DOI:
10.1039/D1MA00829C
(Paper)
Mater. Adv., 2021,
2, 7773-7787
Indoor light-harvesting dye-sensitized solar cells surpassing 30% efficiency without co-sensitizers†
Received
9th September 2021
, Accepted 5th October 2021
First published on 5th October 2021
Abstract
Dye-sensitized solar cells (DSCs) have proven to be one of the best photovoltaic approaches for harnessing indoor/artificial light. Herein, we report two new molecularly engineered, cost-effective, metal-free, carbazole-based D–π–A sensitizers (YK 8 and YK 9) by judiciously varying their π-spacers, which are suitable for indoor photovoltaic applications. Using YK 8, we achieved an efficiency of 28.7% under standard 1000 lux Osram 14 W T2 cool day light fluorescent tube illumination with a power output of 68.88 μW cm−2 and 30.24% with a power output of 108.85 μW cm−2 under a higher illumination intensity of 1500 lux without co-sensitizers using iodide/triiodide electrolyte. With a small footprint/active area of 1.24 cm2, we could power a temperature sensor completely autonomously at a low light intensity of 500 lux, displaying the potential of these indoor photovoltaic devices to serve as energy sources for low-power sensors and actuators on the Internet of Things (IoT) network, reducing the dependence on batteries and leading to a smaller carbon footprint. The role of the π-spacer and its influence on recombination and device performance were explored via extensive interfacial studies.
1. Introduction
During the past decade, the energy demand and environmental issues associated with the overexploitation of fossil fuels have led to the quest for alternative renewable energy sources. Harnessing solar energy occupies a prominent position as it offers a greener and sustainable way of meeting the energy needs of modern society with a low environmental impact.1–3 With the emergence of the fourth industrial revolution (Industry 4.0), there is rapid growth (billions) in the application of remote sensors, actuators, and communication devices under the IoT ecosystem powered by batteries, which in turn limits the large-scale deployment of IoT.4,5 The use of batteries involves significant constraints due to their limited life-span, added operational and maintenance costs, and especially the environmental issues resulting from used batteries.6,7 These problems have triggered the development of indoor photovoltaics (IPVs).7–10 IPVs are devices that are capable of harvesting artificial light in indoor space and converting it to useful power, which can be used to transform conventional IoT power nodes into energy-independent self-powered systems.11–19 Among the various photovoltaic technologies, dye-sensitized solar cells (DSCs) have emerged as one of the leading candidates for power generation under indoor light conditions.20–23 Their simple design, cost-effectiveness, and ability to maintain high photovoltages in indoor/artificial light conditions make DSCs stand out among IPVs. Using conventional iodide/triiodide electrolyte with organic anthracene-based sensitizers, Tingare et al. achieved a PCE of 24.43% under 1200 lux and 28.56% under 6000 lux, which is the best-reported performance under indoor light conditions to date.24 In 2017, Freitag et al. demonstrated the capability of DSCs in artificial lighting environments with a power conversion efficiency of 28.9% under 1000 lux CFL using copper electrolyte.25 This was followed by contributions from Michael Grätzel and Anders Hagfeldt, proving this to be superior technology among the existing first- and second-generation photovoltaics for indoor light-harvesting applications. They realized an efficiency of 32% (1000 lux CFL) with copper electrolyte, opening new avenues to commercialise this technology.22 Grätzel and co-workers also achieved an efficiency of 28.4% under 1000 lux fluorescent light tube illumination using an organic dye with the same copper electrolyte.26 Recent reports have recorded efficiencies greater than 34% with the co-sensitization of organic dyes together with copper electrolyte.21,27
In DSCs, dyes/sensitizers occupy a prominent place in determining the PCE by converting incident light to electricity.8,28–37 With the present advancements in IPV, precisely engineered sensitizers having a good spectral match with indoor/artificial lights (LED/CFL) are prerequisites to achieve improved PCE under indoor/artificial light conditions. Although the conventional ruthenium (Ru) sensitizers have been proven to be the best candidates for sunlight harvesting, they are not suitable for power generation under indoor light. Additionally, the high cost, toxicity, scarcity and low flexibility to impart structural variation limit the use of these metal complexes in IPV. Thus, metal-free organic dyes were introduced to replace metal complex sensitizers by virtue of their low cost and better molecular design possibilities. In general, metal-free organic dyes adopt the donor–π bridge–acceptor (D–π–A) design, enabling superior charge separation, high molar extinction coefficient, easy bandgap engineering and good photostability.38–41 Diphenylamines, triarylamines, carbazoles, porphyrins and indoles have been used as potential organic sensitizers, among which carbazoles occupy a prominent position due to their high electron-donating capability and improved photo and thermal stability.29,35,36,39,42–53 DSCs fabricated with one of the well-studied commercial and efficient carbazole dye MK 2, having regioregular n-hexyl-substituted quarter-thiophene and cyanoacrylic acid resulted in 8.3% power conversion efficiency under 1 sun condition.54 Later, Kakiage and co-workers demonstrated ∼14.5% efficiency employing MK 2 together with a cocktail mixture of metal-free sensitizers.42 In this context, we designed and synthesized two carbazole dyes with variable π-spacers (YK 8 and YK 9). The YK 8 sensitizer is endowed with bis-octyl chains on a terthiophene core to reduce its aggregation and prevent carrier recombination, whereas the YK 9 dye was designed and synthesized using a more planar thienothiophene linker for better donor–acceptor interactions, facilitating rapid electron migration from the donor to acceptor. Our motivation was to introduce a simple D–π–A sensitizer capable of delivering >30% efficiency under fluorescent tube illumination in a cell structure without co-sensitizers using the conventional iodide/triiodide electrolyte.
2. Results and discussion
2.1. Synthesis of dyes
The dyes used herein possess the general architecture of D–π–A (Fig. 1a). The YK 8 and YK 9 dyes consist of N-methylcarbazole as the donor and cyanoacrylic acid as the acceptor and anchoring moiety. Structurally, YK 8 and YK 9 differ mainly in terms of the π-bridge, wherein the former contains bis-octyl-substituted terthiophene and the latter has a thienothiophene linked to one octyl-substituted thiophene unit. The synthetic strategy adopted for the preparation of YK 8 and YK 9 is presented in Fig. 2. Both dyes were obtained in 5–6 steps in good yields (Fig. 2), which promise their cost-effective large-scale production. To prepare YK 8, initially Stille coupling reaction of 2,5-bis(tributylstannyl)thiophene with 2-bromo-3-octylthiophene was performed to yield dioctyl-2,2′:5′,2′′-terthiophene (1), which was then converted to 3 through the Vilsmeier formylation reaction and bromination with NBS. Compound 3 was then converted to YK 8 through successive Suzuki coupling with 9-methyl-9H-carbazole-3-boronic acid pinacol ester and condensation reaction with cyanoacetic acid. Conversely, for the preparation of YK 9, intermediates 5 and 6 were prepared separately and coupled via Suzuki reaction to yield the first compound 7, which was subsequently brominated to produce 8. Similar to YK 8, YK 9 was obtained from 8 through successive Suzuki coupling with 9-methyl-9H-carbazole-3-boronic acid pinacol ester and condensation reaction with cyanoacetic acid.
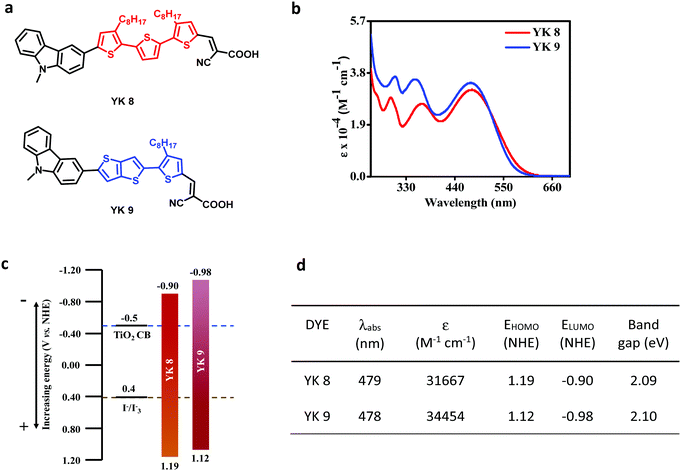 |
| Fig. 1 (a) Structure of the YK 8 and YK 9 dyes. (b) Absorption spectra of the YK 8 and YK 9 dyes in dichloromethane. (c) Energy level diagram of the YK 8 and YK 9 dyes illustrating the position of the HOMO with respect to the redox potential of the I−/I3− electrolyte and LUMO with respect to the TiO2 conduction band. (d) Optical and electrochemical parameters of the YK 8 and YK 9 dyes. | |
 |
| Fig. 2 Synthetic pathway for the YK 8 and YK 9 dyes (a) toluene, P(o-tolyl)3, Pd2(dba)3, and 2-bromo-3-octylthiophene, (b) DCE, DMF, and POCl3, (c) DMF and NBS, (d) toluene, 9-methyl-9H-carbazole-3-boronic acid pinacol ester, K2CO3, and Pd(PPh3)4, (e) CHCl3, cyanoacetic acid, piperidine, (f) THF, n-BuLi, and 2-isopropoxy-4,4,5,5-tetramethyl-4,3,2-dioxaboralane, (g) toluene, K2CO3, and Pd(PPh3)4, and (h) chloroform:acetic acid and NBS. | |
2.2. Optical and electrochemical properties
As expected, the variation in π-architecture did not have any profound effect on the spectral profiles of the dyes. Both dyes exhibited broad absorption in the range of 250–600 nm with three distinguishable peaks (Fig. 1b). The lower energy absorption is mainly due to the intramolecular charge transfer transitions, whereas the two higher energy peaks arise predominantly from the π–π* transition. It should be noted that YK 9 exhibited a slightly higher molar extinction coefficient (ε@478 nm ≈ 3.4 × 104 M−1 cm−1) than YK 8 (ε@479 nm ≈ 3.2 × 104 M−1 cm−1) in the blue region. Also, YK 8 showed a slightly red-shifted absorbance and higher molar extinction coefficient in the red region above 500 nm. To ensure effective electron transfer from the dye to the semiconductor and the regeneration of the dyes by the electrolyte, it is important to determine the ground and excited state energy levels of the sensitizers used in DSCs. Hence, we examined the oxidation potentials of the YK 8 and YK 9 dye molecules using cyclic voltammetry techniques (linear cyclic voltammetry and square wave voltammetry) in dichloromethane solvent with tetrabutylammonium hexafluorophosphate ((n-Bu)4NPF6) as the supporting electrolyte, glassy carbon as working electrode, Pt as the counter electrode and Ag/AgCl as the reference electrode. The reference electrode was calibrated using ferrocene as the standard. The cyclic voltammograms and the energy level calculations are provided in Fig. S1(a–c) (ESI†). Although both dyes exhibited quite similar band gaps (∼2.1 eV), the HOMO and LUMO energy levels of YK 9 are positioned slightly above that of YK 8 (Fig. 1c and d), respectively. In any case, the LUMO levels are located 400 mV above the conduction band of TiO2, which favourably supports electron injection from the dye excited state upon light absorption. Similarly, the positive shift of dye HOMO levels compared to the I−/I3− redox couple ensures efficient regeneration of the ground state for both dyes.
2.3. Theoretical studies
To gain insight into the electron density distribution in the ground and excited states of the YK dyes, we performed molecular modelling experiments. To reduce the complexity in modelling, the octyl chains present in the π-bridge were replaced with methyl groups, given that this operation would not cause considerable changes to the optoelectronic properties of the light-harvesting core. The geometries were first optimized in the gas phase using the DFT method at the B3LYP/6-311G(d,p) level in the Gaussian 16 program package.55,56 The optimized structure of the dyes and the electron density delocalization in their HOMO and LUMO energy levels are depicted in Fig. 3. Compared to YK 8, YK 9 exhibits a planar π-backbone, leading to more dye loading and aggregation. In both cases, the electron density of the HOMO is distributed all over the dyes and extended towards the thiophene π-spacer, including the anchoring group, whereas for the LUMO, the electron density is primarily localized on the cyanoacrylic acid and adjacent thiophene units.
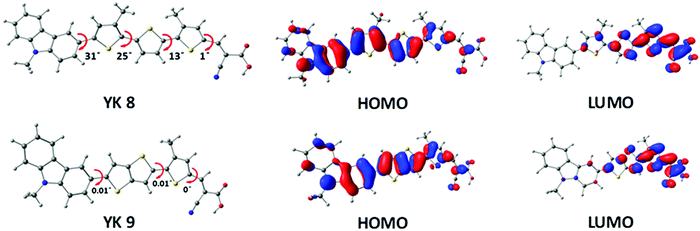 |
| Fig. 3 Optimized geometry, ground state and excited state electron density distribution for the YK 8 and YK 9 dyes. | |
The MK 2 sensitizer also shows a similar HOMO electron density distribution, where the HOMO is delocalized over the whole MK 2 molecule, including the anchoring group, as reported previously.57,58 Despite having similar HOMO electron density distributions, the YK 8 and YK 9 sensitizers showed a difference in recombination given that it depends on factors such as number of alkyl chains, orientation of the dye molecules, Fermi–Dirac distribution of electrons in the TiO2 conduction band and aggregation. Recently H. Zhang et al. has also reported that a small proportion of the HOMO distributed on the anchoring groups of dyes could also reduce charge recombination.59 The HOMO, LUMO and band gap values obtained from the theoretical studies are presented in Table 1, which agree well with the experimental results. We also calculated the light-harvesting efficiency (LHE) of the dyes via TDDFT calculations in toluene (PCM) based on the 6-311G(d,p) basis sets with M062X functional using the equation LHE = 1–10−f, where f is the oscillator strength obtained from TDDFT calculations and the estimated LHE of the two dyes are 0.97 and 0.98 for YK 8 and YK 9, respectively. The energy transfer percentage from the HOMO to LUMO is 61% and 64% for the YK 8 and YK 9 sensitizers, respectively.
Table 1 Electrochemical parameters of YK 8 and YK 9 dyes obtained from the theoretical method
Dye |
HOMO (eV) |
LUMO (eV) |
Bandgap (eV) |
YK 8
|
−5.38 |
−2.99 |
2.38 |
YK 9
|
−5.42 |
−3.02 |
2.40 |
2.4. Photovoltaic performance
Dye-sensitized solar cells were fabricated using the conventional architecture, as reported previously by our group, which is provided in Fig. S2a (ESI†). The detailed fabrication procedures are given in the Experimental section, and the fabricated devices are shown in Fig. S2b (ESI†). Details on the certified equipment used for characterization are also provided in the ESI.† The current density–voltage (J–V) characteristics were measured under one sun illumination (100 mW cm−2, AM1.5G). To achieve better power conversion efficiency, we carried out detailed optimization of the semiconductor architecture and co-adsorbent concentration for both the YK 8 and YK 9 dyes. The J–V plots are displayed in Fig. S3 and S4 (ESI†), and the tabulated data is summarized in Table S1 and S2 (ESI†). Initial optimization of the TiO2 layer thickness was carried out without a co-adsorbent (CDCA), where the YK 8-based DSCs exhibited a power conversion efficiency of 6.62% ± 0.04% using 6 μm TiO2 and 6.30% ± 0.02% using 10 μm TiO2. YK 9 exhibited a power conversion efficiency of 6.55% ± 0.21% with a 6 μm TiO2 layer and 5.51% ± 0.02% using a 10 μm TiO2 layer. With the added advantage of having a higher molar extinction coefficient in the visible region, both organic metal-free sensitizers exhibited the best performances with a 6 μm TiO2 layer. Thinner electrodes render better transparencies and also contribute to less dye usage, thereby reducing the production cost.
One of the significant problems associated with organic metal-free sensitizers is the aggregation of the dyes on the semiconductor surface. Thus, to control the dye aggregation, leading to a higher performance, we carried out a co-adsorbent concentration-dependent study by varying the concentration of chenodeoxycholic acid. YK 8 delivered the best power conversion efficiency of 7.49% ± 0.09% for the devices fabricated with 1 mM CDCA, whereas YK 9 exhibited the best PCE of 6.60% ± 0.13% using 5 mM CDCA (Table 2). The results obtained for the detailed optimization involving various CDCA concentrations (0 mM, 1 mM, 2.5 mM, 5 mM and 10 mM) are summarized in Fig. S4 and Table S2 (ESI†). These observations indicate that YK 9 is more prone to aggregation on the surface of TiO2. The YK 9 dye has only one alkyl chain grafted to the thiophene π-spacer compared to YK 8, which has two-alkyl chains attached to the π-spacer. The presence of alkyl chains prevents the aggregation of the YK 8 dye on the semiconductor surface at the expense of the number of dye molecules. The adsorbed amount of dye molecules for YK 8 and YK 9 is 9.76 × 10−8 mol cm−2 and 18.08 × 10−8 mol cm−2, respectively. Additionally, the incorporation of a more planar thienothiophene π-spacer in YK 9 dye contributed to its planar geometry, as evident from its dihedral angle (Fig. 3). This better planarity led to stronger π–π interactions, resulting in more aggregation and leading to higher charge recombination and decreased performance for YK 9 compared to YK 8. It is evident that the much higher amount of surface coverage of YK 9 is just more aggregates and detrimental to the solar cell performance. Thus, the surface coverage of YK 8 is enough for efficient light-harvesting, as can be inferred from the absorption spectrum of the dyes on TiO2 and the IPCE spectra. The UV-Vis spectra of the dye-adsorbed TiO2 film (Fig. S5, ESI†) show that both the YK 8 and YK 9 sensitized photoanodes show strong absorption bands in the visible region (300–680 nm), and YK 8 shows a red-shifted absorption in the longer wavelength region compared with YK 9, similar to the IPCE plot (Fig. 4b).
Table 2 Summary of photovoltaic performance of DSCs based on the YK 8 and YK 9 dyes
Device |
V
oc (mV) |
J
sc (mA cm−2) |
Integrated Jsc (mA cm−2) |
FF (%) |
Efficiency (%) |
YK 8
|
688 ± 4 |
16.20 ± 0.13 |
15.84 |
67.10 ± 0.18 |
7.49 ± 0.09 |
YK 9
|
697 ± 2 |
13.82 ± 0.08 |
13.04 |
68.29 ± 0.27 |
6.60 ± 0.13 |
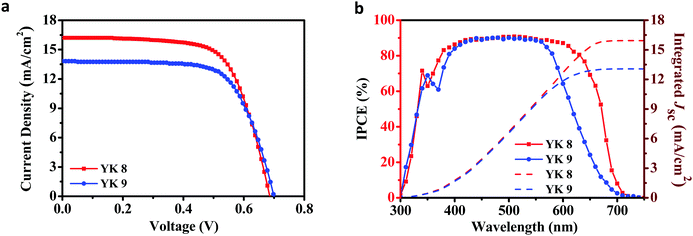 |
| Fig. 4 (a) Current density–voltage (J–V) characteristics and (b) incident photon-to-current conversion efficiency (IPCE) and integrated Jsc calculated from IPCE spectra for DSCs fabricated using the YK 8 and YK 9 dyes. | |
For both the YK 8 and YK 9 dyes, their photovoltaic performance improved with time (ageing). Initially, YK 8 and YK 9 exhibited a power conversion efficiency of 7.49% ± 0.09% and 6.90% ± 0.10%, which improved to 9.39% ± 0.18% and 7.48% ± 0.03% with the ageing of the devices under standard indoor conditions for close to four months (2700 h), respectively (Fig. S6 and Table S4, ESI†). YK 8 showed a 25.37% increase in PCE with ageing, whereas YK 9 exhibited around an 8.40% increase in PCE. With the best-optimized device architecture and co-adsorbent concentration, the power conversion efficiency of 9.39% ± 0.18% with Jsc of 17.24 ± 0.10 mA cm−2, Voc of 790 ± 6 mV and FF of 68.93 ± 0.29% were achieved for the YK 8 dye under one sun illumination (100 mW cm−2, AM1.5G) after 2700 h. Similarly, YK 9 exhibited a PCE of 7.48% ± 0.03% with a Jsc of 14.26 ± 0.02 mA cm−2, Voc of 750 ± 1 mV and FF of 69.91% ± 0.35% after 2700 h. We used the commercially available standard carbazole-based metal free organic dye MK 2 to benchmark and compare the photovoltaic performance of our newly synthesized sensitizers. Under similar experimental conditions, MK 2 exhibited a PCE of 7.74% ± 0.04% with a Jsc of 15.11 ± 0.07 mA cm−2, Voc of 751 ± 1 mV and FF of 68.26% ± 0.15% (Fig. S7 and Table S3, ESI†). Fig. 5(d) shows the variations in efficiency as a function of time, where both the YK 8 and YK 9 dyes outperformed the commercial MK 2 dye and exhibited their best performance after remaining in the dark for 2700 h (∼4 months). The enhancement in PV performance with ageing can be attributed to the better pore filling and electrolyte infiltration in the semiconductor matrix, leading to better dye regeneration and charge collection, as reported previously.60 These factors contribute to the variation in PCE with ageing for both dyes. The use of a thin-film photoanode contributed to a decrease in the density of bandgap trap states (DOS) and the surface area of the semiconductor, which may have contributed to a better electron lifetime (τn), electron diffusion length (Ln) and charge collection efficiency (ηcc).61,62 This is reflected in the IPCE spectra of both dyes after ageing with over 90% absorption in the visible region (Fig. S6b, ESI†). To avoid the overestimation of Jsc due to stray light, during all the photovoltaic characterizations, we used a black mask with an aperture of 0.1256 cm2 (radius of 2 mm) over the device with a total active area of 0.31 cm2. All the photovoltaic results reported are from the data obtained from 8 devices, and the error was estimated using the standard deviation method. We integrated Jsc from the IPCE measurement, which falls well within the permissible error limits with the Jsc obtained from the IV measurements [mismatch is only 2.22% for YK 8 and 5.64% for YK 9 (for the fresh devices) and 1.3% for YK 8 and 3.8% for YK 9 (for the aged devices, 2700 h)], measured under standard experimental conditions. It is clear from Fig. 4b that there is a red-shift in the IPCE spectra for the YK 8 dye between 550 nm and 700 nm compared to YK 9, which contributed to an extra 3 mA cm−2 current density for the YK 8 dye. The IPCE values in the blue region and the onset of IPCE for both YK 8 and YK 9 are the same.
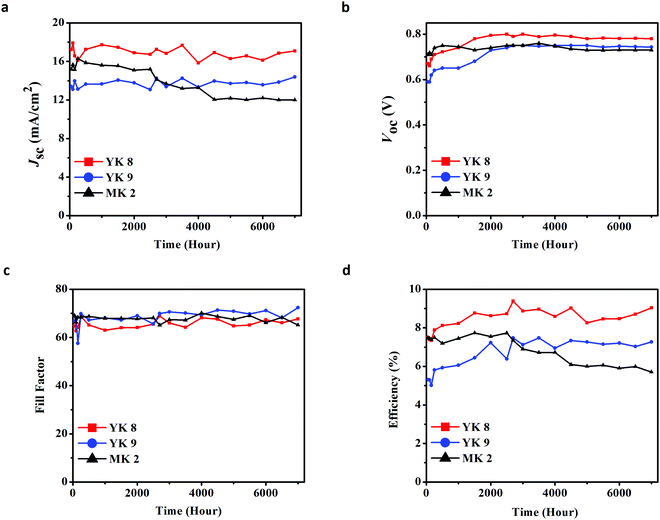 |
| Fig. 5 (a) Jscversus time, (b) Vocversus time, (c) fill factor versus time, and (d) efficiency versus time up to 7000 h for DSCs using YK 8, YK 9 and MK 2 sensitizers. | |
Ensuring device stability is the prime pre-requisite for potential sensitizers to be developed from small-area cells to large-area modules. Fig. 5 reveals 7000 h of stability measurements carried out by keeping the devices under standard indoor conditions and carrying out the measurements at 500 h intervals for the YK 8, YK 9 and commercial MK 2 dyes. YK 8 showed <1% decrease in Voc, ∼5% decrease in Jsc, ∼6% decrease in FF and ∼12% decrease in overall efficiency after 7000 h. Likewise, YK 9 showed a nearly constant Voc, ∼3% decrease in Jsc, <1% decrease in FF and ∼2% drop in efficiency after 7000 h. In contrast, the commercial MK 2 dye resulted in an ∼3% decrease in Voc, ∼19% decrease in Jsc, and ∼2% decrease in FF, which contributed to a >20% decrease in efficiency after 7000 h. These results are quite exciting as both newly synthesized dyes maintained their photovoltaic performance over an extended period compared to the commercial MK 2 dye. Initially, the YK 9 dye showed an inferior performance to MK 2, but with time, after 3000 h, YK 9 outperformed MK 2 in its PV performance. The higher open-circuit potential and short circuit current density endowed the YK 8 sensitizer a higher PV performance than YK 9 under 1 sun (100 mW cm−2) conditions. The presence of more alkyl chains in the π-spacer and by virtue of its inherent molecular geometry, YK 8 was efficient in controlling its aggregation, contributing to its better lifetime and open circuit potential compared to that of YK 9. We carried out a range of electrical perturbation measurements to probe the charge transfer dynamics at various interfaces in the fabricated devices employing both the YK 8 and YK 9 dyes. This helped to gain a deeper understanding of the variations in current and voltage exhibited by both sensitizers and is discussed in detail in Section 2.6.
2.5. Indoor-light measurements
Unlike outdoor light conditions, indoor illumination is diverse in terms of magnitude and the spectrum of sources. Usually, the indoor/ambient illumination ranges between zero and 2000 lux, which is nearly equal to 0.02 sun. We carried out the indoor J–V measurements using Osram 14 W T2 cool daylight fluorescent tube illumination for the YK 8 and YK 9 dyes. The J–V plots under indoor fluorescent light illumination at various intensities (700 lux, 1000 lux, 1500 lux and 2000 lux) are displayed in Fig. 6b and Fig. S8a (ESI†) and the obtained PV parameters are summarized in Table 3. Below 700 lux, we observed larger fluctuations in output power using CFL lamps. The power spectrum of the indoor light source used in the present study overlapped with the EQE spectra of the YK 8 and YK 9 dyes and the AM1.5G spectra are shown in Fig. 6a. Under 1000 lux standard CFL illumination, YK 8 realized a PCE of 28.7% ± 1.14% (Pmax = 68.88 μW cm−2, Voc = 669 ± 7 mV, Jsc = 141 ± 3 μA cm−2, and FF = 72.10% ± 1.28%) and YK 9 attained a lower PCE of 14.21% ± 0.56% (Pmax = 34.10 μW cm−2, Voc = 589 ± 7 mV, Jsc = 103 ± 2 μA cm−2, and FF = 58.39% ± 1.55%) using the conventional iodide/triiodide electrolyte devoid of any co-sensitizers, which is better than the literature reports on related systems under similar testing conditions and device architecture (Table S6, ESI†). With a higher incident light intensity of 1500 lux, the YK 8 sensitizer exhibited the maximum power conversion efficiency of 30.24% ± 1.23% (Pmax = 108.85 μW cm−2, Voc = 679 ± 1 mV, Jsc = 200 ± 2 μA cm−2, and FF = 80.03% ± 2.30%). The DSCs employing YK 9 showed a maximum power conversion efficiency of 20.11% ± 0.96% (Pmax = 96.53 μW cm−2, Voc = 619 ± 1 mV, Jsc = 208 ± 13 μA cm−2, and FF = 76.08% ± 2.53%) at 2000 lux.
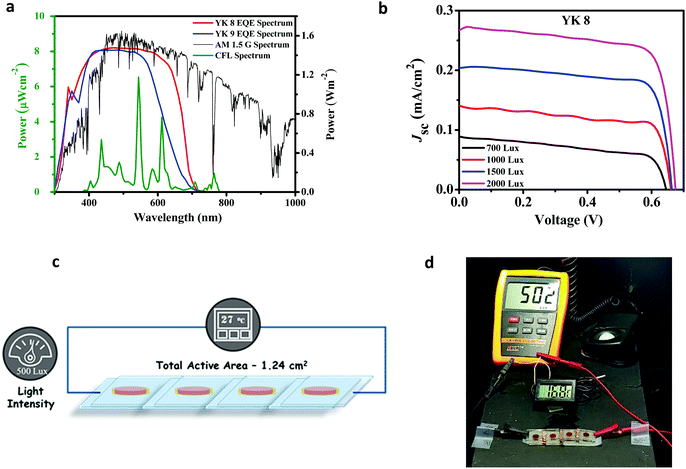 |
| Fig. 6 (a) Power spectra of Osram 14 W T2 cool daylight fluorescent illumination overlapped with the EQE spectra of the YK 8 and YK 9 dyes and AM1.5G spectra. (b) Photovoltaic characteristics of DSCs under indoor-light source (Osram 14 W T2 cool daylight fluorescent tube illumination) for the YK 8 sensitizer at 700 lux, 1000 lux, 1500 lux and 2000 lux. (c) Illustrative design and (d) image of self-powered temperature sensor coupled with four serially connected DSCs fabricated with the YK 8 sensitizer at 500 lux (Osram 14 W T2 cool daylight fluorescent tube illumination). | |
Table 3 Tabulated photovoltaic data of DSCs with the YK 8 and YK 9 dyes under Osram 14 W T2 cool daylight fluorescent tube illumination at 700 lux, 1000 lux, 1500 lux and 2000 lux
Intensity (lux) |
Input power (μW cm−2) |
Output power (μW cm−2) |
V
oc (mV) |
J
sc (μA cm−2) |
FF (%) |
Efficiency (%) |
YK 8
|
700 |
160 |
35.43 |
649 ± 1 |
90 ± 2 |
60.70 ± 0.94 |
22.15 ± 0.42 |
1000 |
240 |
68.88 |
669 ± 7 |
141 ± 3 |
72.10 ± 1.28 |
28.70 ± 1.14 |
1500 |
360 |
108.85 |
679 ± 1 |
200 ± 2 |
80.03 ± 2.30 |
30.24 ± 1.23 |
2000 |
480 |
141.27 |
689 ± 1 |
270 ± 5 |
76.95 ± 1.46 |
29.43 ± 0.46 |
|
YK 9
|
700 |
160 |
19.98 |
568 ± 7 |
69 ± 1 |
52.81 ± 1.37 |
12.49 ± 0.87 |
1000 |
240 |
34.10 |
589 ± 7 |
103 ± 2 |
58.39 ± 1.55 |
14.21 ± 0.56 |
1500 |
360 |
64.23 |
608 ± 6 |
154 ± 4 |
69.51 ± 2.26 |
17.84 ± 1.17 |
2000 |
480 |
96.53 |
619 ± 1 |
208 ± 13 |
76.08 ± 2.53 |
20.11 ± 0.96 |
With an increase in light intensity (700 lux to 2000 lux), both the Jsc and Voc increased for the YK 8 and YK 9 sensitizers. YK 8 outperformed YK 9 under indoor light conditions, which is mainly attributed to the higher voltage and current generated for the YK 8 sensitizer under these conditions. More importantly, the red-shifted absorption in the range of 550–700 nm for the YK 8 sensitizer, as evident from the EQE spectra, matches with the spectra of the cool daylight fluorescent tube illumination used in the present study (Fig. 6a), leading to better light harvesting. The use of thinner electrodes also reduces deep surface and trap states, contributing to a better PCE.63,64 Additionally, the recombination process from existing sub-bandgap states also gets saturated at lower intensities, which can contribute to an improved lifetime and better charge collection efficiency, translating to a better current and voltage for the YK 8 devices under indoor light. Thus, we have shown that in addition to the nature of the donor unit, the π-spacers also contribute to determining the performance of the organic dyes under indoor illumination. By preventing recombination, we can realize a higher shunt resistance, which improves the PV performance under low light illuminations. In the present contribution, YK 8 offers more shunt resistance at 1500 lux, which may have also contributed to its better PCE at this particular light intensity. The shunt resistance follows the order of 700 lux (192.7 kΩ) < 1000 lux (254.6 kΩ) < 2000 lux (352.1 kΩ) < 1500 lux (520.8 kΩ). Under low illumination intensities, suitable control of recombination either by changing the dye or modifying the electrodes/electrolytes can lead to improved power conversion efficiencies. For comparison, the standard metal-free MK 2 organic dye was also tested under similar conditions, which displayed an efficiency of 24.05% ± 0.47% at 1000 lux with Jsc = 132 ± 4 μA cm−2, Voc = 649 ± 7 mV and FF = 66.26% ± 1.20%, leading to a net power output of 57.72 μW cm−2, and a PCE of 25.74 ± 0.46% was obtained at 1500 lux with Jsc = 195 ± 11 μA cm−2, Voc = 669 ± 6 mV and FF = 71.99 ± 1.69%, leading to a net power output of 92.65 μW cm−2 (Fig. S8b and c and Table S5, ESI†). Thus, YK 8 displayed a better device performance than both the YK 9 and commercial MK 2 sensitizers under Osram 14 W T2 cool daylight fluorescent tube illumination. Under 1500 lux, YK 8 yielded a 69.51% increase in PV performance compared to YK 9 and 17.48% increment in the case of the MK 2 dye.
To the best of our knowledge, the obtained efficiency for the YK 8 sensitizer is among the best reported performances to date for a DSC device using a single organic metal-free dye without co-sensitization, in combination with iodide/triiodide electrolyte under fluorescent tube illumination (<2000 lux) (Table S6, ESI†). A detailed comparison of the indoor photovoltaic performance of the YK 8 and YK 9 sensitizers with other dyes reported thus far, which were measured under similar indoor illumination conditions using iodide/triiodide electrolyte, is provided in Table S6 (ESI†) and a list of the highest reported indoor performances using DSCs is provided in Table S7 (ESI†). In addition to the higher indoor performance delivered by the YK 8 sensitizer compared to the standard MK2 dye, the former was cheaper to synthesize. A detailed step-by-step cost calculation for the synthesis of the YK 8 dye is provided in the ESI† (Table S8), which shows that one gram of YK 8 can be synthesized at a lower price ($585 per gram) than many of the commercially available efficient organic sensitizers.
To demonstrate the real potential of these indoor/artificial light harvesters, we custom designed a fully functional self-powered temperature sensor with an LCD that draws power from the indoor solar cells made using the YK 8 sensitizer. Four of these solar cells, each having an active area of 0.31 cm2 were serially interconnected (net active area of 1.24 cm2) to power the temperature sensor (Fig. S9, ESI†). An illumination of 500 lux provided by the fluorescent light source was enough to generate sufficient power to get the temperature sensor completely autonomous without any battery. An illustrative design of the developed setup is given in Fig. 6c and d, and a video is also provided.
2.6. Charge transfer dynamics
The open circuit voltage (Voc) is an intrinsic property of a device, which is influenced by its structure, energetics and materials used for its fabrication. In DSCs, the energy difference between the Fermi level of TiO2 and the redox potential of the electrolyte determines the Voc.65,66 In the present work, we employed iodide/triiodide redox electrolyte (redox potential 0.40 V vs. NHE) with both the YK 8 and YK 9 sensitizers. It is well-known that the electron concentration in a semiconductor determines its Fermi level.67–69 With ample electrons, the Fermi level can lie very close to the conduction band (CB) of TiO2. Again, the position of the TiO2 CB and recombination are two key parameters that maintain TiO2 Fermi level. Any minor change in electrolyte composition, sensitizer design and even TiO2 preparation method influences the TiO2 CB and rate of recombination.70–72
The TiO2/dye/electrolyte interface is the most relevant interface in DSCs given that the injected electrons are prone to recombine with the holes present in the electrolyte and oxidised dye. It is imperative to investigate the TiO2/dye/electrolyte interface to understand the origin of the variation in the open circuit potential. We probed the TiO2/dye/electrolyte interface using electrochemical impedance spectroscopy (EIS) in the dark with a frequency ranging from 100 mHz to 100 kHz. The corresponding Nyquist plots are given in Fig. S10 (ESI†). The Nyquist plots were fitted using the transmission line model and analysed using the theory proposed by Bisquert et al.73,74 Fig. S11 (ESI†) shows the relationship between the chemical capacitance (Cμ) and corrected voltage drop due to the series resistance (VF). The chemical capacitance (Cμ) is commonly used to locate the position of the CB in TiO2.75–77 Both the YK 8 and YK 9 sensitizers showed almost similar capacitance values over the lower voltage range, which indicates that the conduction band of TiO2 remained the same for both dyes and the variation in voltage is independent of the conduction band edge. The capacitance data for YK 9 falls off at higher voltages. This can be due to some type of leakage of electrons for YK 9 as a result of more recombination in YK 9 compared to YK 8.
To probe the origin of the improvement in voltage for the YK 8 sensitizer, we investigated the recombination resistance (Rct) and lifetime (τn) in detail by EIS and IMVS measurements. YK 8 showed a higher recombination resistance compared to YK 9 (Fig. 7a). The lifetime results (Fig. 7b) exhibited the same trend following the recombination resistance. The YK 8 dye having two dodecyl chains attached on the terthiophene π-spacer efficiently prevents the approach of oxidised species present in the electrolyte from coming close to the semiconductor in comparison to the YK 9 dye, having a more planar thienothiophene spacer with only one dodecyl substituent. Thus, YK 8 with more alkyl chains and a non-planar geometry forms a stronger shield against recombination, yielding a better electron lifetime. In addition, the better planarity of the YK 9 dye will impart strong π–π interactions, resulting in more aggregation, as evident from the PV data obtained with variable CDCA concentrations (Fig. S4, ESI†), which leads to more recombination. The dark current measurement data given in Fig. S12 (ESI†) also supports this argument and is consistent with the lifetime plots, where the YK 8 sensitizer showed a lower dark current compared to the YK 9 dye. The lifetime was also measured using intensity modulated photovoltage spectroscopy (IMVS). Fig. S13 (ESI†) shows the lifetime from the IMVS measurements for the metal-free sensitizers, which followed the same trend as the EIS data. Fig. 7c shows the diffusion length (Ln) as a function of corrected voltage (VF). The YK 8 devices exhibited a better Ln compared to the YK 9 devices. The electron diffusion length is defined as the average distance an electron is transported before it gets recombined. Thus, the better diffusion length translated into an improved charge collection efficiency (ηcc) for the YK 8 sensitizer compared to YK 9 (Fig. 7d).
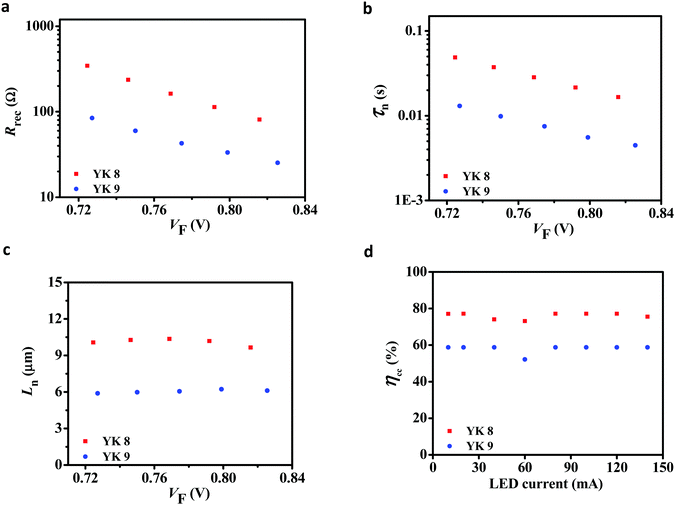 |
| Fig. 7 (a) Recombination resistance (Rrec), (b) lifetime (τn) and (c) diffusion length (Ln) as function of corrected voltage (VF) and (d) charge collection efficiency (ηcc) as a function of LED current for the YK 8 and YK 9 sensitizers. | |
Photo-induced absorption spectroscopy (PIA) is used to qualitatively study the electron injection from the oxidised dye to the CB of TiO2 and regeneration of the oxidised dye by the reduced species present in the electrolyte. To evaluate the injection, the PIA spectra of the YK 8- and YK 9-soaked TiO2 electrodes were analysed. Fig. 8a shows the PIA spectra of the dye-sensitized TiO2. Both YK 8 and YK 9 show ground state bleach superimposed with a Stark shift at 530 nm. The interaction of the oxidised dye and injected electrons in TiO2 induces a local electric field across the dye molecule, contributing to the Stark shift.78–82 The absorption peak due to the oxidised dye molecule was observed at 780 nm for YK 8 and 700 nm for YK 9. The absorption peak is observed when the excited electrons in the LUMO of the dye are injected to the CB of TiO2. The visible absorption peak of the oxidised dye molecules for both sensitizers in the range of 650 nm to 850 nm indicates the efficient injection of electrons for both YK 8 and YK 9 sensitizers.
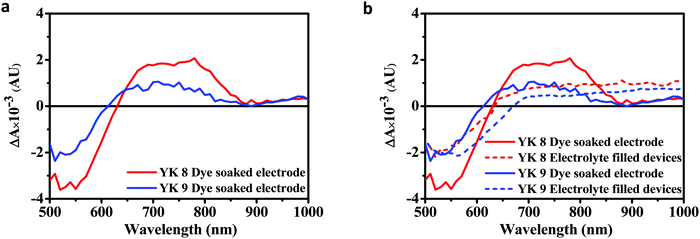 |
| Fig. 8 PIA spectra of the YK 8 and YK 9 dyes (a) adsorbed on 6 μm TiO2 film and (b) dye soaked 6 μm TiO2 film electrodes and iodide/triiodide electrolyte-filled devices. | |
We carried out PIA measurements in electrolyte-filled devices for the regeneration study and compared them with the dye-soaked electrodes. Fig. 8b shows the PIA spectra of the dye alone and electrolyte-filled devices for YK 8 and YK 9. In the electrolyte filled devices, the ground state bleach superimposed with a Stark shift was observed at 530 nm and 560 nm for YK 8 and YK 9, respectively. As observed in Fig. 8b, the peak due to the absorption of the oxidised dye disappeared for both YK 8 and YK 9 with the addition of the electrolyte to the corresponding devices, which reveals that the oxidised ground state of the dye is efficiently regenerated by the reduced species present in the electrolyte for both the YK 8 and YK 9 sensitizers. The difference in intensities in PIA between the YK 8 and YK 9 dyes indicates that YK 8 has a relatively higher injection and regeneration yield than YK 9, which also contributed to the better photovoltaic performance of YK 8.
Briefly, its low aggregation on the surface of TiO2 and the added advantage of having more alkyl chains to prevent recombination assisted the YK 8 dye to achieve an improved lifetime, leading to a better open-circuit voltage. In addition, the better diffusion length contributed to the superior charge collection efficiency of the YK 8 sensitizer. Besides, the added advantage of a red-shifted absorption and relatively higher injection and regeneration yield helped YK 8 in realizing a higher photovoltage and photocurrent in both full sun and indoor light conditions. Further, in addition to the higher performance under indoor illumination, the potential for scaling up the synthesis of the YK 8 sensitizer at a lower cost compared to commercial standards renders immense possibilities to use this dye in modules/panels in the future.
3. Experimental
3.1. Materials and methods
The reagents and materials used for synthesis were of analytical grade and purchased from Ms Sigma-Aldrich, Ms Merck, and Ms Spectrochem Chemical, and used as received without further purification. All reactions were performed under an argon atmosphere. Before the Suzuki and Stille coupling reactions, any dissolved gas bubbles in the reaction mixture were removed by the freeze pump thaw method using liquid nitrogen and vacuum. The progress of the reaction was first assessed using thin layer chromatography on precoated Merck TLC silica gel 60 F254 silica plates. Purification of the product was done using column chromatography with 100–200 mesh Merck silica gel. 1H and 13C NMR spectra were recorded with a Bruker 500 MHz spectrometer using tetramethylsilane as the internal standard. Chemical shifts (δ) are given in ppm, and deuterated solvents were used for the measurements. Mass spectra of all the intermediates and final products were recorded on a Thermo Scientific Exactive spectrometer in electrospray ionization mass spectrometric (ESI) mode. IR spectra (ATR-FTIR or FTIR-KBr) were recorded using a Spectrum Two FTIR spectrometer by PerkinElmer. The structural characterization of the YK 8 and YK 9 dyes (1H NMR, 13C NMR, HRMS and ATR-FTIR) is presented in Fig. S15(i–xxxiv) (ESI†). Electronic absorption spectra were recorded on a UV-2600 UV-Vis spectrophotometer (Ms Shimadzu) using a 1 cm path length quartz cuvette. Cyclic voltammetry experiments were performed on a BAS 50 W voltammetric analyzer using 0.1 M tetrabutylammonium hexafluorophosphate in dichloromethane as the supporting electrolyte, glassy carbon working electrode, Ag/AgCl reference electrode, and platinum wire counter electrode. Density functional theory (DFT) calculations were performed at the B3LYP/6-311G/(d,p) level using the Gaussian 16 program.
3.2. Synthesis
3.2.1. Synthesis of compound 1.
A solution of 2,5-bis(tributylstannyl)thiophene (500 mg, 0.76 mmol) and 2-bromo-3-octylthiophene (436 mg, 1.58 mmol) in 20 mL dry toluene was subjected to the freeze pump thaw method to remove dissolved oxygen and moisture. Then, Pd2(dba)3 (20 mg, 0.02 mmol) and P(o-tolyl)3 (30 mg, 0.1 mmol) were added under an argon atmosphere and stirred under reflux overnight. The reaction mixture was then cooled to room temperature, diluted with dichloromethane, and subsequently washed with water and brine, and then dried over anhydrous Na2SO4. The crude mixture was purified by column chromatography over silica gel (100–200 mesh) using hexane as the eluent to afford the corresponding product 1 as a yellow oil. Yield (303 mg, 85%). Mass (ESI MS): 472.2292, found: 473.2287 (MH+); 1H NMR (CDCl3, 500 MHz) δ/ppm = 7.17 (d, J = 5 Hz, 2H), 7.05 (s, 2H), 6.93 (d, J = 5 Hz, 2H), 2.78 (t, J = 7.5 Hz, 4H), 1.67–1.61 (m, 4H), 1.37–1.26 (m, 20H), 0.87 (t, J = 7 Hz, 6H); 13C NMR (CDCl3, 126 MHz) δ/ppm = 139.72, 136.07, 130.42, 130.08, 126.06, 123.74, 31.91, 30.78, 29.60, 29.48, 29.31, 22.70, 14.14; IR (KBr) ν in cm−1 = 2954, 2922, 2852, 1462, 1417, 1375, 1340, 1290, 1070, 1001, 960, 873, 831, 721, 690, 665, 594.
3.2.2. Synthesis of compound 2.
To a solution of compound 1 (1 g, 2.12 mmol) and N,N-dimethylformamide (1.8 mL, 23.24 mmol) in 1,2-dichloroethane (20 mL), POCl3 (0.3 mL, 3.2 mmol) was slowly added at 0 °C and stirred for 1 h under an argon atmosphere. Then, the reaction mixture was heated to 60 °C and stirred overnight. After cooling to room temperature, it was neutralized with NaHCO3 (aq.), extracted using dichloromethane and dried over anhydrous Na2SO4. The crude product was purified by column chromatography over silica gel (100–200 mesh) using hexane as the eluent to afford product 2 as an orange oil. Yield 800 mg (76%). Mass (ESI MS): 500.2241, found: 501.2249 (MH+); 1H NMR (CDCl3, 500 MHz) δ/ppm = 9.83 (s, 1H), 7.60 (s, 1H), 7.25 (d, J = 5 Hz, 1H), 7.22 (d, J = 5 Hz, 1H), 7.10 (d, J = 5 Hz, 1H), 6.95 (d, J = 5 Hz, 1H), 2.84–2.77 (m, 4H), 1.72–1.62 (m, 4H), 1.42–1.35 (m, 4H), 1.32–1.26 (m, 16H), 0.89–0.86 (m, 6H); 13C NMR (CDCl3, 126 MHz) δ/ppm = 182.59, 141.18, 140.40, 140.31, 140.19, 139.10, 138.51, 134.47, 130.26, 129.77, 127.80, 126.34, 124.41, 31.90, 31.87, 30.72, 30.35, 29.59, 29.51, 29.46, 29.42, 29.38, 29.29, 29.26, 22.69, 14.12; IR (KBr) ν in cm−1 = 2954, 2924, 2850, 1670, 1456, 1433, 1393, 1242, 1155, 858, 829, 798, 721, 675.
3.2.3. Synthesis of compound 3.
To a stirred solution of compound 2 (500 mg, 1 mmol) in N,N-dimethylformamide (10 mL), and N-bromosuccinimide (266 mg, 1.5 mmol) were added in the dark and stirred at room temperature for 24 h. After completion of the reaction, the organic layer was extracted with dichloromethane, washed with NaHCO3 (aq.), water and brine, and dried over anhydrous Na2SO4. The crude product was purified by column chromatography over silica gel (100–200 mesh) using hexane and dichloromethane (1
:
1, v/v) as the eluent to afford the corresponding product 3 as a yellow oil. Yield 400 mg (69%). Mass (ESI MS): 578.1346, found: 579.1421 (MH+), 581.1398 ((M + 2) H+); 1H NMR (CDCl3, 500 MHz) δ/ppm = 9.80 (s, 1H), 7.57 (s, 1H), 7.20 (d, J = 5 Hz, 1H), 7.00 (d, J = 5 Hz, 1H), 6.89 (s, 1H), 2.80–2.67 (m, 4H), 1.70–1.57 (m, 4H), 1.39–1.34 (m, 4H), 1.31–1.25 (m, 16H), 0.87–0.85 (m, 6H); 13C NMR (CDCl3, 126 MHz) δ/ppm = 182.59, 144.58, 141.46, 141.22, 138.88, 138.69, 136.31, 132.85, 131.76, 130.21, 127.35, 113.79, 112.95, 31.87, 30.4, 30.34, 29.50, 29.46, 29.42, 29.36, 29.32, 29.29, 29.25, 29.22, 29.20, 29.17, 26.93, 22.67, 14.12; IR (KBr) ν in cm−1 = 3079, 2960, 2920, 2850, 1662, 1491, 1467, 1409, 1390, 1332, 1261, 1245, 1156, 1097, 1023, 866, 844, 803, 690.
3.2.4. Synthesis of compound 4.
To a solution of compound 3 (543 mg, 0.94 mmol), K2CO3 (276 mg in 1 mL water, 2 M), 9-methyl-9H-carbazole-3-boronic acid pinacol ester (288 mg, 0.94 mmol) was added. 20 mL of toluene was added to it and the entire system was subjected to freeze pump thawing to remove the dissolved oxygen. Then, Pd(PPh3)4 (30 mg, 0.03 mmol) was added under an argon atmosphere and refluxed for 18 h. The reaction mixture was extracted with dichloromethane and purified by column chromatography over silica gel (100–200 mesh) using dichloromethane and hexane (1
:
4, v/v) as the eluent to yield compound 4 as a red solid. Yield 565 mg (88%). Mass (ESI MS): 679.2976, found: 679.2970 (MH+); 1H NMR (500 MHz, CDCl3) δ/ppm = 9.83 (s, 1H), 8.32 (s, 1H), 8.14 (d, J = 8 Hz, 1H), 7.74 (dd, J = 2, 8 Hz, 1H), 7.61 (s, 1H), 7.51 (m, 1H), 7.42 (t, J = 7 Hz, 2H), 7.27 (m, 2H), 7.23 (s, 1H), 7.16 (d, J = 4 Hz, 1H), 3.88 (s, 3H), 2.87–2.82 (m, 4H), 1.78–1.68 (m, 4H), 1.48–1.41 (m, 4H), 1.38–1.26 (m, 16H), 0.89–0.87 (m, 6H); 13C NMR (CDCl3, 126 MHz) δ/ppm = 182.48, 144.25, 143.19, 141.53, 141.41, 141.36, 140.75, 140.21, 140.12, 139.06, 138.96, 128.05, 127.89, 126.92, 125.73, 125.22, 123.97, 123.30, 122.73, 120.50, 119.27, 117.53, 108.81, 108.71, 31.94, 31.89, 30.68, 30.36, 29.84, 29.72, 29.54, 29.52, 29.44, 29.33, 29.28, 29.26, 22.71, 22.68, 14.12; IR (KBr) ν in cm−1 = 3057, 2954, 2924, 2848, 1664, 1539, 1483, 1427, 1393, 1334, 1249, 1153, 1062, 871, 806, 781, 742, 723, 667.
3.2.5. Synthesis of YK 8.
Compound 4 (200 mg, 0.3 mmol) and cyanoacetic acid (130 mg, 1.5 mmol) were dissolved in 20 mL dry chloroform. Then two drops of piperidine were added to this mixture and stirred at 45 °C for 40 h. The reaction mixture was extracted with chloroform, washed with water, dried over anhydrous Na2SO4 and purified by column chromatography over silica gel (100–200 mesh) using chloroform and methanol (9
:
1, v/v) as the eluent to give compound YK 8. Yield 171 mg (78%). Mass (ESI MS): 746.3034, found: 747.3112 (MH+); 1H NMR (500 MHz, DMSO-d6) δ/ppm = 8.42 (s, 1H), 8.30 (s, 1H), 8.20 (d, J = 8 Hz, 1H), 7.78 (s, 1H), 7.71 (d, J = 8.5 Hz, 1H), 7.57 (t, J = 8 Hz, 2H), 7.48 (t, J = 7.5 Hz, 1H), 7.42 (s, 1H), 7.34 (s, 1H), 7.24–7.21 (m, 2H), 3.85 (s, 3H), 2.81 (t, J = 7 Hz, 4H), 1.72–1.63 (m, 4H), 1.40–1.24 (m, 20H), 0.83 (m, 6H); 13C NMR (DMSO-d6, 126 MHz) δ/ppm = 164.04, 144.04, 143.06, 141.65, 140.43, 138.49, 134.40, 133.08, 129.29, 127.44, 126.77, 126.22, 124.47, 123.93, 122.99, 121.03, 119.75, 110.21, 109.87, 99.00, 31.73, 31.71, 30.31, 29.97, 29.56, 29.39, 29.18, 29.13, 29.07, 28.97, 22.53, 14.36; ATR-FTIR ν in cm−1 = 2920, 2850, 2216, 1680, 1563, 1397, 1267, 1248, 1211, 1169, 1152, 931, 870, 789, 742, 723, 650.
3.2.6. Synthesis of compound 5.
To a stirred solution of thieno[3,2-b]thiophene (500 mg, 3.6 mmol) in 20 mL dry THF, 2.0 M n-BuLi in hexane (1.78 mL, 3.6 mmol) was added at −78 °C under an Ar atmosphere. After 1.5 h, 2-isopropoxy-4,4,5,5-tetramethyl-4,3,2-dioxaboralane (0.8 mL, 3.9 mmol) was added and the reaction was allowed to warm to room temperature. After 12 h of stirring, the reaction was quenched with saturated NH4Cl solution. The reaction mixture was extracted using dichloromethane and water, dried over anhydrous Na2SO4 and purified by column chromatography over silica gel (100–200 mesh) using a mixture of ethyl acetate and hexane (1
:
20, v/v) as the eluent to afford product 5 as a yellow solid. Yield 300 mg (30%). Mass (ESI MS): 266.0606, found: 267.0687 (MH+); 1H NMR (CDCl3, 500 MHz) δ/ppm = 7.76 (s, 1H), 7.48 (d, J = 5.5 Hz, 1H), 7.28 (d, J = 5.5 Hz, 1H), 1.36 (s, 12H); 13C NMR (CDCl3, 126 MHz) δ/ppm = 145.58, 140.83, 130.02, 128.98, 119.49, 84.30, 30.94, 24.77; IR (KBr) ν in cm−1 = 3103, 3084, 2976, 2933, 1506, 1460, 1357, 1265, 1139, 1018, 958, 906, 850, 827, 771, 665.
3.2.7. Synthesis of compound 6.
To a solution of compound 2-bromo-3-octylthiophene (500 mg, 1.8 mmol) and N,N-dimethylformamide (1.4 mL, 18 mmol) in 1,2-dichloroethane (20 mL), POCl3 (0.3 mL, 3.2 mmol) was slowly added at 0 °C and stirred for 1 h under an argon atmosphere. Then, the reaction mixture was heated to 60 °C and stirred overnight. After cooling to room temperature, it was neutralized with NaHCO3 (aq.), extracted using chloroform and dried over anhydrous Na2SO4. The crude product was purified by column chromatography over silica gel (100–200 mesh) using chloroform:hexane (1
:
20) as the eluent to afford product 6 as an orange oil. Yield 500 mg (90%). Mass (ESI MS): 302.0339, found: 303.0428 (MH+), 305.0407 ((M + 2) H+); 1H NMR (500 MHZ, CDCl3) δ/ppm = 9.75 (s, 1H), 7.46 (s, 1H), 2.59 (t, J = 7.75 Hz, 2H), 1.62–1.59 (m, 2H), 1.32–1.23 (m, 10H), 0.88 (t, J = 6.5 Hz, 3H); 13C NMR (DMSO-d6, 126 MHz) δ/ppm = 177.16, 139.24, 138.13, 132.06, 117.38, 27.10, 24.73, 24.72,24.57,24.45, 24.39, 17.91, 9.36; IR (KBr) ν in cm−1 = 3089, 2956, 2922, 2870, 1665, 1600, 1531, 1456, 1384, 1253, 1120, 1043, 867, 798, 675.
3.2.8. Synthesis of compound 7.
A solution containing compound 5 (600 mg, 2.3 mmol), compound 6 (700 mg, 2.3 mmol) and K2CO3 (934 mg, 6.8 mmol) in toluene (20 mL) was subjected to the freeze pump thaw method to remove oxygen and moisture. Then, a palladium catalyst [Pd(PPh3)4] (30 mg, 0.03 mmol) was added to it and stirred at 110 °C for 48 h. The mixture was poured into water and extracted using CHCl3. The combined organic layer was washed with water, dried with anhydrous sodium sulphate and purified by column chromatography over silica gel (100–200 mesh) using chloroform and hexane (2
:
3) to give compound 7 as a yellow solid. Yield 483 mg (58%). Mass (ESI MS): 362.0832, found: 363.0914 (MH+); 1H NMR (500 MHz, CDCl3) δ/ppm = 9.84 (s, 1H), 7.61 (s, 1H), 7.44 (d, J = 8 Hz, 2H), 7.27 (d, J = 6 Hz, 1H), 2.83 (t, J = 7.75 Hz, 2H), 1.70–1.67 (m, 2H), 1.41–1.26 (m, 10H), 0.88 (t, J = 7 Hz, 3H); 13C NMR (CDCl3, 126 MHz) δ/ppm = 182.64, 141.39, 140.87, 140.82, 140.40, 139.62, 138.93, 128.53, 119.64, 119.41, 31.85, 30.43, 29.46, 29.38, 29.32, 29.22, 22.67, 14.12; IR (KBr) ν in cm−1 = 3130, 3082, 2943, 2926, 2848, 1651, 1525, 1496, 1465, 1417, 1386, 1336, 1246, 1165, 956, 871, 812, 742, 707, 682, 640.
3.2.9. Synthesis of compound 8.
To a stirred solution of 1.3 g of 7 (3.58 mmol) in acetic acid and dry chloroform (1
:
1, v/v), NBS (637 mg, 3.58 mmol) was added and stirred for 2 h in the dark at 25 °C. The reaction was quenched using water and sodium bicarbonate solution, extracted with chloroform, washed with water, dried over anhydrous sodium sulphate and purified by column chromatography over silica gel (100–200 mesh) using chloroform and hexane (2
:
3) as the eluent to give compound 8 as a yellow solid. Yield 1.09 g (69%). Mass (ESI MS): 439.9937, found: 441.0019 (MH+), 442.9996 ((M + 2) H+); 1H NMR (500 MHZ, CDCl3) δ/ppm = 9.84 (s, 1H), 7.61 (s, 1H), 7.33 (s, 1H), 7.27 (s, 1H), 2.80 (t, J = 7.75 Hz, 2H), 1.69–1.61 (m, 2H), 1.39–1.25 (m, 10H), 0.88 (t, J = 7 Hz, 3H); 13C NMR (CDCl3, 126 MHz) δ/ppm = 182.62, 141.05, 140.97, 140.71, 139.76, 139.13, 138.92, 135.95, 122.08, 119.10, 115.03, 31.85, 30.39, 29.44, 29.37, 29.33, 29.22, 22.67, 14.13; IR (KBr) ν in cm−1 = 3097, 2956, 2922, 2852, 1662, 1541, 1490, 1467, 1409, 1390, 1330, 1246, 1159, 987, 869, 802, 744, 677.
3.2.10. Synthesis of compound 9.
To a solution of compound 8 (500 mg, 1.13 mmol), K2CO3 (276 mg in 1 mL water, 2 M), 9-methyl-9H-carbazole-3-boronic acid pinacol ester (347 mg, 1.13 mmol) was added. Then 20 mL of toluene was added to it and the entire system was subjected to the freeze pump thaw method to remove the dissolved oxygen. Then, Pd(PPh3)4 (30 mg, 0.03 mmol) was added under an argon atmosphere and refluxed for 18 h. The reaction mixture was extracted with chloroform and purified by column chromatography over silica gel (100–200 mesh) using dichloromethane and hexane (1
:
4, v/v) as eluent to yield compound 9 as a red solid. Yield 530 mg (84%). Mass (ESI MS): 541.1567, found: 542.1649 (MH+); 1H NMR (500 MHz, CDCl3) δ/ppm = 9.84 (s, 1H), 8.35 (d, J = 1.5 Hz, 1H), 8.15 (d, J = 7.5 Hz, 1H), 7.78 (dd, J = 2 Hz, 1H) 7.62 (s, 1H), 7.54–7.51 (m, 2H), 7.45–7.42 (m, 3H), 7.29 (d, J = 7.5 Hz, 1H), 3.89 (s, 3H), 2.86 (t, J = 8 Hz, 2H), 1.75–1.68 (m, 2H), 1.43–1.25 (m, 10H), 0.89 (t, J = 6.75 Hz, 3H); 13C NMR (CDCl3, 126 MHz) δ/ppm = 182.58, 149.31, 141.80, 141.55, 141.52, 140.94, 140.52, 139.11, 138.03, 135.07, 126.30, 125.57, 124.20, 123.34, 122.62, 120.53, 119.84, 119.40, 117.92, 113.98, 108.97, 108.78, 31.88, 30.39, 29.71, 29.51, 29.41, 29.26, 26.93, 22.69, 14.14, 14.11; IR (KBr) ν in cm−1 = 3097, 3080, 2961, 2921, 2849, 1663, 1534, 1491, 1467, 1409, 1390, 1331, 1261, 1245, 1156, 897, 866, 842, 803, 745, 677.
3.2.11. Synthesis of YK 9.
Compound 9 (300 mg, 0.55 mmol) and cyanoacetic acid (234 mg, 2.75 mmol) were dissolved in 20 mL dry chloroform. Then, two drops of piperidine were added to this and stirred at 45 °C for 40 h. The reaction mixture was extracted with chloroform, washed with water, dried over anhydrous Na2SO4 and purified by column chromatography using chloroform and methanol (9
:
1, v/v) as the eluent to give compound YK 9. Yield 287 mg (86%). Mass (ESI MS): 608.1625, found: 609.1684 (MH+); 1H NMR (500 MHz, DMSO-d6) δ/ppm = 8.49 (s, 1H), 8.24 (d, 1H, 7.5 Hz), 8.15 (s, 1H), 7.86 (s, 1H), 7.79 (d, J = 8 Hz, 1H), 7.69 (d, J = 6.6 Hz, 2H), 7.63–7.59 (m, 2H) 7.52–7.49 (m, 1H), 7.26–7.23 (m, 1H), 3.87 (s, 3H), 2.78 (t, J = 7.5 Hz, 2H), 1.61 (t, J = 7 Hz 2H), 1.32–1.19 (m, 10H), 0.81 (t, J = 6.5 Hz, 3H); 13C NMR (DMSO-d6, 126 MHz) δ/ppm = 164.25, 148.84, 141.63, 141.18, 140.96, 140.37, 138.20, 134.88, 134.39, 127.49, 126.80, 125.32, 124.17, 123.01, 122.28, 121.10, 120.73, 119.70, 117.74, 117.12, 115.09, 110.34, 109.92, 31.72, 29.97, 29.57, 29.14, 29.07, 28.86, 22.54, 14.41; ATR-FTIR ν in cm−1 = 2923, 2852, 2212, 1680, 1574, 1478, 1385, 1366, 1300, 1249, 796, 766, 745, 726, 674.
3.3. Device fabrication and characterization
Fluorine-doped tin oxide (FTO, TEC 15 Ω Sq−1 cm−1) glass (GreatCell Solar) were used as the substrate. The substrates were cleaned in detergent, deionized water, isopropyl alcohol, and acetone using an ultrasonic bath. Subsequently, the electrodes were annealed at 500 °C, followed by UV-ozone cleaning. The cleaned electrodes were immersed in 40 mM TiCl4 at 70 °C for 30 min to coat an ultra-thin blocking layer over FTO. Then the substrates were rinsed in de-ionized water and ethanol, followed by annealing at 500 °C. A TiO2 layer was coated using the doctor-blading technique. After the deposition of TiO2, the substrates were heat-treated at a ramped heating rate (325 °C for 15 min, 375 °C for 15 min, 450 °C for 15 min and 500 °C for 30 min). Devices with two different TiO2 (GreatCell Solar) layer architectures, i.e., single layer (1L) and bilayer (1L + S), were fabricated. 1L contained a 6 μm TiO2 (18 NR-T) transparent layer, and 1L + S contained 10 μm TiO2 (6 μm 18 NR-T and 4 μm scattering 18 NR-AO) layer. The optimized semiconductor layer thickness was 1L (6 μm) for the best-performing devices. Further, a blocking layer over TiO2 was deposited by adopting the same procedure used for deposition of the pre-blocking layer. The electrodes were immersed in the respective dye solutions (YK 8, YK 9 and MK 2) with a dye concentration of 0.3 mM in toluene for 15 h. For the counter electrode (CE), platinum (Platisol, GreatCell Solar) was coated on pre-drilled fluorine-doped tin oxide (TEC 7 Ω Sq−1 cm−1) glass using a painting brush, and then annealed at 400 °C for 30 min. The working electrode and counter electrode were assembled using 25 μm-thick Surlyn. The space between the electrodes was filled using the standard I−/I3− electrolyte (HPE, GreatCell Solar). The holes at the counter electrode were sealed using a cover glass.
The thickness of the semiconductor layer (TiO2) was measured using a Bruker-DektakXT surface profiler. The current density versus voltage (J–V) curve of the fabricated DSCs was measured under AM1.5G irradiation (100 mW cm−2) using a Newport class AAA solar simulator (Fig. S16 (ESI†), Oriel Sol3A-94023A) calibrated with a standard silicon solar cell (Fig. S17, ESI†) at room temperature. To avoid the overestimation of Jsc due to stray light, during all the photovoltaic characterizations, we used a black mask with an aperture of 0.1256 cm2 (radius of 2 mm) over the device with a total active area of 0.31 cm2. Also, all the photovoltaic results reported are from the data obtained from 8 devices, and the error was estimated using the standard deviation method. For the stability measurements, the devices were kept at open circuit at room temperature (27 °C) under >70% humidity condition, and the measurements were done at equal intervals (500 h) using a class AAA Newport solar simulator. The incident photon-to-current conversion efficiency (IPCE) measurements were carried out in DC mode (Newport, 350 W Xenon lamp, Fig. S18 and S19, ESI†). The low-light (indoor-light) measurements were performed in a home-made indoor light measurement setup (Fig. S14, ESI†) using an Osram 14 W T2 cool daylight fluorescent tube as the illumination source ranging between zero and 2000 lux. The light intensity was calibrated and measured using a high sensitivity certified irradiance measuring unit from Dyenamo AB (DN-AE06). The J–V measurements under lower intensities were performed using a certified low power measuring unit from Dyenamo AB, which was custom made for carrying out low-light (indoor ambient light) measurements with maximum accuracy (DN-AE05). Electrochemical impedance spectroscopy (EIS) measurements of the DSCs were carried out using an Autolab (PGSTAT 302N) workstation equipped with an FRA under forward bias in the dark. The impedance plots were recorded from 0.72 V to 0.80 V, with 200 mV increments and corrected for the voltage drop due to series resistance for representing the derived parameter from EIS. The perturbation bias was 10 mV AC potential in the frequency range of 0.1 Hz to 100 kHz in equally spaced logarithmic steps. The circuit fitting was carried out using the NOVA 1.11 software. Intensity-modulated photovoltage spectroscopy (IMVS) measurements were carried out using an electrochemical workstation (PGSTAT 302N) equipped with an FRA and LED driver. The photovoltage response of the devices was recorded in the frequency range of 1 Hz to 1 kHz. The amplitude of the sinusoidal modulation for IMVS measurement was 10% of the DC light. PIA was carried out using equipment custom developed by Dynamo AB (Sweden), which uses a 1 W blue light-emitting LED (λ = 470 nm) as the pump and 20 W tungsten halogen lamp as the probe. The transmitted light through the sample is focused on a monochromator and detected by a UV-enhanced Si detector connected to a lock-in amplifier (SR830) via a current amplifier. The PIA spectra of the devices were recorded using excitation by LED, which was a square wave with a modulation frequency of 9.3 Hz. For the PIA measurements, the TiO2 thickness was kept at 6 μm.
4. Conclusions
In conclusion, the YK 8 dye with bis-octyl terthiophene proved to be a good candidate in preventing recombination compared to the mono-octyl-substituted thiophene spacer in the YK 9 dye. Both dyes were exceptionally stable and delivered their best performance after ageing for 4 months. Using 1000 lux standard CFL illumination, YK 8 realized a PCE of 28.7% and YK 9 exhibited a PCE of 14.21% using the conventional iodide/triiodide electrolyte devoid of any co-sensitizers. A maximum PCE of 30.24% was exhibited by the YK 8 dye at a higher illumination intensity of 1500 lux with a power output of 108.85 μW cm−2 under Osram T2 cool daylight fluorescent tube illumination. YK 9 displayed a PCE of 17.84% under 1500 lux fluorescent tube illumination. The better lifetime by virtue of the additional octyl chains present in the terthiophene π-spacer along with the added advantage of proper molecular orientation and precise control over aggregation effectively impeded recombination in the YK 8 dye. This translated into higher open circuit potential and improved light-harvesting ability in the visible region for YK 8. The close match of the YK 8 absorption with the fluorescent tube spectra also led to an improved Jsc compared to YK 9. Additionally, its better diffusion length, improved charge collection efficiency and relatively higher injection and regeneration yield helped YK 8 dye to outperform YK 9 and commercial MK 2 metal-free sensitizer both under indoor light and full sun conditions. To demonstrate the potential of these artificial light harvesting solar cells, mini-modules were fabricated by serially interconnecting DSCs sensitized with the YK 8 dye. The serially connected module with a total active area of 1.24 cm2 generated sufficient power even at a very low light intensity of 500 lux fluorescent lamp illumination to realize a completely self-powered temperature sensor with an LCD display, unveiling the real potential of these custom-designed dyes for indoor photovoltaics. The scale-up capability of these organic dyes at a lower cost further suggests their potential widespread application in indoor light harvesting.
Author contributions
Dyes were designed and characterized by KY, RH and AV. Photovoltaic measurements were designed by SS. Solar cells fabricated, measured and analysed by VJ and SCP. SS, KNNU and AA supervised the project. SS wrote the manuscript with inputs from all authors.
Conflicts of interest
There are no conflicts of interest to declare.
Acknowledgements
S. S., N. U. K. N., Y. K. and A. A. thank DST for the support under DST-SERB Project [DST/SERB/F/481]. A. A. is grateful to the DST-SERB, Govt. of India, for a J. C. Bose National Fellowship [SB/S2/JCB-11/2014]. S. S. acknowledges financial support from DST-CRG (CRG/2020/001406) and CSIR-FIRST (MLP65) projects. J. V. and S. C. P. thank DST-SERB and DST-SERI for research fellowships. R. H. thanks CSIR for research fellowship. Prof. Anders Hagfeldt is acknowledged for the scientific discussions and feedbacks.
References
-
World Energy Issues Monitor 2020, 2020.
-
BP Statistical Review of World Energy, 2019.
- L. Roselli, N. Borges Carvalho, F. Alimenti, P. Mezzanotte, G. Orecchini, M. Virili, C. Mariotti, R. Goncalves and P. Pinho, Proc. IEEE, 2014, 102, 1723–1746 Search PubMed.
- E. Hittinger and P. Jaramillo, Science, 2019, 364, 326–328 CrossRef CAS PubMed.
- H. Sun, M. Yin, W. Wei, J. Li, H. Wang and X. Jin, Microsyst. Technol., 2018, 24, 2853–2869 CrossRef CAS.
- M. Prauzek, J. Konecny, M. Borova, K. Janosova, J. Hlavica and P. Musilek, Sensors, 2018, 18, 2446 CrossRef PubMed.
- R. Haight, W. Haensch and D. Friedman, Science, 2016, 353, 124–125 CrossRef CAS PubMed.
- A. Hagfeldt, G. Boschloo, L. Sun, L. Kloo and H. Pettersson, Chem. Rev., 2010, 110, 6595–6663 CrossRef CAS PubMed.
- B. P. Lechêne, M. Cowell, A. Pierre, J. W. Evans, P. K. Wright and A. C. Arias, Nano Energy, 2016, 26, 631–640 CrossRef.
- I. Mathews, S. N. Kantareddy, T. Buonassisi and I. M. Peters, Joule, 2019, 3, 1415–1426 CrossRef CAS.
- R. Cheng, C. Chung, H. Zhang, F. Liu, W. Wang, Z. Zhou, S. Wang, A. B. Djurišić and S. Feng, Adv. Energy Mater., 2019, 9, 1901980 CrossRef CAS.
- G. Kim, J. W. Lim, J. Kim, S. J. Yun and M. A. Park, ACS Appl. Mater. Interfaces, 2020, 12, 27122–27130 CrossRef CAS PubMed.
- X. He, J. Chen, X. Ren, L. Zhang, Y. Liu, J. Feng, J. Fang, K. Zhao and S. (Frank) Liu, Adv. Mater., 2021, 33, 2100770 CrossRef CAS PubMed.
- M. Li, F. Igbari, Z. Wang and L. Liao, Adv. Energy Mater., 2020, 10, 2000641 CrossRef CAS.
- A. Virtuani, E. Lotter, M. Powalla, U. Rau and J. H. Werner, Thin Solid Films, 2004, 451–452, 160–165 CrossRef CAS.
- A. S. Teran, E. Moon, W. Lim, G. Kim, I. Lee, D. Blaauw and J. D. Phillips, IEEE Trans. Electron Devices, 2016, 63, 2820–2825 CAS.
- A. S. Teran, J. Wong, W. Lim, G. Kim, Y. Lee, D. Blaauw and J. D. Phillips, IEEE Trans. Electron Devices, 2015, 62, 2170–2175 CAS.
- Y. Cui, Y. Wang, J. Bergqvist, H. Yao, Y. Xu, B. Gao, C. Yang, S. Zhang, O. Inganäs, F. Gao and J. Hou, Nat. Energy, 2019, 4, 768–775 CrossRef CAS.
- Z. Ding, R. Zhao, Y. Yu and J. Liu, J. Mater. Chem. A, 2019, 7, 26533–26539 RSC.
- G. D. Barber, P. G. Hoertz, S. H. A. Lee, N. M. Abrams, J. Mikulca, T. E. Mallouk, P. Liska, S. M. Zakeeruddin, M. Grätzel, A. Ho-Baillie and M. A. Green, J. Phys. Chem. Lett., 2011, 2, 581–585 CrossRef CAS.
- H. Michaels, M. Rinderle, R. Freitag, I. Benesperi, T. Edvinsson, R. Socher, A. Gagliardi and M. Freitag, Chem. Sci., 2020, 11, 2895–2906 RSC.
- Y. Cao, Y. Liu, S. M. Zakeeruddin, A. Hagfeldt and M. Grätzel, Joule, 2018, 2, 1108–1117 CrossRef CAS.
- A. Venkateswararao, J. K. W. Ho, S. K. So, S.-W. Liu and K.-T. Wong, Mater. Sci. Eng., R, 2020, 139, 100517 CrossRef.
- Y. S. Tingare, N. S. Vinh, H.-H. Chou, Y.-C. Liu, Y.-S. Long, T.-C. Wu, T.-C. Wei and C.-Y. Yeh, Adv. Energy Mater., 2017, 7, 1700032 CrossRef.
- M. Freitag, J. Teuscher, Y. Saygili, X. Zhang, F. Giordano, P. Liska, J. Hua, S. M. Zakeeruddin, J.-E. E. Moser, M. Grätzel and A. Hagfeldt, Nat. Photonics, 2017, 11, 372–378 CrossRef CAS.
- Y. Liu, Y. Cao, W. Zhang, M. Stojanovic, M. I. Dar, P. Péchy, Y. Saygili, A. Hagfeldt, S. M. Zakeeruddin and M. Grätzel, Angew. Chem., Int. Ed., 2018, 57, 14125–14128 CrossRef CAS PubMed.
- D. Zhang, M. Stojanovic, Y. Ren, Y. Cao, F. T. Eickemeyer, E. Socie, N. Vlachopoulos, J.-E. Moser, S. M. Zakeeruddin, A. Hagfeldt and M. Grätzel, Nat. Commun., 2021, 12, 1777 CrossRef CAS PubMed.
- T. W. Hamann, R. A. Jensen, A. B. F. Martinson, H. Van Ryswyk and J. T. Hupp, Energy Environ. Sci., 2008, 1, 66–78 RSC.
- K. Zeng, Z. Tong, L. Ma, W. H. Zhu, W. Wu, Y. Xie and Y. Xie, Energy Environ. Sci., 2020, 13, 1617–1657 RSC.
- J. H. Yum, E. Baranoff, S. Wenger, M. K. Nazeeruddin and M. Grätzel, Energy Environ. Sci., 2011, 4, 842–857 RSC.
- M.-W. Lee, J.-Y. Kim, H. J. Son, J. Y. Kim, B. Kim, H. Kim, D.-K. Lee, K. Kim, D.-H. Lee and M. J. Ko, Sci. Rep., 2015, 5, 7711 CrossRef PubMed.
- A. Yella, H.-W. Lee, H. N. Tsao, C. Yi, A. K. Chandiran, M. K. Nazeeruddin, E. W.-G. Diau, C.-Y. Yeh, S. M. Zakeeruddin and M. Gratzel, Science, 2011, 334, 629–634 CrossRef CAS PubMed.
- S. Mathew, A. Yella, P. Gao, R. Humphry-Baker, B. F. E. Curchod, N. Ashari-Astani, I. Tavernelli, U. Rothlisberger, M. K. Nazeeruddin and M. Grätzel, Nat. Chem., 2014, 6, 242–247 CrossRef CAS PubMed.
- N. Robertson, Angew. Chem., Int. Ed., 2006, 45, 2338–2345 CrossRef CAS PubMed.
- K. Zeng, Y. Chen, W. H. Zhu, H. Tian and Y. Xie, J. Am. Chem. Soc., 2020, 142, 5154–5161 CrossRef CAS PubMed.
- Y. Kurumisawa, T. Higashino, S. Nimura, Y. Tsuji, H. Iiyama and H. Imahori, J. Am. Chem. Soc., 2019, 141, 9910–9919 CrossRef CAS PubMed.
- H. Song, Q. Liu and Y. Xie, Chem. Commun., 2018, 54, 1811–1824 RSC.
- S. Ahmad, E. Guillén, L. Kavan, M. Grätzel and M. K. Nazeeruddin, Energy Environ. Sci., 2013, 6, 3439–3466 RSC.
- Y. S. Yen, H. H. Chou, Y. C. Chen, C. Y. Hsu and J. T. Lin, J. Mater. Chem., 2012, 22, 8734–8747 RSC.
- A. Carella, F. Borbone and R. Centore, Front. Chem., 2018, 6, 1–24 CrossRef PubMed.
- A. Baumann, C. Curiac and J. H. Delcamp, ChemSusChem, 2020, 13, 2503–2512 CrossRef CAS PubMed.
- K. Kakiage, Y. Aoyama, T. Yano, K. Oya, J. I. Fujisawa and M. Hanaya, Chem. Commun., 2015, 51, 15894–15897 RSC.
- M. V. Vinayak, M. Yoosuf, S. C. Pradhan, T. M. Lakshmykanth, S. Soman and K. R. Gopidas, Sustainable Energy Fuels, 2018, 2, 303–314 RSC.
- M. S. Mikhailov, N. S. Gudim, E. A. Knyazeva, E. Tanaka, L. Zhang, L. V. Mikhalchenko, N. Robertson and O. A. Rakitin, J. Photochem. Photobiol., A, 2020, 391, 112333 CrossRef CAS.
- P. V. Santhini, V. Jayadev, S. C. Pradhan, S. Lingamoorthy, P. R. Nitha, M. V. Chaithanya, R. K. Mishra, K. N. Narayanan Unni, J. John and S. Soman, New J. Chem., 2019, 43, 862–873 RSC.
- A. Mishra, M. K. R. Fischer and P. Bäuerle, Angew. Chem., Int. Ed., 2009, 48, 2474–2499 CrossRef CAS PubMed.
- D. P. Hagberg, T. Edvinsson, T. Marinado, G. Boschloo, A. Hagfeldt and L. Sun, Chem. Commun., 2006, 2245–2247 RSC.
- S. M. Feldt, E. A. Gibson, E. Gabrielsson, L. Sun, G. Boschloo and A. Hagfeldt, J. Am. Chem. Soc., 2010, 132, 16714–16724 CrossRef CAS PubMed.
- D. Joly, L. Pellejà, S. Narbey, F. Oswald, T. Meyer, Y. Kervella, P. Maldivi, J. N. Clifford, E. Palomares and R. Demadrille, Energy Environ. Sci., 2015, 8, 2010–2018 RSC.
- Y. Ren, D. Sun, Y. Cao, H. N. Tsao, Y. Yuan, S. M. Zakeeruddin, P. Wang and M. Grätzel, J. Am. Chem. Soc., 2018, 140, 2405–2408 CrossRef CAS PubMed.
- P. R. Nitha, S. Soman and J. John, Mater. Adv., 2021, 2, 6136–6168 RSC.
- J. Zou, Q. Yan, C. Li, Y. Lu, Z. Tong and Y. Xie, ACS Appl. Mater. Interfaces, 2020, 12, 57017–57024 CrossRef CAS PubMed.
- K. Zeng, W. Tang, C. Li, Y. Chen, S. Zhao, Q. Liu and Y. Xie, J. Mater. Chem. A, 2019, 7, 20854–20860 RSC.
- Z. S. Wang, N. Koumura, Y. Cui, M. Takahashi, H. Sekiguchi, A. Mori, T. Kubo, A. Furube and K. Hara, Chem. Mater., 2008, 20, 3993–4003 CrossRef CAS.
-
M. J. Frisch, G. W. Trucks, H. B. Schlegel, G. E. Scuseria, M. A. Robb, J. R. Cheeseman, G. Scalmani, V. Barone, G. A. Petersson, H. Nakatsuji, X. Li, M. Caricato, A. V. Marenich, J. Bloino, B. G. Janesko, R. Gomperts, B. Mennucci, H. P. Hratchian, J. V. Ortiz, A. F. Izmaylov, J. L. Sonnenberg, Williams, F. Ding, F. Lipparini, F. Egidi, J. Goings, B. Peng, A. Petrone, T. Henderson, D. Ranasinghe, V. G. Zakrzewski, J. Gao, N. Rega, G. Zheng, W. Liang, M. Hada, M. Ehara, K. Toyota, R. Fukuda, J. Hasegawa, M. Ishida, T. Nakajima, Y. Honda, O. Kitao, H. Nakai, T. Vreven, K. Throssell, J. A. Montgomery Jr., J. E. Peralta, F. Ogliaro, M. J. Bearpark, J. J. Heyd, E. N. Brothers, K. N. Kudin, V. N. Staroverov, T. A. Keith, R. Kobayashi, J. Normand, K. Raghavachari, A. P. Rendell, J. C. Burant, S. S. Iyengar, J. Tomasi, M. Cossi, J. M. Millam, M. Klene, C. Adamo, R. Cammi, J. W. Ochterski, R. L. Martin, K. Morokuma, O. Farkas, J. B. Foresman and D. J. Fox, Gaussian16, 2016 Search PubMed.
- A. D. Becke, J. Chem. Phys., 1993, 98, 5648–5652 CrossRef CAS.
- C. Koenigsmann, T. S. Ripolles, B. J. Brennan, C. F. A. Negre, M. Koepf, A. C. Durrell, R. L. Milot, J. A. Torre, R. H. Crabtree, V. S. Batista, G. W. Brudvig, J. Bisquert and C. A. Schmuttenmaer, Phys. Chem. Chem. Phys., 2014, 16, 16629–16641 RSC.
- P. Kumar and S. K. Pal, Phys. Chem. Chem. Phys., 2016, 18, 29571–29581 RSC.
- P. Li, C. Song, Z. Wang, J. Li and H. Zhang, New J. Chem., 2018, 42, 12891–12899 RSC.
- C. T. Weisspfennig, D. J. Hollman, C. Menelaou, S. D. Stranks, H. J. Joyce, M. B. Johnston, H. J. Snaith and L. M. Herz, Adv. Funct. Mater., 2014, 24, 668–677 CrossRef CAS.
- J. K. Park, J. C. Kang, S. Y. Kim, B. H. Son, J. Y. Park, S. Lee and Y. H. Ahn, J. Phys. Chem. Lett., 2012, 3, 3632–3638 CrossRef CAS PubMed.
- K. Zhu, S. R. Jang and A. J. Frank, J. Phys. Chem. Lett., 2011, 2, 1070–1076 CrossRef CAS.
- A. C. Fisher, L. M. Peter, E. A. Ponomarev, A. B. Walker and K. G. U. Wijayantha, J. Phys. Chem. B, 2000, 104, 949–958 CrossRef CAS.
- P. R. F. Barnes, A. Y. Anderson, S. E. Koops, J. R. Durrant and B. C. O’Regan, J. Phys. Chem. C, 2009, 113, 1126–1136 CrossRef CAS.
- J. Halme, P. Vahermaa, K. Miettunen and P. Lund, Adv. Mater., 2010, 22, E210–E234 CrossRef CAS PubMed.
- D. Cahen, G. Hodes, M. Grätzel, J. F. Guillemoles and I. Riess, J. Phys. Chem. B, 2000, 104, 2053–2059 CrossRef CAS.
- F. Fabregat-Santiago, I. Mora-Seró, G. Garcia-Belmonte and J. Bisquert, J. Phys. Chem. B, 2003, 107, 758–768 CrossRef CAS.
- P. S. Archana, R. Jose, M. M. Yusoff and S. Ramakrishna, Appl. Phys. Lett., 2011, 98, 2011–2014 CrossRef.
- S. C. Pradhan, A. Hagfeldt and S. Soman, J. Mater. Chem. A, 2018, 6, 22204–22214 RSC.
- J. Idïgoras, G. Burdzinski, J. Karolczak, J. Kubicki, G. Oskam, J. A. Anta and M. Ziöłek, J. Phys. Chem. C, 2015, 119, 3931–3944 CrossRef.
- J. R. Jennings, Y. Liu, Q. Wang, S. M. Zakeeruddin and M. Grätzel, Phys. Chem. Chem. Phys., 2011, 13, 6637–6648 RSC.
- M. S. Góes, E. Joanni, E. C. Muniz, R. Savu, T. R. Habeck, P. R. Bueno and F. Fabregat-Santiago, J. Phys. Chem. C, 2012, 116, 12415–12421 CrossRef.
- F. Fabregat-Santiago, J. Bisquert, G. Garcia-Belmonte, G. Boschloo and A. Hagfeldt, Sol. Energy Mater. Sol. Cells, 2005, 87, 117–131 CrossRef CAS.
- J. Bisquert, J. Phys. Chem. B, 2002, 106, 325–333 CrossRef CAS.
- S. Soman, S. C. Pradhan, M. Yoosuf, M. V. Vinayak, S. Lingamoorthy and K. R. Gopidas, J. Phys. Chem. C, 2018, 122, 14113–14127 CrossRef CAS.
- J. W. Ondersma and T. W. Hamann, J. Phys. Chem. C, 2010, 114, 638–645 CrossRef CAS.
- E. M. Barea, V. Gónzalez-Pedro, T. Ripollés-Sanchis, H. P. Wu, L. L. Li, C. Y. Yeh, E. W. G. Diau and J. Bisquert, J. Phys. Chem. C, 2011, 115, 10898–10902 CrossRef CAS.
- G. Boschloo and A. Hagfeldt, Chem. Phys. Lett., 2003, 370, 381–386 CrossRef CAS.
- G. Boschloo and A. Hagfeldt, Inorg. Chim. Acta, 2008, 361, 729–734 CrossRef CAS.
- W. Yang, N. Vlachopoulos and G. Boschloo, ACS Energy Lett., 2017, 2, 161–167 CrossRef CAS.
- U. B. Cappel, S. M. Feldt, J. Schöneboom, A. Hagfeldt and G. Boschloo, J. Am. Chem. Soc., 2010, 132, 9096–9101 CrossRef CAS PubMed.
- M. Pazoki, A. Hagfeldt and G. Boschloo, Electrochim. Acta, 2015, 179, 174–178 CrossRef CAS.
Footnotes |
† Electronic supplementary information (ESI) available. See DOI: 10.1039/d1ma00829c |
‡ These authors contributed equally to this work. |
|
This journal is © The Royal Society of Chemistry 2021 |
Click here to see how this site uses Cookies. View our privacy policy here.