DOI:
10.1039/D0MA00732C
(Review Article)
Mater. Adv., 2021,
2, 2561-2583
Injectable hydrogels in stroke and spinal cord injury treatment: a review on hydrogel materials, cell–matrix interactions and glial involvement
Received
24th September 2020
, Accepted 4th March 2021
First published on 6th March 2021
Abstract
Central nervous system (CNS) pathologies, such as stroke and spinal cord injury, remain debilitating issues due to the inhibitory environment in the CNS. Many research works have focused on combinatorial therapeutic approaches, such as biomaterial scaffolding, cell transplantation and biomolecule delivery, in the hope of effectively improving functional recovery in vivo. Unfortunately, to date, there is still no effective treatment to regain mobility post-injury. In search of better therapeutic strategies, injectable hydrogels are becoming a popular treatment option for CNS diseases due to their tuneable mechanical properties and the minimally invasive nature of administration. Moreover, the ability to encapsulate exogenous cells and therapeutic molecules while providing an environment that is permissive to cells and promote cell survival incentivises the use of injectable hydrogels in CNS disease treatment. In this review, we will discuss the advances that have been achieved in the recent decade in injectable hydrogel systems for tissue regeneration after stroke and spinal cord injuries. In particular, we focus on the cellular response and tissue integration related to these hydrogel systems. We hope to provide useful insights on materials choices for future research work in injectable hydrogels for stroke and spinal cord regeneration.
1. Introduction
Despite advances in medicine and technology, pathologies of the central nervous system (CNS), such as stroke and spinal cord injury (SCI), remain debilitating issues globally. Stroke is one of the main causes of adult disability and the third leading cause of death worldwide.1 In the next decade, stroke will continue to increase the burden on patients and society.2 By 2030, approximately 3.4 million Americans are expected to suffer from ischemic stroke, a prevalence increment of 20.5% as compared to 2012.3 In the case of SCI, it is estimated that over 1 million people suffer from SCI in North America. The lifetime costs for treatment and care range from $1.1 to $4.7 million USD per person, which aggregates a direct cost that exceeds $7 billion per year in the United States alone.4 To date, there is still no effective treatment for CNS diseases. The inhibitory micro-environment in the CNS makes it difficult for self-regeneration to take place. Hence, in search for better therapeutic treatment options, recent studies have focused on the introduction of tissue scaffolds that mimic the extracellular matrix (ECM) to promote regeneration.5–7 Correspondingly, a variety of scaffold materials in combination with various biological agents have been developed. These may include exogenous cells, microRNAs and growth factors.8–15
Injectable hydrogels have become a popular scaffolding treatment option for CNS diseases. The tunable mechanical properties and the ability for minimally-invasive administration make injectable hydrogels a more attractive option as compared to other traditional scaffolding methods, which require surgical procedures. While there have been many reviews on injectable hydrogels for stroke and SCI treatments, most of them have focused on the design strategies, particularly the physical and chemical properties of various scaffold materials.5,6,16–20 In this review, the advances of injectable hydrogels and cell transplantation for recovery in CNS diseases will be discussed, focusing on the aspect of cellular response and tissue integration. We then assess the suitability of hydrogel materials in different stages of tissue regeneration in vitro and in vivo. We hope that this review will aid the future selection of materials and the development of injectable hydrogels for CNS disease treatment.
2. Disease pathogenesis and cellular involvement
2.1. Stroke and SCI pathology
Stroke can be divided into haemorrhagic stroke and ischemic stroke. Haemorrhagic stroke is caused by ruptured cerebral blood vessels or abnormal blood vessel structures, while ischemic stroke is a result of interruption in blood supply to the brain. Studies have shown that about 85% of stroke patients suffered from ischemic stroke.21 Thus, in this review we will mainly discuss studies related to ischemic stroke. Ischemic stroke is mainly due to the blockage of blood vessels in the brain and the inability of the body to establish reperfusion. Subsequent brain damage stems from a complex series of pathological events, such as depolarization, inflammation, and excitotoxicity. These phenomena greatly impair the stability of the blood–brain barrier and activate the release of free radicals and proteases, which not only causes local neuronal cell death, but also further expands the damage.20 Unlike the other organs and tissues, brain tissues are very sensitive to ischemia. The core of the infarct is usually immediately and irreversibly damaged and the related nerve function is immediately lost. Soon after, the core boundary expands to adjacent tissues, resulting in apoptosis and cell death to the distal end of tissues due to vascular occlusion.22
The cause of SCI is commonly due to sudden mechanical impact to the spinal cord parenchyma that results in fracture, contusion, compression or laceration of spinal cord tissue.23 Following the initial impact on spinal cord tissue, the focal destruction of neural tissue at the lesion triggers a sequence of chronological events that eventually lead to the destruction of neural tracts, also known as secondary injury. Within 15 min post-injury, multiple hemorrhages are often seen in the grey matters where re-perfusion does not usually occur within the first 24 h.24 Following the initial injury, the loss of blood supply and the lack of self-healing mechanism lead to vascular insult, hemorrhages and ischemia which ultimately result in cell death and necrotic tissues within 24–48 h post-trauma.23,25 The native microenvironment of the spinal cord inhibits its ability for self-recovery as axonal destruction results in filtration of glial cells and other non-CNS cells to clear up the debris. The debris from myelin and oligodendrocytes form the initial component of glial scar to contain further damage, followed by migration and proliferation of astrocytes that upregulate the production of glial fibrillary acidic protein (GFAP) and form the bulk of non-permissive scar.26 The clearing of debris and glial scarring form a cystic cavity at the lesion which acts as a wall to prevent axonal regrowth and neurite outgrowth.6 Over time, continuous apoptosis of oligodendrocytes within the lesion leads to further demyelination of axons and Wallerian degeneration. This further creates an environment that prevents axonal regrowth after SCI.5
Injuries in the CNS lead to tricky pathologies as self-regeneration and recovery are discouraged in the native CNS microenvironment and axonal regeneration and functional recovery does not occur actively.27 Treatments in CNS injuries have focused on restoring secondary injuries and providing structural support and extracellular cues for cell ingrowth and tissue recovery.28 The use of injectable hydrogel has become an appealing treatment option due to the capability in providing mechanical support as well as flexibility in shapes and material choices. In SCI, the lesion is further complicated by glial scars formed by reactive astrocytes, leading to further inhibitory barrier that prevents recovery. With regard to the administration approaches, hydrogels could be implanted via open-wound surgery and injection in the SCI model, but limited to the injection method in the stroke model.
2.2. The roles of glial cells in stroke and spinal cord injury treatment and the potential of cell transplantation
Glial cells in the CNS, mainly astrocytes, microglia and oligodendrocytes and their progenitors, support and enable effective nervous system function. In the healthy CNS, glia aid in the homeostasis of the microenvironment. While remaining relatively inactive in the healthy state, glial cells act to increase inflammatory actions and help modulate the environment in the event of an infection or injury. These cells play crucial roles in tissue regeneration after CNS pathology by regulating inflammation and supporting neuronal growth.29
Reactive astrocytes limit the expansion of the lesion by forming glial scar around the lesion and releasing neurotrophins through anti-excitatory toxicity, thereby providing neuroprotection.30 The views on the effects of glial scar remain controversial. Traditionally, the glial scar is believed to prevent axonal regrowth.31 However, recent studies have shown that, by preventing glial scar formation, axonal regrowth is reduced in vivo, thus suggesting the potential beneficial effects of astrocytes and glial scarring in axonal regeneration.32 Polarization of microglia can be either pro-inflammatory, which produces cytotoxic factors leading to inflammation and oligodendrocyte apoptosis by releasing pro-inflammatory cytokines, or anti-inflammatory reactions that produce trophic factors to promote axon regeneration.33,34 In the case of oligodendrocytes, despite the extensive neuronal cell death after CNS injuries, activated oligodendrocyte progenitor cells (OPCs) show significant proliferation and migration to replace lost cells. The interaction with cytokines promotes OPC differentiation into oligodendrocytes to replace dead oligodendrocytes in the lesion and support axon regrowth.35,36
Neuron cell death and glial inflammation pose a major challenge in tissue regeneration following CNS injuries. In this regard, cell transplantation has become a popular therapeutic option for the treatment of stroke and SCI due to the potential of (1) directly replacing damaged cells in the lesion, (2) providing neuroprotection to the surviving neuro connective tissues, and (3) providing a supportive cellular growth substrate for axonal regrowth.37 However, cell transplantation commonly faces major cell death, low cell migration and integration, as well as limited directional guidance of axonal growth.38,39 In addition, the potential safety risks associated with stem cell transplantation require the development of rigorous protocols to ensure cell homogeneity, quality assurance, and no tumorigenicity.40
3. The roles of injectable hydrogels in cellular response and tissue regeneration in stroke and spinal cord injury treatment
To overcome the challenges faced in tissue regeneration within the CNS, combinations of scaffold materials and cellular and molecular therapies have to be considered according to the type and severity of the injury.5,41 Injectable hydrogels are widely used to treat pathologies in both hard and soft tissues due to their resemblance to natural ECM structures and minimally-invasive administration. Some common requirements for injectable hydrogels include biocompatibility and non-cytotoxicity. Depending on the types of tissues and application, the rate of degradation, gelation time and mechanical properties of hydrogels need to be carefully considered.42 In hard tissue applications, such as bones and cartilages, the mechanical properties of hydrogels are one of the main considerations due to the weight bearing nature of these tissues.43 However, the good mechanical properties of hydrogels are difficult to achieve, particularly up to the mega pascal range, without modifications in functional groups or crosslinking which often use cytotoxic agents that affect the cytocompatibility of the hydrogels.44 Instead, the viscoelastic nature of injectable hydrogels is more suitable for applications in soft tissues like skin, cardiac tissues and neural tissues.43
Injectable hydrogels are viscoelastic materials that are able to fill the irregularly-sized defects in the CNS injuries. They may also facilitate tissue-implant integration and allow modulation of the microenvironment to reduce scarring while promoting regeneration.45–50 The use of hydrogels provides a more favorable environment by mimicking the natural ECM structure and/or providing suitable stiffness that supports cell attachment and functionality.51 The mechanical properties of hydrogels should be tuned to closely match the native CNS tissues, which possess a mechanical stiffness of about 100 Pa to 1000 Pa for both brain and spinal cord tissues,52,53 thus allowing primary cell attachment and differentiation towards a neural lineage. As hydrogels are crosslinked with high porosity, they are ideal carriers for neuroprogenitor cells. The potential of differentiating into functional neurons, astrocytes and oligodendrocytes aids in modulation of the microenvironment at the injury site.16
Natural polymers are a popular choice of material for injectable hydrogels due to their resemblance to the native CNS tissues. Many contain intrinsic amino acids that can be readily modified for cell adhesion.54 Some common materials of choice include collagen, gelatin, hyaluronic acid (HA), chitosan, alginate, agarose and methylcellulose.10,16,55,56 Such natural polymers have their inherent advantages as they are typically the components of the ECM, thus making them more biologically active in stimulating cellular functions.57 For example, collagen is known to improve cell migration,58 HA is known to directly activate intracellular signalling pathways via CD44 cell surface receptors59 and chitosan is known for its antibacterial properties.60 Combining the unique properties from various natural polymers and their inherent characteristics, such as biocompatibility, non-immunogenicity and non-toxicity, cells and host tissues could potentially have a higher chance of survival and proliferation in these hydrogels.61
Self-assembling peptides (SAPs) are amino acid-based molecules that undergo sol–gel transition at neutral pH and ionic concentration to form ECM-like networks.62 ECM molecules, growth factors and cells are also frequently incorporated to enhance cell attachment, cell migration and tissue regeneration.6 The most suitable time for regeneration to take place post traumatic CNS injury is at the sub-acute stage, i.e., 7–14 days post injury. This is the period when the initial cascade of inflammation starts to take place and the microenvironment is not too harsh for implantation to take place.63 A brief summary of the use of injectable hydrogels in CNS disease treatment is shown in Fig. 1A.
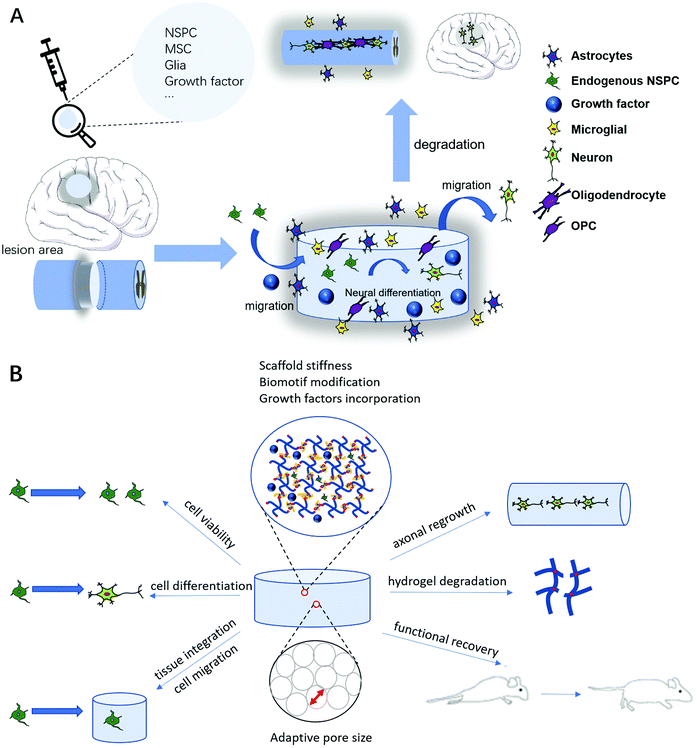 |
| Fig. 1 (A) Administration of injectable hydrogel gelates into the lesion area. The ECM-mimicking network modulates the microenvironment and promotes cell migration and differentiation in the lesion to restore nerve connection. (B) Possible cell behavior considerations of injectable hydrogels. | |
3.1. Challenges and advances of injectable hydrogels
Injectable hydrogels are able to form scaffolds in situ and fill irregular defects to aid in establishing tissue-implant integration. As injuries to the CNS are typically irregular in shapes, the use of injectable hydrogels eliminates the needs for preform scaffolds that require excising viable tissues to accommodate implantation and reduces the risks of scaffold deformation and compression.64,65 Scaffold compression may be unfavourable in CNS treatment as it can significantly increase scaffold stiffness beyond theYoung's modulus of the CNS tissues.66 In addition, when comparing to traditional scaffolding or nerve guides, injectable hydrogels are typically highly porous with a high proportion of water (>90%), making them ideal drugs and cell carriers with a controllable diffusion rate by changing the crosslinking density. A downside would be the high water content that significantly increases the rate of diffusion of hydrophilic drugs out of the hydrogel and could be problematic if prolonged drug release is required.67 The in situ gelation property of injectable hydrogels allows the release of molecules in a controlled fashion and prevents drugs from being washed away by the body fluids from the targeted regions.68 Lastly, as direct cell transplantation often faced significant cell death, injectable hydrogels are favorable in this context, since these hydrogels can support the survival of exogenous stem cells by allowing modification of scaffold stiffness, incorporation of cell binding cues and inclusion of growth factors.69–71
Advances in injectable hydrogels help to solve various clinical challenges in CNS disease treatment. Specifically, injectable hydrogels have received attention as carriers for proteins and drugs. However, initial burst release of loaded proteins and drugs can lead to undesired effects and ineffectiveness of treatment and this has been one of the main challenges for injectable hydrogels.72 Many approaches have been developed to overcome this problem. For example, the formation of complex networks via crosslinking to reduce the burst release of incorporated proteins and drugs,73,74 “pendant chain” system which delays the release by covalently grafting a protein backbone to the hydrogels via a cleavable linker,75 incorporation of proteins and drugs into micro- or nanoparticles to serve as a drug reservoir in the composite hydrogel system,76 and incorporation of high-affinity ligands to prolong drug release77 have all been reported. Other enhancements in the hydrogel system were also developed to improve the biological effects. In this respect, ligand-functionalization has been extensively studied to enhance cell infiltration and binding capabilities. Small oligopeptide sequences within the ECM proteins such as RGD peptides were incorporated to enhance cell adhesion within the hydrogel.78 Furthermore, peptide sequences like IKVAV and YIGSR are known to interact with mammalian neurons. Correspondingly, the incorporation of these sequences has been shown to enhance neural differentiation.79,80
3.2. Incorporation of exogenous cells in injectable hydrogels
Injectable hydrogels in combination with cell transplantation have been studied extensively in recent years in the hope of improving neural regeneration and functional recovery. Several types of primary cells have been explored. These include neural stem/progenitor cells (NSPCs), Schwann cells (SCs), olfactory-ensheathing cells (OECs), mesenchymal stem cells (MSCs) and human-derived induced pluripotent stem cells (iPSCs).20,63,81 Among these cell types, NSPCs, MSCs and glial cells are the common cell types to be incorporated within hydrogels for transplantation treatments. In particular, NSPCs can not only differentiate into mature neurons to replace lost cells, but also promote endogenous repair, such as enhancing angiogenesis, providing immunosuppression, and promoting recruitment of endogenous cells.82–84 MSCs, on the other hand, play a bigger role in contributing to the stimulation of angiogenesis and inhibition of microglial activation by secreting cytokines (IGF-1, VEGF, EGF and FGF) to reduce neuronal death. MSCs could also potentially differentiate towards the neural lineage.85,86 Glial cell transplantation could also be advantageous due to its potential to directly modulate the environment and meditate myelin regeneration.87
Despite the efforts from the past decades, there is no effective CNS disease treatment that results in the restoration of function. Here, we will look at the recent research studies on injectable hydrogels, including the use of cell transplantation, in the past decade. This ensures that the latest findings on the use of injectable hydrogels are covered. The choices of hydrogel materials will mainly be based on tissue and cellular responses. A brief summary of possible design considerations is shown in Fig. 1B.
3.3.
In vitro cell viability
Cell transplantation to the CNS often faces the problem of low cell survival rates. In this regard, in vitro cell culture studies focusing on evaluating the roles of hydrogels in the regulation of cellular responses have allowed the close examination of the viability of various cell types when delivered within these biomaterials. In particular, cell–matrix interactions are crucial in modulating cellular homeostasis and directing communication to cell cytoskeleton, growth factor receptors and intracellular signalling cascades to ensure cell survival.88Table 1 summarizes the in vitro studies on cell-incorporated injectable hydrogels and their corresponding cell viability and differentiation outcomes. Previous studies showed that mesenchymal and neural stem cells have the potential to replace lost cells and modulate inflammation and the local wound environment.89 Both NSPCs and MSCs seem to be able to survive and proliferate well with proper cellular and physical cues. Most studies have shown that these cells are able to survive in their respective hydrogels for more than 2 weeks,84,85,90–92 suggesting sufficient cell–matrix interactions between the incorporated cells and the hydrogel substrates.
Table 1 Examples of injectable hydrogels in enhancing cell viability and differentiation in vitro (papers are only taken from 2010 onwards)
|
Injectable polymers |
Cell source |
Culture conditions |
Culture duration |
In vitro cell viability |
In vitro differentiation |
Ref. |
Neural stem/progenitor cells |
Collagen/genipin |
NSCs |
DMEM-LG, 10% FBS, 1% penicillin–streptomycin, N2 supplement, and 20 nm mL−1 FGF |
10 days |
NSC viability reduces significantly at genipin concentrations higher than 0.25 mM after 3 days in culture. |
|
Macaya et al. (2011)55 |
SAP |
NSCs |
Serum-free basal medium, supplement FGF2 and EGF |
12 weeks |
Significant improvement in cell viability for cells encapsulated in SAP compared to Matrigel and collagen groups |
Significant increase in Tuj-1, GFAP and GalC expression by 12 weeks (∼30% Tuj-1, ∼25% GFAP and ∼25% GalC) in culture compared to days 3 (∼5% Tuj-1, <1% GFAP and <1% GalC) |
Koutsopoulos et al. (2013)98 |
HA-MC covalently modified with or without recombinant rat platelet-derived growth factor-A (rPDGF-A) |
Rat adult brain-derived NSPCs |
Neurobasal-A, 2% B27, 1% L-glutamine, 1% penicillin–streptomycin |
7 days |
Significant improvement in cell viability for NSPCs encapsulated in the hydrogels |
RIP (>2-fold increase) compared to HAMC and control groups. Non-significant between HAMC and control groups |
Mothe et al. (2013)105 |
HA modified with PLGA microspheres containing VEGF and Ang1 |
NSCs derived from mouse forebrain |
DMEM/F12 |
5 days |
NSCs were seeded and viable on the HA hydrogel with PLGA microspheres |
|
Ju et al. (2014)101 |
Silk fibroin hydrogel functionalized with IKVAV peptide |
hESC-derived NSCs |
(DMEM)/F12 medium, supplemented with 2% StemPro Neural Supplement and 2 mM Glutamax, bFGF (20 ng ml−1) and EGF (20 ng ml−1) |
7 days |
Silk-IKVAV group showed the higher fluorescence intensity of encapsulated cells than that of the unmodified silk group, reflecting greater cell viability |
β III-tubulin (3%). MAP2 (1.8%) |
Sun et al. (2017)114 |
HA-based self-polymerizing hydrogel |
Human iPSC-derived NSPCs |
DMEM F12 supplemented with L-glutamine, nonessential amino acids, penicillin–streptomycin, and 10 ng ml−1 basic FGF |
7 days |
Significant increase in NSPC proliferation in gels with an elastic modulus of 350 Pa |
|
Moshayedi et al. (2016)83 |
Gelatin methacrylate hydrogel (GelMA) for encapsulation of iPSC-derived NSCs. Cells were cultured for 7 days |
Human iPSC-derived NSCs |
1 : 1 mix of neurobasal medium and MEM/F12 supplemented with N2, B27, 1 × Glutamax and penicillin/streptomycin |
7 days |
Cell viability was found to be significantly higher in soft-GelMA at both days 3 and 7. Encapsulated cells in soft-GelMA showed elongation compared to rounded cells in medium- and stiff-GelMA |
Significant increase in the level of Tuj-1 in soft-GelMA compared to both stiff- (7.6-fold) and medium-GelMA (3.3-fold) at day 3 |
Fan et al. (2018)115 |
Collagen-HA-Laminin based hydrogel |
Rat spinal cord derived NPCs |
DMEM/F12, 1% N2, 1% penicillin/streptomycin |
5 days |
|
NG2: Col-HA-Lam (66.8%), Col-HA (40.1%), Col-Lam (24.1%) |
Geissler et al. (2018)107 |
Thiolated HA (HA-SH) modified with RGD/YIGSR/IKVAV |
hESC-NSPC |
hESC serum-free media, DMEM/F12, 1× StemPro neural supplement, 2 mM Glutamax, 0.1× antibiotic/antimycotic, 20 ng mL−1 bFGF, 20 ng mL−1 EGF |
>70 days |
Significantly higher viable cells in hydrogels with RGD than in hydrogels without RGD at day 70 |
Significant increase in olig2, β III-tubulin and OCX in 3D hydrogel culture compared to 2D culture Significant reduction in GFAP in 3D hydrogel culture compared to 2D culture |
Seidlits et al. (2019)71 |
Chondroitin-4-sulfate A (CS-A) hydrogel |
Human iPSC-derived NSPCs |
DMEM/F12, 45% Neurobasal, 0.5% N2 supplement, 1% B27 |
2 weeks |
Total number of survival neurons was nearly tripled compared to the non-encapsulated NPCs |
|
McCrary et al. (2020)84 |
HA matrix modified with laminin-derived IKVAV and Leu-Arg-Glu (LRE) peptide and GPQGIWGQ crosslinker (PEP + MMP-HA) |
hiPSC |
Supplement, 1% GlutaMAX, 1% nonessential amino acids, 0.1 mM β-mercaptoethanol (β-ME; Sigma Aldrich), 100 U mL−1 penicillin/streptomycin (Sigma Aldrich), 5% knockout serum replacement |
2 weeks |
The inclusion of IKVAV and LRE and/or GPQGIWGQ in the matrix did not significantly affect cell number in the matrices at any studied time point based on DNA content |
Tuj-1+ cells were found in all HA groups: (1) HA difunctionalised with azide and thiol reactive groups (DIFF-HA), (2) HA modified with IKVAV and LRE peptides (PEP + HA), (3) DIFF + MMP + HA and (4) PEP + MMP-HA. Only PEP + MMP-HA group was found to have significant increase in neurite length. |
Perera et al. (2020)106 |
Silk fibroin |
hiPSC-derived NSPC |
50% F12/DMEM, 50% Neurobasal medium, 1% Glutamax, 1% nonessential amino acids, 0.5% N2 supplement, 1% B27 supplement, 1% penicillin/streptomycin and 20 ng mL−1 FGF-2 |
>2 years |
DAPI counts maintain throughout the culture duration. |
|
Rouleau et al. (2020)92 |
|
|
|
|
|
|
|
|
Mesenchymal stem cells |
Gelatin polymer hydrogel |
Mouse BMSCs |
DMEM, 10% FBS, 1% penicillin–streptomycin |
2 weeks |
The BMSCs proliferated while maintaining their original morphology in the gels |
|
Osanai et al. (2010)85 |
|
Collagen/genipin |
Porcine MSCs |
DMEM-LG, 10% FBS, 1% penicillin–streptomycin |
10 days |
Significant increase in DNA content (7-fold increase) for MSCs in collagen/0.25 mM genipin gels from day 1 to day 7 |
|
Macaya et al. (2011)55 |
|
HA-MC hydrogels |
Human umbilical tissue-derived cells (hUTC) |
Dulbecco's modified Eagle medium, Penicillin–streptomycin, GlutaMAXTM |
3 days |
Out of 4 blends of hydrogel (0.5/0.5, 0.75/0.75, 1.0/1.0 and 1.0/0.75), only a significant decrease in encapsulated cells was found in the 1.0/1.0 blend |
|
Caicco et al. (2012)100 |
|
Self-assembling bone marrow homing peptide (BMHP1) |
Human endometrial-derived stromal cells (hEnSC) |
NPBM, bFGF, EGF, NSF-1, BDNF (50 ng/ml), 1% antibiotic, 2 nM glutamine |
21 days |
hEnSC viability significantly improved when encapsulated in BMHP1 |
Neural-like differentiation was observed for hEnSCs encapsulated into BMPH1 14 days post incubation Significant fold increment in Tuj-1, NF, MAP2 and Bcl2 expression 21 days post incubation |
Tavakol et al. (2016)90 |
|
|
|
|
|
|
|
|
Glial cells |
0.5%/0.5% w/v HA-MC hydrogels modified with RGD peptide and PDGF-A |
hiPSC-OPC |
OPC media |
7 days |
Significant increase in viable cells in HAMC-RGD (∼60%) compared to HAMC (∼25%) and TCP group (∼20%). |
|
Führmann et al. (2016)10 |
|
|
|
|
|
|
|
|
Primary cells |
Crosslinked sericin hydrogel (GSH) |
Cortical neurons from rat pup |
DMEM, supplemented with 2% B27, 1% glutamine and 1% penicillin/streptomycin |
20 days |
Primary cells effectively attached and grew on the GSH. The hydrogel promotes axon extension and branching of primary neurons. |
|
Wang et al. (2015)91 |
Many materials have been tested for cell survival and proliferation in vitro. Among these, we noticed significant improvement in cell proliferation in hyaluronan-, chondroitin sulfate- and collagen-based materials.55,71,83,84 The findings suggest that natural polymers found abundantly in the body are more suitable for inducing cell proliferation. We believe that the CD44 interaction could be the reason for improvement in cell viability. CD44 is a cell receptor found in various cell types, including neural stem cells and glial cells in the CNS.93–95 Specifically, CD44 forms receptor–ligand interactions with natural polymers including HA, chondroitin sulfate and collagen.96–98 The activation of the CD44 ligand binding domain allows binding of activator proteins that in turn triggers downstream signalling pathways, such as the Ras-Raf-MEK-ERK pathway, which leads to cell proliferation.99 While studies on cell viability in vivo typically last for 4–8 weeks, Rouleau and colleagues92 were able to maintain viable hiPSC-derived neurons and glial cells in the silk fibroin hydrogel for more than 2 years. However, given the fact that the silk fibroin hydrogel showed minimal degradation and maintained its hydrogel network over the long culture duration, such materials may be less ideal for the regeneration and replacement by new native tissues. Some studies only conduct in vitro experiments for less than 7 days.100,101 It could be beneficial to conduct in vitro experiments for a longer duration to ensure cell survival and the differential potential in vivo, where the environment is harsher.
3.4.
In vitro cell differentiation
Cell transplantation can modulate the diseased microenvironment by enhancing cell differentiation. Cell differentiation is crucial for the transplanted stem and progenitor cells to differentiate into mature and functional cells. This allows replacement of diseased tissues, promotes tissue regeneration through the secretion of regenerative factors and provides neuroprotection.102,103 While the effectiveness of cell transplantation depends largely on the number of cells transplanted, undifferentiated cells were shown to minimize cell death as compared to fully differentiated transplanted cells.104 Previous work done by Payne and colleagues82 showed that the transplantation of undifferentiated induced pluripotent stem cell-derived neural stem cells (iPSC-NSCs) resulted in greater functional repairs compared to late-differentiated cells which caused more tissue damage due to greater cell death.
From Table 1, some of the studies demonstrated the ability of injectable hydrogels to promote encapsulated cell differentiation to express immature neuronal markers at short time points105,106 and mature neural markers at longer time points.90,98 The choice of hydrogel materials seems to be critical in promoting the differentiation of encapsulated cells. SAPs are able to facilitate the differentiation of cells to express mature neuronal or oligodendrocyte markers without further modification of materials.90,98 Natural materials, on the other hand, often rely on additional modification to enhance cell differentiation in vitro. For example, hyaluronic acid-methylcellulose (HA-MC) alone did not enhance the differentiation of NSPCs as compared to cells cultured in neurobasal media.105 Collagen-only hydrogels worsened the differentiation potential of NSCs to neuronal lineage as compared to cells cultured on poly-D-lysine (PDL)-coated coverslips.107In vitro cell differentiation was enhanced when these natural polymers were further modified with bioactive motifs (e.g. IKVAV, LRE peptides) or incorporation of growth factors (e.g. PDGF, VEGF).108–111 We believe that, while injectable hydrogels made of natural materials are better in promoting cell viability in vitro, cell differentiation could be attributed to the presence of bioactive peptide sequences. SAPs are shown to have greater potential in promoting differentiation as they can be readily modified to include bioactive peptide sequences. Natural polymers, however, also achieved improved differentiation outcomes after biomotif modification. Specifically, Silva and colleagues112 compared the differentiation outcomes between scaffolds containing the bioactive IKVAV sequence, a non-bioactive sequence and a cell suspension with IKVAV soluble peptide. The results demonstrated that physical entrapment of bioactive epitope SAPs was the main reason for improvement in cell differentiation. Moreover, the stiffness of the hydrogels influences not only cell survival, but also the differentiation fate of transplanted cells.113 For example, silk is a stiff material by nature,83 thus affecting the differentiation potential of encapsulated neural stem cells. Although there are conflicting reports on cell differentiation potential in silk fibroin hydrogels in vitro,92,114 the high intrinsic stiffness of silk could explain the inconsistency in different studies and the low percentage of neural differentiation observed by Sun and colleagues. On the other hand, Fan and colleagues115 tested gelatin methacrylate hydrogels (GelMA) of different stiffnesses using a compression test. Specifically, three different stiffnesses of hydrogels were tested, including soft (∼680 Pa), medium (∼1230 Pa) and hard (∼2030 Pa), and the group concluded significant improvements in both in vitro cell viability and differentiation with soft-GelMA gels. The measured stiffness of the soft hydrogel matches the stiffness of the CNS tissues,52 which explains the findings from the study.
3.5.
In vivo tissue integration and cell migration
Tissue integration can be defined as the physical, biological and mechanical connection of the interface between the implanted materials and the surrounding native tissues.116 Poor tissue integration of the scaffold is often one of the factors contributing to poor cell survival and tissue regeneration in vivo. It often leads to the formation of fibrous scarring and cystic cavity which obstructs potential neuronal ingrowth.64,117 Proper integration between the tissue and scaffold often results in a high degree of cell infiltration to modulate the microenvironment in the lesion, given that the architectures of the scaffold are permissive to cell infiltration.118 In addition, tissue integration is also related to supporting axonal growth by facilitating endogenous cell infiltration and migration and deposition of laminin.119 Endothelial cell infiltration and the formation of blood vessels as blood flow are also essential to sustain the growth of regenerating axons and the surrounding tissues.84
In the local inhibitory microenvironment of the CNS, differentiation of transplanted stem cells into neurons for long-term survival and tissue integration is particularly challenging. As shown in Table 2, scaffolds that exhibit good tissue integration and promote cell migration typically result in reduced presence of reactive astrocytes (GFAP+) and inflammatory cells (IBA-1+). Further examination also confirms the ability of neurons to infiltrate into the hydrogel (NF+, NeuN+, DCX+, Tuj-1+, β III Tubulin+, etc.). We believe that these injectable hydrogels do provide sufficient tissue integration as evident from the reduced inflammation and enhanced cell migration and differentiation.
Table 2 Examples of injectable hydrogels in promoting tissue regeneration in vivo and functional recovery (papers are only taken from 2010 onwards)
|
Injectable polymers |
Cell source |
Implantation site |
Implant duration |
Tissue integration and cell migration |
Axonal regrowth |
Scaffold degradation |
Functional recovery |
Ref. |
Ang1, Angiogenesis marker; Bcl2, Antiapoptotic marker; GFAP, Astrocyte marker; GAD, Axons originating from GABAergic interneurons; Glut1, Brain endothelial cell marker; RIP, Early oligodendrocyte marker; BrdU; RECA, Endothelial cell marker; GAP43, Growth neurite marker; DCX, Immature neuron marker; MAP2; N52; NeuN; NF; RT-97, Mature neuron marker; IBA-1, Microglial marker; SMA, Muscular artery marker; MBP, Myelin protein marker; Tuj-1; β III-tubulin, Neuron-specific marker; Sox2, Neural stem cell marker; GalC; NG2; Olig2, Oligodendrocyte marker; Ki67, Proliferating cell marker; CGRP, Sensory axon marker; 5-HT, Serotonergic neuron marker. |
Neural stem/progenitor cells |
HA hydrogel matrix modified with RGD |
hiPSC-NPC |
1.5 mm anterior, 2 mm lateral to the bregma |
1 week |
Significant reduction in IBA-1+ signals in the cell + hydrogel group compared to cell only and control groups |
Significant increase in the DCX+ signal in the cell + hydrogel group compared to cell only and control groups |
|
|
Lam et al. (2014)9 |
Self-assembling laminin-derived epitope hydrogel |
hiPSC-NSC |
0.5 mm and 2.0 mm anterior, 2.5 mm lateral to bregma, and 1.5 mm below the dura |
36 weeks |
|
Grafted cells were observed around the hydrogels in the host tissue which were NeuN+. |
|
Significant functional improvement in forelimb sensorimotor function test in the cell + hydrogel group over cell only and control groups over 9 months post-injury |
Somaa et al. (2017)128 |
|
|
|
|
|
Significant increment in NeuN+ cells compared to cell-only group |
|
|
|
HA-MC hydrogels |
hiPSC-derived cortically-specified neuroepithelial progenitor cells (cMEP) |
Two locations corresponding to cortical lesion sites: AP 0.0 mm, ML 3.0 mm; and AP 2.3 mm, ML 3.0 mm |
50 days |
|
No significant difference in Sox2, DCX, βIII-tubulin and NeuN signals among the early-, mid-, and late-differentiated cell groups 50 days post-transplantation |
|
Comparing to day 4: Early-differentiated cNEPs: Significant improvement at day 42 and 56; |
Payne et al. (2019)82 |
|
|
|
|
|
|
|
Mid-differentiated cNEPs: Significant improvement at day 56 only |
|
|
|
|
|
|
|
|
Late-differentiated cNEPs: Non-significance at both days 42 and 56 |
|
Chondroitin sulfate-A hydrogel |
hiPSC-NSPCs |
Intracranial transplantations (2 μL, 100,000 cells μL−1) were performed into the stroke core region |
2 weeks |
Significant increase in the number of microvessels (Glut1, SMA, BrdU) in the stroke core region following treatment with hydrogel + cell compared to cell or hydrogel only groups 2 weeks post-transplantation |
|
|
Significant forelimb sensorimotor function test in the cell + hydrogel group over 2 weeks compared to the hydrogel only group and control |
Mccrary et al. (2020)84 |
|
Mesenchymal stem cells |
Poly(N-isopropylacrylamide) (PNIPAAm), crosslinked with either PEG or MC |
Rat skin fibroblasts (RSFs) |
C4-5 hemisection or L4-L5 hemisection |
2 weeks |
Hydrogels allow cell migration from the host tissue into the scaffold |
RT-97 staining observed in both PNIPAAm-PEG and PNIPAAm-MC 2 weeks post-implantation |
PNIPAAm-PEG is non-degradable |
|
Conova et al. (2011)8 |
|
|
|
|
IBA-1 staining indicates no increase in inflammatory response compared to commercial Gelfoam matrix |
CGRP staining indicates that axons are grown from the dorsal root into the lesion |
PNIPAAm-MC's degradability was not tested |
|
|
|
Glial Cells |
0.5%/0.5% w/v HA-MC hydrogels modified with RGD peptide and PDGF-A |
hiPSC-OPC |
1 week after clip compression at T2 |
8 weeks |
The HAMC-RGD/PDGF-A group showed significant reduction in cystic cavity compared to the cell transplantation only group and control |
NF+ axons were observed to be able to extend processes into the graft |
|
Non-significant improvement over the control group throughout 9 weeks post-injury (except week 8) |
Führmann et al. (2016)10 |
|
|
|
|
Limited glial scarring observed in the HAMC-RGD/PDGF-A group |
|
|
|
|
|
Acellular |
Fibrin/fibronectin (FB/FN) hydrogel |
|
T7–T9 hemisection |
4 weeks |
FB and FB/FN hydrogels generally integrated to the spinal cord with little to no cavities, while the FN hydrogel showed large cavities at the tissue–implant interface 4 weeks post-surgery |
Significantly higher N52+ signal at 4 weeks for FB/FN (∼12%) compared to FB (∼7%), but non-significant compared to FN (∼10%) |
|
|
King et al. (2010)119 |
HA-MC hydrogel incorporating erythropoietin |
|
A disk with 2 mm opening was fixed over the burr hole with bone glue. Hydrogels were injected into the hole such that the gel is in direct contact with the brain cortical surface |
11 days |
Significant reduction in the GFAP+ signal in the ipsilateral cortex ventral to the lesion site in the HAMC group at both 4 d and 11 d post-injury compared to the non-treated group |
HA-MC incorporating erythropoietin group significantly increased the number of DCX+ positive cells compared with the no treatment or HAMC group at 4d and 11d post implantation (2-fold increase) |
PNIPAAM is non-degradable |
|
Wang et al. (2012)129 |
|
|
|
|
|
HA-MC incorporating erythropoietin enhances the number of NeuN+ mature neurons in the injured area by more than 4-fold at day 4 and more than 10-fold at day 11 |
|
|
|
Free GDNF loaded or GDNF microsphere loaded alginate/fibrinogen hydrogel |
|
T9-T10 hemisection |
12 weeks |
|
NF+ signal was significantly higher in the free GDNF group compared to the microsphere-loaded GDNF group and non-treated group 6 weeks post-surgery |
|
The free GDNF group achieves superior results in hindlimb paw angle and left hind paw intensity using CatwalkTM compared to the microsphere-loaded group and non-treated group |
Ansorena et al. (2013)56 |
|
|
|
|
|
NF+ signal was significantly higher in the free GDNF group compared to the non-treated group but non-significant against the microsphere-loaded group 3 months post-surgery |
|
|
|
|
|
|
|
|
GAP43+ signal was significantly higher in the microsphere-loaded group compared to the free GDNF group and non-treated group both 6 weeks and 3 months post-surgery |
|
|
|
VEGF loaded alginate/fibrinogen hydrogel |
|
T9–10 hemisection |
4 weeks |
Significant increase in endothelial cell infiltration (RECA+) in hydrogels loaded with VEGF 4 weeks post-surgery |
Significantly higher neural cells (βIII tubulin+) in the VEGF loaded hydrogel group (∼16%) compared to the control group (∼11%) but non-significant against the hydrogel only group (∼13%) 4 weeks post-surgery |
|
|
des Rieux et al. (2014)123 |
|
|
|
|
|
Significantly higher neurite growth (GAP43+) in the VEGF loaded hydrogel group (∼14%) against hydrogel only (∼9%) and control groups (∼3%) |
|
|
|
SAP hydrogel made of K2(QL)6K2 (QL6) |
|
1 day after T6-7 clip injury |
8 weeks |
Significant reduction in both GFAP+ and IBA-1+ signals in the lesion are in the QL6 group compared to control 8 weeks post-injury |
BDA retrograde labeling showed significant increment in the density of the BDA-labeled fibers in the QL6 group compared to the control group 8 weeks post-SCI at 2 mm, 4 mm and 8 mm rostral to the lesion center |
The scaffold was found to be almost fully degraded in vivo at 8 weeks |
Significant overall improvement in the BBB locomotor score over the control group |
Liu et al. (2013)120 |
HA based hydrogel modified with anti-Nogo receptor antibody and poly-L-lysine incorporated VEGF and Ang1 implantation |
|
1.5 mm × 1.5 mm × 1.0 mm cortical cavity |
10 weeks |
Significant reduction in IBA-1+ and GFAP+ 2 weeks post-injection compared to the non-treatment group |
|
|
After implantation of the HA hydrogel together with PLGA microspheres, a significant improvement in forelimb sensorimotor functional test was observed at 6 weeks compared to the HA hydrogel only group |
Ju et al. (2014)101 |
HA-MC hydrogels with cyclosporine-A |
|
Brain's cortical surface |
2 weeks |
|
HA-MC treatment with cyclosporine-A significantly enhanced the number of proliferating cells (Ki67+), with a 1.32-fold increase compared to untreated group and a 1.11-fold increase compared to HA-MC only group after 7-day post-implantation. However, DCX staining showed non-significance among groups |
|
|
Tuladhar et al. (2015)11 |
Single walled carbon nanotube-functionalised poly(N-isopropylacrylamide) (SWNT-PNIPAAM) |
|
C7 1 mm × 1 mm × 1 mm incision |
8 weeks |
Migrated neuron observed in the injury site with reduction in cyst size and tissue scarring compared to the PNIPAAM only group and non-treated sham group |
β III-tubulin staining showed infiltration of axons into the scaffold in SWNT-PNIPAAM group |
|
|
Sang et al. (2016)133 |
Porcine-derived UBM-ECM hydrogel |
|
Ventral posterior region of the cavity |
12 weeks |
|
Infiltration of DCX+ cells is evident in the peri-infarct area, as well as within the degrading ECM hydrogel 1 day post-injury |
Decrease of approximately 32% of ECM hydrogel volume over 12 weeks post-implantation and the lesion volume was reduced by 28% |
Non-significant functional recovery |
Ghuman et al. (2017)124 |
|
|
|
|
|
Neovascularization (RECA) was observed in 3 mg mL−1 and 4 mg mL−1 hydrogel groups, but not in the 8 mg mL−1 group |
|
|
|
Imidazole-poly(organophosphazenes) (I-5) hydrogel |
|
T10-11 1 week after 200 kDyns contusion |
8 weeks |
I-5 injection resulted in an almost complete disappearance of cystic cavity 4 weeks post-injection, while an increase in cystic cavity size was observed in the control group |
A substantial number of NF+ axons observed in the matrix. |
Rapid degradation of hydrogel in vitro with 7 days |
Significant improvement in the BBB score at 6, 7 and 8 weeks post-surgery |
Hong et al. (2017)122 |
|
|
|
|
|
Significant increase in 5-HT+ axons 8 weeks post-surgery |
|
|
|
HA-based microporous annealed particle (MAP) hydrogel |
|
Stereotaxic coordinates 0.26 mm AP, 3 mm ML and 1 mm DV |
10 days |
Significant reduction in both GFAP+ and IBA-1+ in the MAP group |
Significant increment in Glut-1+, DCX+ and Ki67+ in the peri-infract area |
|
|
Nih et al. (2017)121 |
aFGF-loaded heparin-poloxamer hydrogel |
|
T9 after moderate crushing injury using a vascular clip |
4 weeks |
Significant reduction in both glial scar thickness and glial scar volume in the aFGF-HP group 28 days post-surgery. |
Significant improvement in MBP+, MAP2+, and NF+ signals in the aFGF-HP group 28 days post-surgery. |
|
Significant improvement in the BBB score and inclined plane core for the aFGF-HP group at 3, 7, 14 and 28 days post-surgery. |
Wang et al. (2017)130 |
|
|
|
|
|
Anterograde BDA staining 5 mm caudal to the injury suggested significant axonal regeneration into the lesion in the aFGF-HP group 28 days post-surgery |
|
|
|
Decellularized optimized acellular nerve-derived hydrogel (iOA) |
|
C3/4 after 200 kDynes contusion |
8 weeks |
|
Significant increase in %NF+ coverage at the distal area in the iOA group compared to control 8 weeks post-surgery, but non-significant throughout the lesion |
Rapid degradation of hydrogel |
Non-significant functional outcome |
Cornelison et al. (2018)134 |
Glycosaminoglycan-based hybrid hydrogel encapsulating SDF-1α and bFGF |
|
2 mm lateral to the midline of a 3 mm by 5 mm craniotomy window and 1.5 mm anterior to the Bregma |
5 weeks |
|
DCX+ signal increased 4-fold and Ki767+ signal increased 3-fold 21 days post-implantation |
|
A significant recovery of forelimb movements could be seen clearly in the hydrogel group throughout 4 weeks post-implantation compared to the PBS group |
Jian et al. (2018)13 |
Porcine-derived urinary bladder matrix (UBM)-ECM hydrogel |
|
Ventral posterior region of the cavity |
90 days |
|
Significant increment in NeuN+ cells in 3 mg mL−1 and 4 mg mL−1 hydrogel groups compared to the 8 mg mL−1 hydrogel group |
Approximately 80% of the low-strength ECM hydrogel was degraded within 14 days of implantation and the density of nerve cells in ischemia cavity gradually increased, whereas only 32% of the high-intensity ECM hydrogel resorbed by 90 days |
|
Ghuman et al. (2018)135 |
Overall, there seems to be no distinctively “perfect” material that promotes tissue integration and cell migration. In fact, many studies have shown evidence of improvement in scaffold–tissue connection and cell infiltration regardless of the choice of materials. Improvement in tissue–scaffold integration can be indicated by (1) a reduction in tissue scarring,101,120,121 (2) a reduction in cystic cavity,119,122 and (3) infiltration of endothelial cells.84,123,124 While natural polymers and ECM-mimicking SAPs can be modified to improve implant integration into native tissues, it is worthwhile to note that other than ensuring good integration to the tissues surrounding the lesion, the pore size of the scaffold should also be carefully considered to ensure that the internal architecture of the hydrogel is conducive to cell infiltration.5 Furthermore, while scaffolds are usually made of hydrophilic materials or coupled with hydrophilic antigens to improve tissue and cell attachment, the stiffness of the scaffold is also an important parameter to consider for proper tissue integration and promoting cell migration. As studied by Lam and colleagues,9 HA hydrogels with two different stiffnesses were examined and significantly higher GFAP+ signals were found in the animals that were implanted with the stiffer scaffold. This potentially leads to larger cystic cavity and reduced cell migration.
3.6.
In vivo axonal regrowth
One of the main objectives in CNS therapy is to promote axonal regrowth and to reconnect neuronal network. Axonal regeneration is one important factor influencing tissue recovery by sprouting uninjured axons and eventually leading to functional recovery.125 The use of injectable hydrogels could enhance axon regeneration from the corticospinal tract (CST).6 Cell transplantation also facilitates the regeneration by either the differentiation of exogenous transplanted cells9 or facilitating the differentiation of endogenous neurons.13 Since injured axons in the CNS do not actively regenerate, the amount and extent of axonal regrowth within the scaffold are important parameters to be considered for potential functional recovery. From Table 2, most groups deployed the strategy of attracting the migration of endogenous NSPCs into the lesion and promoting their proliferation and differentiation into neurons. Although neuron markers like DCX and Tuj-1 could sufficiently prove the capability of these hydrogels in promoting neural-like proliferation and differentiation, most studies do not provide evidence of mature neuron differentiation. On the other hand, mature neuron markers (MAP2, NeuN, NF, etc.) could be better choices as indicators of growing mature neurons that are associated with axonal sprouting.126 This is because axonal sprouting is known for strengthening existing connections and facilitating new synaptic connection across the lesion, which are essential to restore proper signal transmission.127
While most growth factor-incorporated scaffolds seemed to be more effective in promoting axonal regrowth and tissue regeneration, we identify three materials with significant improvement in axon regeneration marked by the expression of mature neuron markers: SAP,128 HA-MC10,129 and heparin-poloxamer (HP).130 The SAP was made of laminin-derived IKVAV,128 HA-MC, was modified with the RGD sequence and growth factors were incorporated in both HA-MC and HP hydrogels.10,130 These modifications facilitated attachment and promoted the proliferation of cells to modulate the microenvironment that is conducive to axonal regrowth. The incorporation of OPCs in the hydrogel also potentiates the differentiation into oligodendrocytes.10 We believe that these materials, with proper modifications that facilitate cell infiltration and differentiation, could effectively promote axonal regeneration and preserve the integrity of newly formed axons. In addition, BDA labelling done by Liu's group120 (SAP hydrogel) and Wang's group130 (HP hydrogel) confirmed the connection of neurons between newly formed axons and those in native tissues, which could potentiate functional recovery.
3.7. Scaffold degradation
The degradation rate of an injectable scaffold plays an important role in tissue regeneration. Fast scaffold degradation potentially leads to implant failure as the void space suppresses the formation of mechanically competent tissues to support the growth and development of newly formed cells and tissues.131,132 In contrast, slow scaffold degradation impedes the growth of new axons and tissues.6 With the implantation time being prolonged, an excessively large volume of hydrogel will reduce the invasion of migrating cells and the potential recovery could be sub-optimal. One important consideration for hydrogel degradation is to anticipate the rate of degradation with respect to axonal regrowth.
From Table 2, the non-degradable poly(N-isopropylacrylamide) (PNIPAAm) scaffold could provide permanent support for regeneration,8,133 but it could potentially prevent tissue regrowth. On the other hand, the rapid degradation of the decellularized optimized acellular nerve-derived hydrogel (iOA)134 resulted in thick and dense GFAP at the scaffold–tissue interface. We believe that the lack of overall neurite ingrowth and functional recovery could be due to insufficient early mechanical support. In the early stage of hydrogel scaffold implantation, there will be continuous cell migration, including macrophages, NSPCs, astrocytes and OPC, where the migration of macrophages accelerates the biodegradation of the scaffold.135 Loss of mechanical support leads to failure in the cell–ECM interaction and the following signal transduction to promote cell development and growth, hence the decline in the potential regrowth of native tissues to serve as the structural support to continue assisting in cell and tissue regeneration. In this regard, Cornelison et al.134 suggested a duration that is required for the onset of axon regeneration, which is typically between 4 and 6 weeks post-injury. However, we observed robust NF infiltration into fiber–hydrogel scaffolds as early as one week post-injury.136 Significant improvement in axonal regrowth was also found in other studies with the hydrogel degradation rate being faster than this proposed period.122,135 These observations could be attributed to the hydrogel materials that were used and the corresponding affinity of cells towards these materials, which in turn facilitated cell–matrix interactions to promote tissue regrowth. Along this line, studies have also shown that hydrogel degradation plays a significant role in influencing cell behavior and differentiation.137 For example, MSC spreading and survival have been shown to be directly correlated to the hydrogel degradation rate.138 Therefore, the degradation rate of hydrogels should be tailored to the hydrogel materials used and controlled to promote cell survival and differentiation behavior.
3.8. Functional recovery
One of the ultimate goals of CNS treatment is to restore both sensory and motor functional capabilities. Axonal regeneration has been shown to be closely related to functional recovery in CNS diseases. Angiogenesis and recruitment of cells for neurogenesis to the injured areas are equally crucial.1,83,139,140 While axonal regrowth into the lesion is encouraging, functional recovery requires reconnection of neurons between rostral and caudal sides.123 From Table 2, the discoveries from various research groups seem to suggest that significant axonal regrowth does not always equate to functional recovery. For example, HA-based hydrogels were observed to promote axonal regrowth in the previous sections.10,129 However, not all studies showed the expected functional recovery. One possible reason could be the differentiation nature of transplanted cells. Führmann et al.10 suggested that only a portion of transplanted cells would differentiate into mature and functional oligodendrocytes and myelinated axons. Payne et al.82 compared the differentiation stages of NSPCs in transplantation and found out that late-differentiated cells were less effective in promoting functional recovery. Perhaps the maturity of transplanted cells should also be an important consideration, given that matured cells are more sensitive to the injection transplantation process. In addition, the type of transplanted cell also plays a role in either supporting the growth of endogenous tissues or integrating with the host tissues.141 Embryonic stem cells (ESCs) are known to be capable of differentiating into nearly all cell phenotypes. Advances in genetic modification allow directed differentiation of ESCs to the desired cell types, such as neurons and oligodendrocytes for CNS repair.142 MSCs are used in cell transplantation in the CNS for both neuroprotection and neuroregeneration strategies, where cells are used to either protect parenchymal cells in the lesion or promote axonal regeneration and sprouting. MSCs are able to secrete pro-survival growth factors (e.g. BDNF, VEGF, FGF2, etc.) and have the potential to be differentiated into neurons.143 The use of iPSCs has gained popularity in recent years due to the ability to reprogram to xeno-free and genetically stable cells to potentiate recovery in the CNS such as neuroprotection, modulation and regeneration. The ability to be derived into different neural lineages, for example, neural network reconstruction by iPSC-derived neural cells, axon remyelination by oligodendrocytes, and neurotrophic factors secreted by neural cells, shows promising therapeutic effects for cell transplantation.144
As a result of CNS injury, trophic factors are downregulated.145 Many groups have incorporated growth and trophic factors into hydrogels to help stimulate neural regeneration and growth. Both Ansorena et al.56 and des Rieux et al.123 studied growth factor-loaded alginate/fibrinogen hydrogels, but the latter were unable to achieve improved functional recovery. This could be due to the insufficient amount of growth factors incorporated into the hydrogels to support axonal growth. Lutton et al.146 also suggested that the sole effect of VEGF might not be sufficient for modulating regeneration in the complex CNS environment and would require a combination of effects from other growth or trophic factors. Scaffolds that promoted decent animal behaviour outcomes typically had a degradation rate of no less than 4 weeks and were almost fully degraded at about 8 weeks post-injury. The fast rate of hydrogel degradation might be a possible reason for poor functional recovery in some studies as fast degradation of hydrogels failed to provide sufficient support for growing axons.134
There are many factors contributing to functional recovery after CNS injuries. Although studies show conflicting findings on the correlation between axonal regeneration and functional recovery, reconnection of the neural network is still important as neural signal conduction is crucial for recovery of movements. Axonal regeneration is affected by the hydrogel material used, the maturity and types of transplanted cells, the involvement of hydrogel modification and growth factors, and hydrogel degradation. Recent studies have also focused on the involvement of glial cells in axonal regeneration and functional recovery. For example, studies have suggested that astrocyte recruitment to the lesion site may have a permissive role in axonal growth instead of only forming an inhibitory barrier147,148 and demonstrated the ability for inflammation regulation and minimizing cellular degeneration.149,150 OPC proliferation, oligodendrocyte differentiation and maturation are also important to promote axonal remyelination.151
4. Summary and suggestions for future works
The treatments for CNS diseases are limited. The prognosis of patients is often poor and this could seriously reduce their quality of life. Stem cell transplantation has registered several clinical studies by protecting and repairing CNS injuries. The transplanted stem cells can aid in repairing damaged nerves and also play a beneficial role through immune regulation or regulation of endogenous regeneration. However, cell transplantation faces minimal success at clinical stage mainly to due poor survivability and poor tissue integration of transplanted cells.17
From the studies in the recent years, HA-based hydrogels seem to be more popular options in CNS disease treatments. Since the first report of the study involving HA coupled with methyl cellulose,152 hyaluronan-based hydrogels have been widely used in the animal models for the treatments of CNS diseases owing to its injectability and thermoresponsiveness. HA also helps to attenuate inflammatory response and promote endogenous axonal regrowth in the body.11 Nevertheless, regardless of hydrogel materials, recent CNS disease treatments seem to be focusing on eliciting endogenous neurogenesis, probably due to the concerns in immune response due to exogenous cell transplantation and the possibility of teratoma formation. Although exogeneous cell incorporation in injectable hydrogels has become an increasingly popular option, many studies only focused on the potential and differentiation outcomes of cell encapsulation within injectable hydrogels in vitro, but did not proceed with cell transplantation in vivo and only focused on the effects of acellular hydrogels and encapsulated molecules on tissue regeneration. It is difficult to determine the best scaffold material with the most effective therapeutic outcomes. For example, certain materials are more effective in promoting cell survival (e.g. natural materials) and certain materials promote cell differentiation (e.g. SAPs). ECM modifications and biomolecule incorporation are also effective in enhancing the therapeutic effects of injectable hydrogels. The choice of hydrogel materials could, therefore, be based on the desired outcome of the studies. We are hopeful that the information provided in this review could offer insights that would be useful for the future design of injectable hydrogels.
To ensure that research on injectable hydrogels is clinically relevant, functional recovery should be the focus of the studies. This is because the end goal for any treatment options is to improve and restore the functional capabilities of patients.153 The CNS functions are so diverse and complex that it is not possible to only use a single assessment method to cover all aspects of functions. This includes sensory, locomotor, and cognitive functions such as learning, memory and problem solving.154 Sensory recovery and feedback are important in controlling motor functions, while motor recovery is responsible for initiating muscle fibers to achieve limb movements.155 Common sensory tests include Von Frey Hair (VFH) microfilament tactile sensory test156 and heat and cold sensation tests.157,158 Locomotor tests, on the other hand, include Basso, Beattie and Bresnahan (BBB) scale,159 open field tests160–163 and swim tests.164 As symptoms of anxiety are common post-stroke and SCI, open field tests that make use of rodents’ behavior to stay at the corners under stress or anxiety are also commonly used.155,165 Cognition assessment is crucial for neurological functions including memory and emotions. This is especially critical after stroke as patients often suffer from cognitive impairments.166 Some common methods to assess memory in animals include Morris water maze test (Morris, 1981),167 Y-maze test,168 novel object recognition/location test169 and radial-arm test.170 The key for choosing the appropriate functional assessment scale should depend on the surgical models used and knowing the advantages and limitations of different systems to choose the most appropriate assessment methods.
Instead of discovering new material combinations for CNS disease treatment, the focus of future research may shift more from the development of new materials to understanding the mechanisms behind the tissue response towards the hydrogels and axon reconnection leading to functional recovery after hydrogel implantation. While animal models are essential to examine the effectiveness of injectable hydrogels in vivo, alternatives, such as ex vivo or organ-on-a-chip models, may be useful platforms to assess the effectiveness of hydrogel materials. Ex vivo models, organotypic slice culture (OSC), for example, can serve as alternatives to assess the post-injury interaction of cells within the spinal cord. OSC is able to mimic the post-injury environment by recapitulating elements of glial scar. This could be useful in tackling the issues of glial scarring in spinal cord repair.171 Neural system-on-a-chip is a scaffold-based in vitro system for studies of complex physiological tissue interactions on a functional and customizable substrate. The system may be useful as a platform to study the nerve injury model since it allows direct observation of the states of injury and regeneration. It also potentially allows the studies of higher order neural anatomies.172 With these platforms, we could possibly achieve direct observation of cellular response towards various scaffold materials within a shorter period of time and with reproducibility. This could ease the efforts of testing scaffolds in vivo and cut down on the costs of animal studies. The limitations of these platforms could be the inability to assess inflammatory response and interaction between the immune system and the injury lesion. Additionally, functional studies can only be carried out with in vivo models.
Despite some limitations in current studies on in situ injectable hydrogels, some groups have managed to obtain promising axonal recovery and functional improvement. This provides incentives for continuing the development of injectable hydrogels in CNS injury treatment. With increasing understanding on the physiologies of CNS, there is hope that we can engineer optimal injectable hydrogels that are useful and beneficial to clinical applications.
Conflicts of interest
The authors declare no conflict of interest.
Acknowledgements
This work was supported by the National Research Foundation, Singapore, under its Intra-CREATE Thematic Grant Programme (NRF2019-THE002-0001) and the MOE Tier 1 grants (RG38/19 and RG37/20).
Notes and references
- A. Gopalakrishnan, S. A. Shankarappa and G. K. Rajanikant, Hydrogel scaffolds: Towards restitution of ischemic stroke-injured brain, Transl. Stroke Res., 2019, 10(1), 1–18 CrossRef CAS PubMed.
- S. H. Koh and H. H. Park, Neurogenesis in stroke recovery, Transl. Stroke Res., 2017, 8(1), 3–13 CrossRef CAS PubMed.
- A. S. Go, D. Mozaffarian, V. L. Roger, E. J. Benjamin, J. D. Berry and W. B. Borden,
et al., Heart disease and stroke statistics – 2013 update: A report from the American Heart Association, Circulation, 2013, 127(1), e6–e245 Search PubMed.
- J. H. Badhiwala, C. S. Ahuja and M. G. Fehlings, Time is spine: A review of translational advances in spinal cord injury, J. Neurosurg., 2018, 30(1), 1–18 Search PubMed.
- D. Macaya and M. Spector, Injectable hydrogel materials for spinal cord regeneration: A review, Biomed. Mater., 2012, 7(1), 012001 CrossRef CAS PubMed.
- P. I. Morgado, M. Palacios and J. Larrain,
In situ injectable hydrogels for spinal cord regeneration: advances from the last 10 years, Biomed. Phys. Eng. Exp., 2019, 6, 1 Search PubMed.
- Z. Yu, H. Li, P. Xia, W. Kong, Y. Chang and C. Fu,
et al., Application of fibrin-based hydrogels for nerve protection and regeneration after spinal cord injury, J. Biol. Eng., 2020, 14, 22 CrossRef CAS PubMed.
- L. Conova, J. Vernengo, Y. Jin, B. T. Himes, B. Neuhuber and I. Fischer,
et al., A pilot study of poly(N-isopropylacrylamide)-g-polyethylene glycol and poly(N-isopropylacrylamide)-g-methylcellulose branched copolymers as injectable scaffolds for local delivery of neurotrophins and cellular transplants into the injured spinal cord, J. Neurosurg., 2011, 15(6), 594–604 Search PubMed.
- J. Lam, W. E. Lowry, S. T. Carmichael and T. Segura, Delivery of iPS-NPCs to the stroke cavity within a hyaluronic acid matrix promotes the differentiation of transplanted cells, Adv. Funct. Mater., 2014, 24(44), 7053–7062 CrossRef CAS PubMed.
- T. Fuhrmann, R. Y. Tam, B. Ballarin, B. Coles, I. Elliott Donaghue and D. van der Kooy,
et al., Injectable hydrogel promotes early survival of induced pluripotent stem cell-derived oligodendrocytes and attenuates longterm teratoma formation in a spinal cord injury model, Biomaterials, 2016, 83, 23–36 CrossRef CAS PubMed.
- A. Tuladhar, C. M. Morshead and M. S. Shoichet, Circumventing the blood-brain barrier: Local delivery of cyclosporin A stimulates stem cells in stroke-injured rat brain, J. Controlled Release, 2015, 215, 1–11 CrossRef CAS PubMed.
- J. M. Obermeyer, A. Tuladhar, S. L. Payne, E. Ho, C. M. Morshead and M. S. Shoichet, Local delivery of brain-derived neurotrophic factor enables behavioral recovery and tissue repair in stroke-injured rats, Tissue Eng., Part A, 2019, 25(15–16), 1175–1187 CrossRef CAS PubMed.
- W. H. Jian, H. C. Wang, C. H. Kuan, M. H. Chen, H. C. Wu and J. S. Sun,
et al., Glycosaminoglycan-based hybrid hydrogel encapsulated with polyelectrolyte complex nanoparticles for endogenous stem cell regulation in central nervous system regeneration, Biomaterials, 2018, 174, 17–30 CrossRef CAS PubMed.
- L. Fernandez-Garcia, J. Perez-Rigueiro, R. Martinez-Murillo, F. Panetsos, M. Ramos and G. V. Guinea,
et al., Cortical reshaping and functional recovery induced by silk fibroin hydrogels-encapsulated stem cells implanted in stroke animals, Front. Cell. Neurosci., 2018, 12, 296 CrossRef PubMed.
- M. J. Cooke, Y. Wang, C. M. Morshead and M. S. Shoichet, Controlled epi-cortical delivery of epidermal growth factor for the stimulation of endogenous neural stem cell proliferation in stroke-injured brain, Biomaterials, 2011, 32(24), 5688–5697 CrossRef CAS PubMed.
- K. S. Straley, C. P. F. Wong and S. C. Heilshorn, Biomaterial design strategies for the treatment of spinal cord injuries, J. Neurotrauma, 2010, 27, 1–19 CrossRef PubMed.
- T. C. Lim and M. Spector, Biomaterials for Enhancing CNS Repair, Transl. Stroke Res., 2017, 8(1), 57–64 CrossRef CAS PubMed.
- V. A. Kornev, E. A. Grebenik, A. B. Solovieva, R. I. Dmitriev and P. S. Timashev, Hydrogel-assisted neuroregeneration approaches towards brain injury therapy: A state-of-the-art review, Comput. Struct. Biotechnol. J., 2018, 16, 488–502 CrossRef CAS PubMed.
- B. Niemczyk, P. Sajkiewicz and D. Kolbuk, Injectable hydrogels as novel materials for central nervous system regeneration, J. Neural Eng., 2018, 15(5), 051002 CrossRef CAS PubMed.
- M. Modo, Bioscaffold-induced brain tissue regeneration, Front. Neurosci., 2019, 13, 1156 CrossRef PubMed.
- V. Feigin, B. Norrving, C. L. M. Sudlow and R. L. Sacco, Updated criteria for population-based stroke and transient ischemic attack incidence studies for the 21st century, Stroke, 2018, 49(9), 2248–2255 CrossRef PubMed.
- C. L. Gooch, E. Pracht and A. R. Borenstein, The burden of neurological disease in the United States: A summary report and call to action, Ann. Neurol., 2017, 81(4), 479–484 CrossRef PubMed.
- A. Alizadeh, S. M. Dyck and S. Karimi-Abdolrezaee, Traumatic spinal cord injury: An overview of pathophysiology, models and acute injury mechanisms, Front Neurol., 2019, 10, 282 CrossRef PubMed.
- H. S. Sekhon and M. G. Fehlings, Epidemiology, demographics, and pathophysiology of acute spinal cord injury, Spine, 2001, 26, S2–S12 CrossRef PubMed.
- C. H. Tator and M. G. Fehlings, Review of the secondary injury theory of acute spinal cord trauma with emphasis on vascular mechanisms, J. Neurosurg., 1991, 75, 15–26 CAS.
- C. Profyris, S. S. Cheema, D. Zang, M. F. Azari, K. Boyle and S. Petratos, Degenerative and regenerative mechanisms governing spinal cord injury, Neurobiol. Dis., 2004, 15(3), 415–436 CrossRef PubMed.
- J. W. Fawcett, The extracellular matrix in plasticity and regeneration after CNS injury and neurodegenerative disease, Prog. Brain Res., 2015, 218, 213–226 Search PubMed.
- N. Hlavac, M. Kasper and C. E. Schmidt, Progress toward finding the perfect match: Hydrogels for treatment of central nervous system injury, Mater. Today Adv., 2020, 6, 100039 CrossRef.
- A. D. Gaudet and L. K. Fonken, Glial cells shape pathology and repair after spinal cord injury, Neurotherapeutics, 2018, 15(3), 554–577 CrossRef CAS PubMed.
- M. Pekny, U. Wilhelmsson, T. Tatlisumak and M. Pekna, Astrocyte activation and reactive gliosis: A new target in stroke?, Neurosci. Lett., 2019, 689, 45–55 CrossRef CAS PubMed.
- I. B. Wanner, M. A. Anderson, B. Song, J. Levine, A. Fernandez and Z. Gray-Thompson,
et al., Glial scar borders are formed by newly proliferated, elongated astrocytes that interact to corral inflammatory and fibrotic cells via STAT3-dependent mechanisms after spinal cord injury, J. Neurosci., 2013, 33(31), 12870–12886 CrossRef CAS PubMed.
- M. A. Anderson, J. E. Burda, Y. Ren, Y. Ao, T. M. O'Shea and R. Kawaguchi,
et al., Astrocyte scar formation aids central nervous system axon regeneration, Nature, 2016, 532(7598), 195–200 CrossRef CAS PubMed.
- K. A. Kigerl, J. C. Gensel, D. P. Ankeny, J. K. Alexander, D. J. Donnelly and P. G. Popovich, Identification of two distinct macrophage subsets with divergent effects causing either neurotoxicity or regeneration in the injured mouse spinal cord, J. Neurosci., 2009, 29(43), 13435–13444 CrossRef CAS PubMed.
- Y. Ma, J. Wang, Y. Wang and G. Y. Yang, The biphasic function of microglia in ischemic stroke, Prog. Neurobiol., 2017, 157, 247–272 CrossRef CAS PubMed.
- D. M. McTigue, P. Wei and B. T. Stokes, Proliferation of NG2-positive cells and altered oligodendrocyte numbers in the contused rat spinal cord, J. Neurosci., 2001, 21(10), 3392–3400 CrossRef CAS PubMed.
- X. He, Y. Li, H. Lu, Z. Zhang, Y. Wang and G. Y. Yang, Netrin-1 overexpression promotes white matter repairing and remodeling after
focal cerebral ischemia in mice, J. Cereb. Blood Flow Metab., 2013, 33(12), 1921–1927 CrossRef CAS PubMed.
- S. Liu, T. Schackel, N. Weidner and R. Puttagunta, Biomaterial-supported cell transplantation treatments for spinal cord injury: Challenges and perspectives, Front. Cell. Neurosci., 2017, 11, 430 CrossRef PubMed.
- D. D. Pearse and D. J. Barakat, Cellular repair strategies for spinal cord injury, Expert Opin. Biol. Ther., 2006, 6(7), 639–652 CrossRef CAS PubMed.
- A. M. Parr, I. Kulbatski, T. Zahir, X. Wang, C. Yue and A. Keating,
et al., Transplanted adult spinal cord-derived neural stem/progenitor cells promote early functional recovery after rat spinal cord injury, Neuroscience, 2008, 155(3), 760–770 CrossRef CAS PubMed.
- W. Zakrzewski, M. Dobrzynski, M. Szymonowicz and Z. Rybak, Stem cells: Past, present, and future, Stem Cell Res. Ther., 2019, 10(1), 68 CrossRef CAS PubMed.
- A. R. Massensini, H. Ghuman, L. T. Saldin, C. J. Medberry, T. J. Keane and F. J. Nicholls,
et al., Concentration-dependent rheological properties of ECM hydrogel for intracerebral delivery to a stroke cavity, Acta Biomater., 2015, 27, 116–130 CrossRef CAS PubMed.
- A. Mellati and J. Akhtari, Injectable hydrogels: A review of injectability mechanisms and biomedical applications, Res. Mol. Med., 2019, 1–14 Search PubMed.
- Y. Sun, D. Nan, H. Jin and X. Qu, Recent advances of injectable hydrogels for drug delivery and tissue engineering applications, Polym. Test., 2020, 81, 106283 CrossRef CAS.
- P. J. Kondiah, Y. E. Choonara, P. P. Kondiah, T. Marimuthu, P. Kumar and L. C. du Toit,
et al., A review of injectable polymeric hydrogel systems for application in bone tissue engineering, Molecules, 2016, 21(11), 1580 CrossRef PubMed.
- K. Y. Choi, H. S. Han, E. S. Lee, J. M. Shin, B. D. Almquist and D. S. Lee,
et al., Hyaluronic acid-based activatable nanomaterials for stimuli-responsive imaging and therapeutics: Beyond CD44-mediated drug delivery, Adv. Mater., 2019, e1803549 CrossRef PubMed.
- S. Naahidi, M. Jafari, M. Logan, Y. Wang, Y. Yuan and H. Bae,
et al., Biocompatibility of hydrogel-based scaffolds for tissue engineering applications, Biotechnol. Adv., 2017, 35(5), 530–544 CrossRef CAS PubMed.
- B. Saleh, H. K. Dhaliwal, R. Portillo-Lara, E. Shirzaei Sani, R. Abdi and M. M. Amiji,
et al., Local immunomodulation using an adhesive hydrogel loaded with miRNA-laden nanoparticles promotes wound healing, Small, 2019, e1902232 CrossRef PubMed.
- A. Gupta, S. M. Briffa, S. Swingler, H. Gibson, V. Kannappan and G. Adamus,
et al., Synthesis of silver nanoparticles using curcumin-cyclodextrins loaded into bacterial cellulose-based hydrogels for wound dressing applications, Biomacromolecules, 2020, 1802–1811 CrossRef CAS PubMed.
- X. Du, Y. Liu, X. Wang, H. Yan, L. Wang and L. Qu,
et al., Injectable hydrogel composed of hydrophobically modified chitosan/oxidized-dextran for wound healing, Mater. Sci. Eng., C, 2019, 104, 109930 CrossRef CAS PubMed.
- N. Annabi, D. Rana, E. Shirzaei Sani, R. Portillo-Lara, J. L. Gifford and M. M. Fares,
et al., Engineering a sprayable and elastic hydrogel adhesive with antimicrobial properties for wound healing, Biomaterials, 2017, 139, 229–243 CrossRef CAS PubMed.
- J. Li and G. Lepski, Cell transplantation for spinal cord injury: A systematic review, BioMed Res. Int., 2013, 2013, 786475 Search PubMed.
- L. M. Marquardt and S. C. Heilshorn, Design of injectable materials to improve stem cell transplantation, Curr. Stem Cell Rep., 2016, 2(3), 207–220 CrossRef PubMed.
- S. Budday, G. Sommer, C. Birkl, C. Langkammer, J. Haybaeck and J. Kohnert,
et al.,
Mechanical characterization of human brain tissue, Acta Biomater., 2017, 48, 319–340 CrossRef CAS PubMed.
- S. M. Willerth and S. E. Sakiyama-Elbert, Approaches to neural tissue engineering using scaffolds for drug delivery, Adv. Drug Delivery Rev., 2007, 59(4–5), 325–338 CrossRef CAS PubMed.
- D. Macaya, K. K. Ng and M. Spector, Injectable collagen-Genipin gel for the treatment of spinal cord injury: In vitro studies, Adv. Funct. Mater., 2011, 21(24), 4788–4797 CrossRef CAS.
- E. Ansorena, P. De Berdt, B. Ucakar, T. Simon-Yarza, D. Jacobs and O. Schakman,
et al., Injectable alginate hydrogel loaded with GDNF promotes functional recovery in a hemisection model of spinal cord injury, Int. J. Pharm., 2013, 455(1-2), 148–158 CrossRef CAS PubMed.
- M. C. Catoira, L. Fusaro, D. Di Francesco, M. Ramella and F. Boccafoschi, Overview of natural hydrogels for regenerative medicine applications, J. Mater. Sci.: Mater. Med., 2019, 30(10), 115 CrossRef PubMed.
- E. G. Norris, D. Dalecki and D. C. Hocking, Acoustic modification of collagen hydrogels facilitates cellular remodeling, Mater Today Bio, 2019, 3, 100018 CrossRef PubMed.
- K. Matsumoto, Y. Li, C. Jakuba, Y. Sugiyama, T. Sayo and M. Okuno,
et al., Conditional inactivation of Has2 reveals a crucial role for hyaluronan in skeletal growth, patterning, chondrocyte maturation and joint formation in the developing limb, Development, 2009, 136(16), 2825–2835 CrossRef CAS PubMed.
- H. Hamedi, S. Moradi, S. M. Hudson and A. E. Tonelli, Chitosan based hydrogels and their applications for drug delivery in wound dressings: A review, Carbohydr. Polym., 2018, 199, 445–460 CrossRef CAS PubMed.
- H. Li, Z. Qi, S. Zheng, Y. Chang, W. Kong and C. Fu,
et al., The application of hyaluronic acid-based hydrogels in bone and cartilage tissue engineering, Adv. Mater. Sci. Eng., 2019, 2019, 1–12 Search PubMed.
- C. E. Semino, Self-assembling peptides: From bio-inspired materials to bone regeneration, J. Dent. Res., 2008, 87(7), 606–616 CrossRef CAS PubMed.
- O. Steward, B. Zheng and M. Tessier-Lavigne, False resurrections: Distinguishing regenerated from spared axons in the injured central nervous system, J. Comp. Neurol., 2003, 459(1), 1–8 CrossRef PubMed.
- H. Nomura, Y. Katayama, M. S. Shoichet and C. H. Tator, Complete spinal cord transection treated by implantation of a reinforced synthetic hydrogel channel results in syringomyelia and caudal migration of the rostral stump, Neurosurgery, 2006, 53(1), 183–192 CrossRef PubMed.
- B. M. Gillette, J. A. Jensen, B. Tang, G. J. Yang, A. Bazargan-Lari and M. Zhong,
et al., In situ collagen assembly for integrating microfabricated three-dimensional cell-seeded matrices, Nat. Mater., 2008, 7(8), 636–640 CrossRef CAS PubMed.
- M. Tsintou, K. Dalamagkas and A. Seifalian, Injectable hydrogel versus plastically compressed collagen scaffold for central nervous system applications, Int. J. Biomater., 2018, 2018, 3514019 Search PubMed.
- M. M. Pakulska, B. G. Ballios and M. S. Shoichet, Injectable hydrogels for central nervous system therapy, Biomed. Mater., 2012, 7(2), 024101 CrossRef PubMed.
- E. Bellotti, A. L. Schilling, S. R. Little and P. Decuzzi, Injectable thermoresponsive hydrogels as drug delivery system for the treatment of central nervous system disorders: A review, J. Controlled Release, 2020, 329, 16–35 CrossRef PubMed.
- S. Bai, W. Zhang, Q. Lu, Q. Ma, D. L. Kaplan and H. Zhu, Silk nanofiber hydrogels with tunable modulus to regulate nerve stem cell fate, J. Mater. Chem. B, 2014, 2(38), 6590–6600 RSC.
- C. P. Addington, S. Dharmawaj, J. M. Heffernan, R. W. Sirianni and S. E. Stabenfeldt, Hyaluronic acid-laminin hydrogels increase neural stem cell transplant retention and migratory response to SDF-1alpha, Matrix Biol., 2017, 60–61, 206–216 CrossRef CAS PubMed.
- S. K. Seidlits, J. Liang, R. D. Bierman, A. Sohrabi, J. Karam and S. M. Holley,
et al., Peptide-modified, hyaluronic acid-based hydrogels as a 3D culture platform for neural stem/progenitor cell engineering, J. Biomed. Mater. Res. A, 2019, 107(4), 704–718 CrossRef CAS PubMed.
- K. H. Bae, L. S. Wang and M. Kurisawa, Injectable biodegradable hydrogels: Progress and challenges, J. Mater. Chem. B, 2013, 1(40), 5371–5388 RSC.
- P. van de Wetering, A. T. Metters, R. G. Schoenmakers and J. A. Hubbell, Poly(ethylene glycol) hydrogels formed by conjugate addition with controllable swelling, degradation, and release of pharmaceutically active proteins, J. Controlled Release, 2005, 102(3), 619–627 CrossRef CAS PubMed.
- M. Kurisawa, F. Lee, L.-S. Wang and J. E. Chung, Injectable enzymatically crosslinked hydrogel system with independent tuning of mechanical strength and gelation rate for drug delivery and tissue engineering, J. Mater. Chem., 2010, 20(26), 5371–5375 RSC.
- E. Verheyen, L. Delain-Bioton, S. van der Wal, N. el Morabit, A. Barendregt and W. E. Hennink,
et al., Conjugation of methacrylamide groups to a model protein via a reducible linker for immobilization and subsequent triggered release from hydrogels, Macromol. Biosci., 2010, 10(12), 1517–1526 CrossRef CAS PubMed.
- J. Lee, C. Y. Tan, S. K. Lee, Y. H. Kim and K. Y. Lee, Controlled delivery of heat shock protein using an injectable microsphere/hydrogel combination system for the treatment of myocardial infarction, J. Controlled Release, 2009, 137(3), 196–202 CrossRef CAS PubMed.
- B. Soontornworajit, J. Zhou, Z. Zhang and Y. Wang, Aptamer-functionalized in situ injectable hydrogel for controlled protein release, Biomacromolecules, 2010, 11(10), 2724–2730 CrossRef CAS PubMed.
- M. P. Lutolf, G. P. Raeber, A. H. Zisch, N. Tirelli and J. A. Hubbell, Cell-responsive synthetic hydrogels, Adv. Mater., 2003, 15(11), 888–892 CrossRef CAS.
- K. L. Niece, J. D. Hartgerink, J. J. J. M. Donners and S. I. Stupp, Self-assembly combining two bioactive peptide-amphiphile molecules into nanofibers by electrostatic attraction, J. Am. Chem. Soc., 2003, 125(24), 7146–7147 CrossRef CAS.
- G. A. Silva, C. Czeisler, K. L. Niece, E. Beniash, D. A. Harrington and J. A. Kessler,
et al., Selective differentiation of neural progenitor cells by high-epitope density nanofibers, Science, 2004, 303, 1352–1355 CrossRef CAS PubMed.
- M. C. Jin, Z. A. Medress, T. D. Azad, V. M. Doulames and A. Veeravagu, Stem cell therapies for acute spinal cord injury in humans: A review, Neurosurg. Focus, 2019, 46(3), E10 Search PubMed.
- S. L. Payne, A. Tuladhar, J. M. Obermeyer, B. V. Varga, C. J. Teal and C. M. Morshead,
et al., Initial cell maturity changes following transplantation in a hyaluronan-based hydrogel and impacts therapeutic success in the stroke-injured rodent brain, Biomaterials, 2019, 192, 309–322 CrossRef CAS.
- P. Moshayedi, L. R. Nih, I. L. Llorente, A. R. Berg, J. Cinkornpumin and W. E. Lowry,
et al., Systematic optimization of an engineered hydrogel allows for selective control of human neural stem cell survival and differentiation after transplantation in the stroke brain, Biomaterials, 2016, 105, 145–155 CrossRef CAS PubMed.
- M. R. McCrary, K. Jesson, Z. Z. Wei, M. Logun, C. Lenear and S. Tan,
et al., Cortical transplantation of brain-mimetic glycosaminoglycan scaffolds and neural progenitor cells promotes vascular regeneration and functional recovery after ischemic stroke in mice, Adv. Healthcare Mater., 2020, 9(5), e1900285 CrossRef PubMed.
- T. Osanai, S. Kuroda, H. Yasuda, Y. Chiba, K. Maruichi and M. Hokari,
et al., Noninvasive transplantation of bone marrow stromal cells for ischemic stroke: Preliminary study with a thermoreversible gelation polymer hydrogel, Neurosurgery, 2010, 66(6), 1140–1147 CrossRef ; discussion 7.
- I. Osama, N. Gorenkova, C. M. McKittrick, T. Wongpinyochit, A. Goudie and F. P. Seib,
et al., In vitro studies on space-conforming self-assembling silk hydrogels as a mesenchymal stem cell–support matrix suitable for minimally invasive brain application, Sci. Rep., 2018, 8(1), 13655 CrossRef CAS PubMed.
- L. Xu, J. Ryu, H. Hiel, A. Menon, A. Aggarwal and E. Rha,
et al., Transplantation of human oligodendrocyte progenitor cells in an animal model of diffuse traumatic axonal injury: Survival and differentiation, Stem Cell Res. Ther., 2015, 6, 93 CrossRef.
- P. J. Reddig and R. L. Juliano, Clinging to life cell to matrix adhesion and cell survival, Cancer Metastasis Rev., 2005, 24, 425–439 CrossRef.
- F. Barnabe-Heider and J. Frisen, Stem cells for spinal cord repair, Cell Stem Cell, 2008, 3(1), 16–24 CrossRef CAS.
- S. Tavakol, R. Saber, E. Hoveizi, H. Aligholi, J. Ai and S. M. Rezayat, Chimeric self-assembling nanofiber containing bone marrow homing peptide's motif induces motor neuron recovery in animal model of chronic spinal cord injury; an in vitro and in vivo investigation, Mol. Neurobiol., 2016, 53, 3298–3308 CrossRef CAS PubMed.
- Z. Wang, J. Wang, Y. Jin, Z. Luo, W. Yang and H. Xie,
et al., A neuroprotective sericin hydrogel as an effective neuronal cell carrier for the repair of ischemic stroke, ACS Appl. Mater. Interfaces, 2015, 7(44), 24629–24640 CrossRef CAS PubMed.
- N. Rouleau, W. L. Cantley, V. Liaudanskaya, A. Berk, C. Du and W. Rusk,
et al., A long-living bioengineered neural tissue platform to study neurodegeneration, Macromol. Biosci., 2020, 20(3), e2000004 CrossRef.
- M. Naruse, K. Shibasaki, S. Yokoyama, M. Kurachi and Y. Ishizaki, Dynamic changes of CD44 expression from progenitors to subpopulations of astrocytes and neurons in developing cerebellum, PLoS One, 2013, 8(1), e53109 CrossRef CAS.
- A. Konopka, A. Zeug, A. Skupien, B. Kaza, F. Mueller and A. Chwedorowicz,
et al., Cleavage of hyaluronan and CD44 adhesion molecule regulate astrocyte morphology via Rac1 signalling, PLoS One, 2016, 11(5), e0155053 CrossRef.
- R. Sawada, A. Nakano-Doi, T. Matsuyama, N. Nakagomi and T. Nakagomi, CD44 expression in stem cells and niche microglia/macrophages following ischemic stroke, Stem Cell Investig., 2020, 7, 4 CrossRef CAS PubMed.
- T. Fujimoto, H. Kawashima, T. Tanaka, M. Hirose, N. Toyama-Sorimachi and Y. Matsuzawa,
et al., CD44 binds a chondrotin sulfate proteoglycan, aggrecan, Int. Immunol., 2001, 13, 359–366 CrossRef CAS PubMed.
- M. S. Kurtis, B. P. Tu, O. A. Gaya, J. Mollenhauer, W. Knudson, R. F. Loeser, C. B. Knudson and R. L. Sah, Mechanisms of chondrocyte adhesion to cartilage role of pl-integrins, CD44, and annexin V, J. Orthopaed. Res., 2001, 19(6), 1122–1130 CrossRef CAS.
- S. Koutsopoulos and S. Zhang, Long-term three-dimensional neural tissue cultures in functionalized self-assembling peptide hydrogels, matrigel and collagen I, Acta Biomater., 2013, 9(2), 5162–5169 CrossRef CAS PubMed.
- H. Ponta, L. Sherman and P. A. Herrlich, CD44: From adhesion molecules to signalling regulators, Nat. Rev. Mol. Cell Biol., 2003, 4(1), 33–45 CrossRef CAS.
- M. J. Caicco, T. Zahir, A. J. Mothe, B. G. Ballios, A. J. Kihm and C. H. Tator,
et al., Characterization of hyaluronan-methylcellulose hydrogels for cell delivery to the injured spinal cord, J. Biomed. Mater. Res. A, 2013, 101(5), 1472–1477 CrossRef PubMed.
- R. Ju, Y. Wen, R. Gou, Y. Wang and Q. Xu, The experimental therapy on brain ischemia by improvement of local angiogenesis with tissue engineering in the mouse, Cell Transplant., 2014, 23(Suppl
1), S83–S95 Search PubMed.
- J. Qu and H. Zhang, Roles of mesenchymal stem cells in spinal cord injury, Stem Cells Int., 2017, 2017, 5251313 Search PubMed.
- A. Shao, S. Tu, J. Lu and J. Zhang, Crosstalk between stem cell and spinal cord injury: Pathophysiology and treatment strategies, Stem Cell Res. Ther., 2019, 10(1), 238 CrossRef PubMed.
- T. Yamashita and K. Abe, Recent progress in therapeutic strategies for ischemic stroke, Cell Transplant., 2016, 25(5), 893–898 Search PubMed.
- A. J. Mothe, R. Y. Tam, T. Zahir, C. H. Tator and M. S. Shoichet, Repair of the injured spinal cord by transplantation of neural stem cells in a hyaluronan-based hydrogel, Biomaterials, 2013, 34(15), 3775–3783 CrossRef CAS PubMed.
- T. H. Perera, X. Lu, S. M. Howell, Y. E. Kurosu and L. A. Smith Callahan, Combination of IKVAV, LRE, and GPQGIWGQ bioactive signaling peptides increases human induced pluripotent stem cell derived neural stem cells extracellular matrix remodeling and neurite extension, Adv. BioSyst., 2020, e2000084 CrossRef PubMed.
- S. A. Geissler, A. L. Sabin, R. R. Besser, O. M. Gooden, B. D. Shirk and Q. M. Nguyen,
et al., Biomimetic hydrogels direct spinal progenitor cell differentiation and promote functional recovery after spinal cord injury, J. Neural Eng., 2018, 15(2), 025004 CrossRef PubMed.
- J. Nicolas, S. Magli, L. Rabbachin, S. Sampaolesi, F. Nicotra and L. Russo, 3D Extracellular matrix mimics: Fundamental concepts and role of materials chemistry to influence stem cell fate, Biomacromolecules, 2020, 21(6), 1968–1994 CrossRef CAS PubMed.
- R. Patel, M. Santhosh, J. K. Dash, R. Karpoormath, A. Jha and J. Kwak,
et al., Ile-Lys-Val-ala-Val (IKVAV) peptide for neuronal tissue engineering, Polym. Adv. Technol., 2019, 30(1), 4–12 CrossRef CAS.
- F. Tabatabaei, Z. Aghamohammadi and L. Tayebi,
In vitro and in vivo effects of concentrated growth factor on cells and tissues, J. Biomed. Mater. Res. A, 2020, 108(6), 1338–1350 CrossRef CAS PubMed.
- A. Younsi, G. Zheng, M. Scherer, L. Riemann, H. Zhang and M. Tail,
et al., Three growth factors induce proliferation and differentiation of neural precursor cells in vitro and support cell-transplantation after spinal cord injury in vivo, Stem Cells Int., 2020, 2020, 10046–10064 Search PubMed.
- G. A. Silva, C. Czeisler, K. L. Niece, E. Beniash, D. A. Harrington and J. A. Kessler,
et al., Selective differentiation of neural progenitor cells by high-epitope density nanofibers, Science, 2004, 303(5662), 1352–1355 CrossRef CAS PubMed.
- N. Mukherjee, A. Adak and S. Ghosh, Recent trends in the development of peptide and protein-based hydrogel therapeutics for the healing of CNS injury, Soft Matter, 2020, 10046–10064 RSC.
- W. Sun, T. Incitti, C. Migliaresi, A. Quattrone, S. Casarosa and A. Motta, Viability and neuronal differentiation of neural stem cells encapsulated in silk fibroin hydrogel functionalized with an IKVAV peptide, J. Tissue Eng. Regen. Med., 2017, 11(5), 1532–1541 CrossRef CAS PubMed.
- L. Fan, C. Liu, X. Chen, Y. Zou, Z. Zhou and C. Lin,
et al., Directing induced pluripotent stem cell derived neural stem cell fate with a three-dimensional biomimetic hydrogel for spinal cord injury repair, ACS Appl. Mater. Interfaces, 2018, 10(21), 17742–17755 CrossRef CAS PubMed.
- D. Ye and A. Peramo, Implementing tissue engineering and regenerative medicine solutions in medical implants, Br. Med. Bull., 2014, 109, 3–18 CrossRef PubMed.
- H. Altinova, S. Hammes, M. Palm, P. Achenbach, J. Gerardo-Nava and R. Deumens,
et al., Dense fibroadhesive scarring and poor blood vessel-maturation hamper the integration of implanted collagen scaffolds in an experimental model of spinal cord injury, Biomed. Mater., 2020, 15(1), 015012 CrossRef CAS PubMed.
- D. Tukmachev, S. Forostyak, Z. Koci, K. Zaviskova, I. Vackova and K. Vyborny,
et al., Injectable extracellular matrix hydrogels as scaffolds for spinal cord injury repair, Tissue Eng., Part A, 2016, 22(3–4), 306–317 CrossRef CAS PubMed.
- V. R. King, A. Alovskaya, D. Y. Wei, R. A. Brown and J. V. Priestley, The use of injectable forms of fibrin and fibronectin to support axonal ingrowth after spinal cord injury, Biomaterials, 2010, 31(15), 4447–4456 CrossRef CAS PubMed.
- Y. Liu, H. Ye, K. Satkunendrarajah, G. S. Yao, Y. Bayon and M. G. Fehlings, A self-assembling peptide reduces glial scarring, attenuates post-traumatic inflammation and promotes neurological recovery following spinal cord injury, Acta Biomater., 2013, 9(9), 8075–8088 CrossRef CAS PubMed.
- L. R. Nih, E. Sideris, S. T. Carmichael and T. Segura, Injection of microporous annealing particle (MAP) hydrogels in the stroke cavity reduces gliosis and inflammation and promotes NPC migration to the lesion, Adv. Mater., 2017, 29(32), 1606471 CrossRef PubMed.
- L. T. A. Hong, Y. M. Kim, H. H. Park, D. H. Hwang, Y. Cui and E. M. Lee,
et al., An injectable hydrogel enhances tissue repair after spinal cord injury by promoting extracellular matrix remodeling, Nat. Commun., 2017, 8(1), 533 CrossRef PubMed.
- A. des Rieux, P. De Berdt, E. Ansorena, B. Ucakar, J. Damien and O. Schakman,
et al., Vascular endothelial growth factor-loaded injectable hydrogel enhances plasticity in the injured spinal cord, J. Biomed. Mater. Res. A, 2014, 102(7), 2345–2355 CrossRef PubMed.
- H. Ghuman, M. Gerwig, F. J. Nicholls, J. R. Liu, J. Donnelly and S. F. Badylak,
et al., Long-term retention of ECM hydrogel after implantation into a sub-acute stroke cavity reduces lesion volume, Acta Biomater., 2017, 63, 50–63 CrossRef CAS PubMed.
- E. A. Huebner and S. M. Strittmatter, Axon regeneration in the peripheral and central nervous systems, Res. Probl. Cell Differ., 2009, 48, 339–351 CAS.
- K. E. Dow, S. E. Mirski, J. C. Roder and R. J. Riopelle, Neuronal proteoglycans: Biosynthesis and functional interaction with neurons in vitro, J. Neurosci., 1988, 8(9), 3278–3289 CrossRef CAS PubMed.
- C. Darian-Smith, Synaptic plasticity, neurogenesis, and functional recovery after spinal cord injury, Neuroscientist, 2009, 15(2), 149–165 CrossRef PubMed.
- F. A. Somaa, T. Y. Wang, J. C. Niclis, K. F. Bruggeman, J. A. Kauhausen and H. Guo,
et al., Peptide-based scaffolds support human cortical progenitor graft integration to reduce atrophy and promote functional repair in a model of stroke, Cell Rep., 2017, 20(8), 1964–1977 CrossRef CAS PubMed.
- Y. Wang, M. J. Cooke, C. M. Morshead and M. S. Shoichet, Hydrogel delivery of erythropoietin to the brain for endogenous stem cell stimulation after stroke injury, Biomaterials, 2012, 33(9), 2681–2692 CrossRef CAS PubMed.
- Q. Wang, Y. He, Y. Zhao, H. Xie, Q. Lin and Z. He,
et al., A thermosensitive heparin-poloxamer hydrogel bridges aFGF to treat spinal cord injury, ACS Appl. Mater. Interfaces, 2017, 9(8), 6725–6745 CrossRef CAS PubMed.
- V. Gupta, D. V. Lyne, A. D. Laflin, T. A. Zabel, M. Barragan and J. T. Bunch,
et al., Microsphere-based osteochondral scaffolds carrying opposing gradients of decellularized cartilage and demineralized bone matrix, ACS Biomater. Sci. Eng., 2017, 3(9), 1955–1963 CrossRef CAS PubMed.
- C. R. Rowland, K. A. Glass, A. R. Ettyreddy, C. C. Gloss, J. R. L. Matthews and N. P. T. Huynh,
et al., Regulation of decellularized tissue remodeling via scaffold-mediated lentiviral delivery in anatomically-shaped osteochondral constructs, Biomaterials, 2018, 177, 161–175 CrossRef CAS PubMed.
- L. Sang, Y. Liu, W. Hua, K. Xu, G. Wang and W. Zhong,
et al., Thermally sensitive conductive hydrogel using amphiphilic crosslinker self-assembled carbon nanotube to enhance neurite outgrowth and promote spinal cord regeneration, RSC Adv., 2016, 6(31), 26341–26351 RSC.
- R. C. Cornelison, E. J. Gonzalez-Rothi, S. L. Porvasnik, S. M. Wellman, J. H. Park and D. D. Fuller,
et al., Injectable hydrogels of optimized acellular nerve for injection in the injured spinal cord, Biomed. Mater., 2018, 13(3), 034110 CrossRef PubMed.
- H. Ghuman, C. Mauney, J. Donnelly, A. R. Massensini, S. F. Badylak and M. Modo, Biodegradation of ECM hydrogel promotes endogenous brain tissue restoration in a rat model of stroke, Acta Biomater., 2018, 80, 66–84 CrossRef CAS PubMed.
- L. H. Nguyen, M. Gao, J. Lin, W. Wu, J. Wang and S. Y. Chew, Three-dimensional aligned nanofibers-hydrogel scaffold for controlled non-viral drug/gene delivery to direct axon regeneration in spinal cord injury treatment, Sci. Rep., 2017, 7(1), 42212 CrossRef CAS PubMed.
- P. Lu, K. Takai, V. M. Weaver and Z. Werb, Extracellular matrix degradation and remodeling in development and disease, Cold Spring Harb. Perspect. Biol., 2011, 3(12), a005058 Search PubMed.
- G. A. Hudalla, T. S. Eng and W. L. Murphy, An approach to modulate degradation and mesenchymal stem cell behavior in poly(ethylene glycol) networks, Biomacromolecules, 2008, 9(3), 842–849 CrossRef CAS.
- K. Ravina, D. I. Briggs, S. Kislal, Z. Warraich, T. Nguyen and R. K. Lam,
et al., Intracerebral delivery of brain-derived neurotrophic factor using HyStem((R))-C hydrogel implants improves functional recovery and reduces neuroinflammation in a rat model of ischemic stroke, Int. J. Mol. Sci., 2018, 19(12), 3782 CrossRef PubMed.
- D. J. Cook, C. Nguyen, H. N. I. L. L. Chun, A. S. Chiu and M. Machnicki,
et al., Hydrogel-delivered brain-derived neurotrophic factor promotes tissue repair and recovery after stroke, J. Cereb. Blood Flow Metab., 2017, 37(3), 1030–1045 CrossRef CAS PubMed.
- A. A. Foster, L. M. Marquardt and S. C. Heilshorn, The diverse roles of hydrogel mechanics in injectable stem cell transplantation, Curr. Opin. Chem. Eng., 2017, 15, 15–23 CrossRef.
- C. G. Liew, H. Moore, L. Ruban, N. Shah, K. Cosgrove and M. Dunne,
et al., Human embryonic stem cells: Possibilities for human cell transplantation, Ann. Med., 2005, 37(7), 521–532 CrossRef CAS PubMed.
- M. F. Azari, L. Mathias, E. Ozturk, D. S. Cram, R. L. Boyd and S. Petratos, Mesenchymal stem cells for treatment of CNS injury, Curr. Neuropharmacol., 2010, 8(4), 316–323 CrossRef CAS PubMed.
- M. G. Cefalo, A. Carai, E. Miele, A. Po, E. Ferretti and A. Mastronuzzi,
et al., Human iPSC for therapeutic approaches to the nervous system: Present and future applications, Stem Cells Int., 2016, 2016, 4869071 Search PubMed.
- G. W. Hawryluk, A. Mothe, J. Wang, S. Wang, C. Tator and M. G. Fehlings, An in vivo characterization of trophic factor production following neural precursor cell or bone marrow stromal cell transplantation for spinal cord injury, Stem Cells Dev., 2012, 21(12), 2222–2238 CrossRef CAS PubMed.
- C. Lutton, Y. W. Young, R. Williams, A. C. Meedeniya, A. Mackay-Sim and B. Goss, Combined VEGF and PDGF treatment reduces secondary degeneration after spinal cord injury, J. Neurotrauma, 2012, 29(5), 957–970 CrossRef PubMed.
- M. V. Sofroniew, Molecular dissection of reactive astrogliosis and glial scar formation, Trends Neurosci., 2009, 32(12), 638–647 CrossRef CAS PubMed.
- S. Karimi-Abdolrezaee and R. Billakanti, Reactive astrogliosis after spinal cord injury-beneficial and detrimental effects, Mol. Neurobiol., 2012, 46(2), 251–264 CrossRef CAS PubMed.
- G. Yiu and Z. He, Glial inhibition of CNS axon regeneration, Nat. Rev. Neurosci., 2006, 7(8), 617–627 CrossRef CAS PubMed.
- A. Rolls, R. Shechter and M. Schwartz, The bright side of the glial scar in CNS repair, Nat. Rev. Neurosci., 2009, 10(3), 235–241 CrossRef CAS PubMed.
- V. Gallo and B. Deneen, Glial development: The crossroads of regeneration and repair in the CNS, Neuron, 2014, 83(2), 283–308 CrossRef CAS PubMed.
- D. Gupta, C. H. Tator and M. S. Shoichet, Fast-gelling injectable blend of hyaluronan and methylcellulose for intrathecal, localized delivery to the injured spinal cord, Biomaterials, 2006, 27(11), 2370–2379 CrossRef CAS PubMed.
- K. Anderson, S. Aito, M. Atkins, F. Biering-Sørensen, S. Charlifue and A. Curt,
et al., Functional recovery measures for spinal cord injury an evidence-based review for clinical practice and research, J. Spinal Cord Med., 2008, 31(2), 133–144 CrossRef PubMed.
- W. S. Redfern, A. Dymond, I. Strang, S. Storey, C. Grant and L. Marks,
et al., The functional observational battery and modified Irwin test as global neurobehavioral assessments in the rat: Pharmacological validation data and a comparison of methods, J. Pharmacol. Toxicol. Methods, 2019, 98, 106591 CrossRef CAS PubMed.
- R. U. Ahmed, M. Alam and Y. P. Zheng, Experimental spinal cord injury and behavioral tests in laboratory rats, Heliyon, 2019, 5(3), e01324 CrossRef PubMed.
- M. R. Detloff, L. C. Fisher, R. J. Deibert and D. M. Basso, Acute and chronic tactile sensory testing after spinal cord injury in rats, J. Vis. Exp., 2012, 62, e3247 Search PubMed.
- K. Gale, H. Kerasidis and J. R. Wrathall, Spinal cord contusion in the rat Behavioral analysis of functional neurologic impairment, Exp. Neurol., 1985, 88(1), 123–134 CrossRef CAS.
- W. Yu, J. X. Hao, X. J. Xu, J. Saydoff, A. Haegerstrand and T. Hökfelt,
et al., Long-term alleviation of allodynia-like behaviors by intrathecal implantation of bovine chromaffin cells in rats with spinal cord injury, Pain, 1998, 74(2–3), 115–122 CrossRef CAS PubMed.
- D. M. Basso, M. S. Beattie and J. C. Bresnahan, A sensitive and reliable locomotor rating scale for open field testing in rats, J. Neurotrauma, 1995, 12(1), 1–21 CrossRef CAS PubMed.
- G. Laviola, G. Renna, G. Bignami and V. Cuomo, Ontogenetic and pharmacological dissociation of various components of locomotor activity and habituation in the rat, Int. J. Dev. Neurosci., 1988, 6(5), 431–438 CrossRef CAS.
- L. Prut and C. Belzung, The open field as a paradigm to measure the effects of drugs on anxiety-like behaviors: A review, Eur. J. Pharmacol., 2003, 463(1–3), 3–33 CrossRef CAS.
- S. H. Wang, Z. J. Zhang, Y. J. Guo, G. J. Teng and B. A. Chen, Hippocampal neurogenesis and behavioural studies on adult ischemic rat response to chronic mild stress, Behav. Brain Res., 2008, 189(1), 9–16 CrossRef PubMed.
- A. Sarkaki, Y. Farbood, M. Badavi, L. Khalaj, F. Khodagholi and G. Ashabi, Metformin improves anxiety-like behaviors through AMPK-dependent regulation of autophagy following transient forebrain ischemia, Metab. Brain Dis., 2015, 30(5), 1139–1150 CrossRef CAS.
- N. Xu, E. Akesson, L. Holmberg and E. Sundstrom, A sensitive and reliable test instrument to assess swimming in rats with spinal cord injury, Behav. Brain Res., 2015, 291, 172–183 CrossRef.
- J. Ruan and Y. Yao, Behavioral tests in rodent models of stroke, Brain Hemorrhages, 2020, 1(4), 171–184 CrossRef.
- M. W. McDonald, S. E. Black, D. A. Copland, D. Corbett, R. M. Dijkhuizen and T. D. Farr,
et al., Cognition in stroke rehabilitation and recovery research: Consensus-based core recommendations from the second Stroke Recovery and Rehabilitation Roundtable, Int. J. Stroke, 2019, 14(8), 774–782 CrossRef PubMed.
- R. G. M. Morris, Spatial localization does not require the presence of local cues, Learn. Motivat., 1981, 12(2), 239–260 CrossRef.
- L. Kokkinidis, M. D. Walsh, R. Lahue and H. Anisman, Tolerance to d-amphetamine Behavioral specificity, Life Sci., 1976, 18(9), 913–917 CrossRef CAS PubMed.
- J. P. Aggleton, One-trial object recognition by rats, Q. J. Exp. Psychol. Sect. B, 1985, 37(4), 279–294 CrossRef.
- D. S. Olton, C. Collison and M. A. Werz, Spatial memory and radial arm maze performance of rats, Learn. Motivat., 1977, 8(3), 289–314 CrossRef.
- A. Patar, P. Dockery, S. McMahon and L. Howard,
Ex vivo rat transected spinal cord slices as a model to assess lentiviral vector delivery of neurotrophin-3 and short hairpin RNA against NG2, Biology, 2020, 9(3), 54 CrossRef CAS PubMed.
- A. P. Haring, H. Sontheimer and B. N. Johnson, Microphysiological human brain and neural systems-on-a-chip: Potential alternatives to small animal models and emerging platforms for drug discovery and personalized medicine, Stem Cell Rev. Rep., 2017, 13(3), 381–406 CrossRef CAS PubMed.
|
This journal is © The Royal Society of Chemistry 2021 |
Click here to see how this site uses Cookies. View our privacy policy here.