and Materials for Interfacing Microfluidic Systems and SEMI MS9 - Specification for High Density Permanent Connections Between Microfluidic Devices, https://store-us.semi.org/products/ms01100-semi-ms11-specification-for-microfluidic-port-and-pitch-dimensions?_pos=1&_sid=4f2648dbe&_ss=r, (accessed April 20, 2020) Search PubMed.
-
www.microfluidics-association.org
.
-
ISO, ISO/CD 22916 - Laboratory equipment — Interoperability of microfluidic devices, https://www.iso.org/standard/74157.html, (accessed April 20, 2020) Search PubMed.
- M. Mastrangeli, S. Millet, J. van den Eijnden-van Raaij, A. Puech, B. van Meer, N. Franzen and O. Partners, ALTEX, 2019, 36, 650–668 CrossRef.
-
T. O. Myers, Doctor of Philosophy, University of Hull, 2010 Search PubMed.
-
J. R. Alcock and U. M. Attia, in 2nd International through-Life Engineering Services Conference, ed. R. Roy, A. Tiwari, A. Shaw, C. Bell and P. Phillips, Elsevier Science Bv, Amsterdam, 2013, vol. 11, pp. 272–277 Search PubMed.
- C. M. Klapperich, Expert Rev. Med. Devices, 2009, 6, 211–213 CrossRef.
- A. W. Martinez, S. T. Phillips, M. J. Butte and G. M. Whitesides, Angew. Chem., Int. Ed., 2007, 46, 1318–1320 CrossRef CAS.
- M. M. Gong and D. Sinton, Chem. Rev., 2017, 117, 8447–8480 CrossRef CAS.
- D. J. Shim, H. W. Sun, S. T. Vengallatore and S. M. Spearing, J. Microelectromech. Syst., 2006, 15, 246–258 CrossRef CAS.
- E. Wilhelm, C. Neumann, T. Duttenhofer, L. Pires and B. E. Rapp, Lab Chip, 2013, 13, 4343–4351 RSC.
-
ISO, Plastics — Methods of test for the determination of the effects of immersion in liquid chemicals, 2010 Search PubMed.
-
ISO, ISO 10993-4:2017 - Biological evaluation of medical devices — Part 4: Selection of tests for interactions with blood, 2017 Search PubMed.
-
I. E. Araci, P. Pop and K. Chakrabarty, Microfluidic Very Large-Scale Integration for Biochips: Technology, Testing and Fault-Tolerant Design, IEEE, New York, 2015 Search PubMed.
- M. Ahrens, S. Klein, B. Nestler and C. Damiani, Meas. Sci. Technol., 2014, 25, 9 CrossRef.
-
J. D. Wright and J. W. Schmidt, presented in part at the 18th International Flow Measurement Conference (FLOMEKO 2019), Lisbon, Portugal, June 26–28, 2019 Search PubMed.
- G. A. Cooksey, P. N. Patrone, J. R. Hands, S. E. Meek and A. J. Kearsley, Anal. Chem., 2019, 91, 10713–10722 CrossRef CAS.
- E. Batista, A. Furtado, J. Pereira, M. Ferreira, H. Bissig, E. Graham, A. Niemann, A. Timmerman, J. A. E. Sousa, F. Ogheard and A. W. Boudaoud, Flow Meas. Instrum., 2020, 72, 5 CrossRef.
- H. Bissig, M. Tschannen and M. de Huu, Flow Meas. Instrum., 2020, 73, 7 Search PubMed.
- P. Salipante, S. D. Hudson, J. W. Schmidt and J. D. Wright, Exp. Fluids, 2017, 58, 10 CrossRef.
-
H. Liu, A. Richardson, T. G. Harvey, T. Ryan and C. Pickering, Embedded Test & Health Monitoring Strategies for Bio-Fluidic Microystems, IEEE, New York, 2008 Search PubMed.
- Q. Al-Gayem, H. Y. Liu, A. Richardson and N. Burd, Journal of Electronic Testing - Theory and Applications, 2011, 27, 57–68 CrossRef.
-
Q. Al-Gayem, H. Liu, A. Richardson and N. Burd, in ETS 2009: European Test Symposium, Proceedings, IEEE Computer Soc, Los Alamitos, 2009, pp. 73–78 Search PubMed.
- H. Becker, Lab Chip, 2010, 10, 1894–1897 RSC.
-
H. Becker and C. Gärtner, Proceedings COMS – Commercialization of Micro and Nano-Systems Conference, Baden-Baden, 2005 Search PubMed.
- H. van Heeren, Lab Chip, 2012, 12, 1022–1025 RSC.
- K. C. Bhargava, R. Ermagan, B. Thompson, A. Friedman and N. Malmstadt, Micromachines, 2017, 8, 9 CrossRef.
-
J. Podczerviensky and L. Levine, Modularity in the Design and Volume Production of Microfluidic Devices, CRC Press-Taylor & Francis Group, Boca Raton, 2011 Search PubMed.
-
D. Densmore, http://cidarlab.org/microfluidics.
- R. Sanka, J. Lippai, D. Samarasekera, S. Nemsick and D. Densmore, Sci. Rep., 2019, 9, 10 CrossRef.
- A. M. Christensen, D. A. Chang-Yen and B. K. Gale, J. Micromech. Microeng., 2005, 15, 928–934 CrossRef.
- D. R. Reyes and H. van Heeren, J. Res. Natl. Inst. Stand. Technol., 2019, 124, 1–22 CrossRef.
- O. H. Paydar, C. N. Paredes, Y. Hwang, J. Paz, N. B. Shah and R. N. Candler, Sens. Actuators, A, 2014, 205, 199–203 CrossRef CAS.
-
B. L. Gray, S. Jaffer, D. G. Sahota and S. M. Westwood, Mechanical and Fluidic Characterization of Microfluidic Interconnects for Lab-on-a-Chip Applications, IEEE, New York, 2008 Search PubMed.
-
D. Simko, Pharmaceutical Manufacturing, 2008 Search PubMed.
- A. Pfreundt, K. B. Andersen, M. Dimaki and W. E. Svendsen, J. Micromech. Microeng., 2015, 25, 10 CrossRef.
-
https://www.uspnf.com
.
- U. Rothaar, M. Klause and B. Hladik, PDA J. Pharm. Sci. Technol., 2016, 11/12, 560–567 CrossRef.
- R. Tantra and H. van Heeren, Lab Chip, 2013, 13, 2199–2201 RSC.
- L. R. Volpatti and A. K. Yetisen, Trends Biotechnol., 2014, 32, 347–350 CrossRef CAS.
-
J. A. Walraven, in International Test Conference 2003, Proceedings, IEEE, New York, 2003, pp. 850–855 Search PubMed.
- D. R. Reyes, M. Halter and J. Hwang, J. Microsc., 2015, 259, 26–35 CrossRef CAS.
- Test Method Standard: Microcircuits, MIL-STD 883E, 1996, p. 641.
-
ISO, UNE EN 1779:2000 - Non-destructive testing - Leak testing - Criteria for method and technique selection, 2000 Search PubMed.
- G. Schroder, Insight, 2001, 43, 797–800 Search PubMed.
-
ISO, ISO 7199:2016 - Cardiovascular Implants And Artificial Organs - Blood-Gas Exchangers (Oxygenators), ISO, 2016 Search PubMed.
-
ISO, ISO 1135-4:2015 - Transfusion equipment for medical use — Part 4: Transfusion sets for single use, gravity feed, ISO, 2015 Search PubMed.
-
ISO, ISO 8638:2010 - Cardiovascular
implants and extracorporeal systems — Extracorporeal blood circuit for haemodialysers, haemodiafilters and haemofilters, ISO, 2010 Search PubMed.
- H. Bissig, H. T. Petter, P. Lucas, E. Batista, E. Filipe, N. Almeida, L. F. Ribeiro, J. Gala, R. Martins, B. Savanier, F. Ogheard, A. K. Niemann, J. Lotters and W. Sparreboom, Biomed. Tech., 2015, 60, 301–316 Search PubMed.
- M. Ahrens, B. Nestler, S. Klein, P. Lucas, H. T. Petter and C. Damiani, Biomed. Tech., 2015, 60, 337–345 Search PubMed.
-
S. Hudson, https://www.nist.gov/noac/technology/fluid-measurements/flow-and-pressure.
- F. Ogheard, P. Cassette and A. W. Boudaoud, Flow Meas. Instrum., 2020, 73, 11 CrossRef.
- S. C. Terry, J. H. Jerman and J. B. Angell, IEEE Trans. Electron Devices, 1979, 26, 1880–1886 Search PubMed.
-
S. Clerc and A. Siari, Next-Generation Sequencing & DNA Synthesis: Technology, Consumables Manufacturing and Market Trends, Yole Dèveloppement, 2019 Search PubMed.
- M. Baker, Nature, 2016, 533, 452–454 CrossRef CAS.
|
This journal is © The Royal Society of Chemistry 2021 |
Click here to see how this site uses Cookies. View our privacy policy here.