DOI:
10.1039/D1NA00680K
(Paper)
Nanoscale Adv., 2021,
3, 7002-7006
Morphology effect of ceria supports on gold nanocluster catalyzed CO oxidation
Received
10th September 2021
, Accepted 5th October 2021
First published on 7th October 2021
Abstract
The interfacial perimeter is generally viewed as the catalytically active site for a number of chemical reactions over oxide-supported nanogold catalysts. Here, well-defined CeO2 nanocubes, nanorods and nanopolyhedra are chosen to accommodate atomically precise clusters (e.g. Au25(PET)18) to give different Au cluster–CeO2 interfaces. TEM images show that Au particles of ∼1.3 nm are uniformly anchored on the ceria surface after annealing in air at 120 °C, which can rule out the size hierarchy of nanogold in CO oxidation studies. The gold nanoclusters are only immobilized on the CeO2(200) facet in Au25/CeO2-C, while they are selectively loaded on CeO2(002) and (111) in the Au25/CeO2-R and Au25/CeO2-P catalysts. X-ray photoelectron spectroscopy (XPS) and in situ infrared CO adsorption experiments clearly demonstrate that the gold species in the Au25/CeO2 samples are similar and partially charged (Auδ+, where 0 < δ < 1). It is observed that the catalytic activity decreases in the order of Au/CeO2-R ≈ Au/CeO2-P > Au/CeO4-C in the CO oxidation. And the apparent activation energy over Au25/CeO2-C (60.5 kJ mol−1) is calculated to be about two-fold of that over the Au25/CeO2-R (28.6 kJ mol−1) and Au25/CeO2-P (31.3 kJ mol−1) catalysts. It is mainly tailored by the adsorbed [O] species on the ceria surface, namely, Au25/CeO2(002) and Au25/CeO2(111) which were more active than the Au25/CeO2(200) system in the CO oxidation. These insights at the molecular level may provide guidelines for the design of new oxide-supported nanogold catalysts for aerobic oxidations.
Introduction
Since the discovery of active nanogold for efficient CO oxidation (CO + 0.5 O2 → CO2) by Haruta et al. in 1987, it has ignited substantial interest in exploring gold nanoparticles (NPs) as a heterogeneous catalyst for a variety of gas and organic reactions.1–5 Initially, Au NPs were simply loaded on the surface of metal oxides (e.g., TiO2, CeO2, Fe3O4, etc.) via deposition–precipitation and co-precipitation methods in a basic system (e.g. pH: 8–10).6 The preferential conversion of trace CO (typically containing <1 v% CO with >99 v% H2) is a highly desirable technique for the CO removal in the industrial hydrogen production under mild conditions. It can reduce H2 consumption and energy loss, as well as prevent the poisoning of the Pt catalyst by CO in the fuel cell.7
Although CO oxidation, as a probe reaction to examine the activity for nanogold catalysts, has been widely investigated for over thirty years, the catalytic mechanism is indeed complicated and has not been fully elucidated yet. The valence state of surface Au atoms, the size of Au particles, and the nature of the support oxide as well as the interaction between Au NPs and the support, can intensely affect the activity of CO oxidation.8 Usually, carbon monoxide and dioxygen are deemed to be adsorbed and activated on the surface of naked gold NPs and lattice oxygen of reducible oxides in the Mars–van Krevelen mechanism, respectively.9 And then the activated CO and [O] species move toward each other and react at the perimeter sites of the interface of the oxide-supported nanogold.
Thus, the morphology of the oxides plays an important role in the catalytic processes.10–13 The gold particles loaded on the nanocrystalline CeO2 were explored; it is observed that the gold particles on CeO2-rods with {100}/{110} facets gave the best performance.14 Of note, ceria is a reducible oxide, exhibiting unique redox properties and high oxygen storage capacity, and has been widely applied as an excellent support for Au catalysts.15–18 However, the size of the Au NPs is not uniform on the different shaped ceria supports. The size-hierarchy is also a key factor in the CO oxidation over nanogold catalysts.8,19 Thus, controlling the well-defined oxide supports and uniform gold NPs is a big challenge in the fundamental research and mechanism study.
Recently, the breakthrough progress of synthesis of atomically precise gold clusters capped by organic ligands (e.g., thiolate) provided a good platform to study the model system (e.g., uniform gold particles) at the molecular or atomic levels.20,21 These Au clusters have been exploited in gas oxidations (e.g., CO oxidation) and a series of organic transformation reactions.22–25 In our previous studies, the capping ligands of the gold clusters (on the surface of ceria) were partially detached under 120 °C annealing (in air), leading to the exposure of gold atoms which interact with the oxide surface.26 The reactants (e.g., CO) can only be adsorbed and reacted at the perimeter sites of the oxides and nanogold species, as the other surface gold atoms are fully capped with the organic ligands, which can well block off the CO adsorption.
Herein, we introduce a model of the nanogold catalyst: the atomically precise gold clusters (Au25(PET)18, PET: phenylethanethiolate) are anchored onto the surface of three well-shaped ceria materials (nanorods, nanocubes and nanopolyhedra). The Au25 clusters are intact after the 120 °C pretreatment to rule out the size hierarchy of nanogold in the CO oxidation tests. An Au25/CeO2(200) interface is formed in the Au25/CeO2-C catalyst. And Au25/CeO2(002) and (111) are found in both Au/CeO2-R and Au/CeO2-P. The nanogold of Au25 nanoclusters on the CeO2 nanorods and nanopolyhedra shows a similar activity and better than that on the CeO2 nanocubes in the CO oxidation, which is largely associated with the concentration of the [O] species on the ceria surface.
Experimental
Immobilization of clusters onto CeO2
Well-defined ceria materials with different morphologies (nanocube, nanorod and nanopolyhedra) were prepared according to the reported protocols.27–29 The Au25(PET)18 clusters are synthesized via the reported literature.30 Typically, 2 g CeO2 oxide was dispersed in 30 mL ethyl acetate, and then 10 mg Au25(PET)18 cluster (in 10 mL CH2Cl2) was drop-wise added into the EtOAc solution. After stirring for 2 h, the solids were collected by centrifugation, dried in a vacuum, and treated at 120 °C in air, and were denoted as Au25/CeO2-C, Au25/CeO2-R and Au25/CeO2-P. ICP-MS showed that the gold content of the Au25/CeO2-C, Au25/CeO2-R and Au25/CeO2-P was 0.25%, 0.31%, and 0.23%.
Catalyst characterization
The specific surface area of the samples was measured on a Micromeritics ASAP 2020 surface area analyzer. Inductively coupled plasma-mass spectrometry (ICP-MS) was performed on a PerkinElmer ICP-MS NexION 300D. TEM and energy dispersive X-ray spectroscopy (EDX) were performed on a Hitachi 7000 transmission electron microscope operated at 75 kV. X-ray photoelectron spectroscopy (XPS) analysis was performed on a VG ESCAB mk-2.
Catalytic test for CO oxidation
The catalytic activity of these supported nanogold catalysts was evaluated in a fixed bed, continuous flow quartz reactor with gas hourly space velocity (GHSV) ranging from 15
000 mL g−1 h−1. In a typical experiment, 50 mg catalysts were heated to 120 °C at a heating rate of 5 °C min−1 in an O2 flow (30 mL min−1) and were kept for 2 h to remove the adsorbed species (e.g., CO2). And then the reactor was spontaneously cooled to ambient temperature before switching to the reactant gas mixture consisting of 1 v% CO, 20 v% O2, and 79 v% N2. The flows of inlet gases were controlled by using mass-flow controllers. The catalyst was conditioned for 0.5 h in this mixture at ambient temperature before the products were analyzed and determined by using an online gas chromatograph and a thermal conductivity detector. Analogous measurements were performed in 20 ° C intervals from room temperature to 120 °C. And then, the temperature was dropped to 100 °C and kept for ca. 13 h for the durability investigation.
Results and discussion
Characterization of Au25/CeO2
TEM images showed that the Au25 clusters were loaded on the surface of CeO2 (Fig. 1). The size of the Au clusters was retained at ca. 1.3 nm in all three samples (Au25/CeO2-C, Au25/CeO2-R and Au25/CeO2-P), consistent with our previous studies.31 Of note, a few protecting thiolate ligands (ca. 3–4 thiolate) are detached during the 120 °C annealing process in air, which has been investigated in our previous results.26
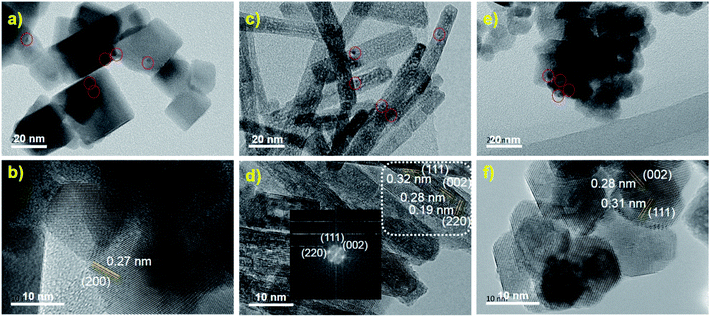 |
| Fig. 1 TEM images of the (a and b) Au25/CeO2-C, (c and d) Au25/CeO2-R, and (e and f) Au25/CeO2-P catalysts. The gold clusters are highlighted in the red rings, and the particle size is about 1.3 nm, consistent with the size of the unsupported Au25(PET)18 parent clusters. The inset in (d) is the electron diffraction diagram. | |
The size of the nanocube-like CeO2 oxides is approximately 27.5 ± 12 nm, and only the CeO2(200) facet was found in the Au25/CeO2-C sample, as shown in Fig. 1a and b. And Au25 clusters were loaded onto the (200) facet. In the case of Au25/CeO2-P, the oxide particle is ca. 10.9 ± 2.7 nm, and two facets of CeO2(002) and (111) were observed, which agrees well with the reported results.27–29 The clusters are attached on both the CeO2 facets (Fig. 1e and f). With regard to Au25/CeO2-R, the length and width of the oxides are 50–200 nm and 8–13 nm, and three facets of CeO2(002), (111) and (220) are discovered in the TEM analysis. Similar to Au25/CeO2-P, the Au25 particles are found to be immobilized on the CeO2(002) and (111) facets, as shown in Fig. 1c and d. And we applied EDX analysis to confirm whether the Au25(PET)18 nanoclusters are immobilized onto the ceria surface. As shown in Fig. 2, gold (Au), sulfur (S), oxygen (O), and cerium (Ce) elements are observed in the Au25/CeO2 catalysts, suggesting that the Au25 clusters should be intact after wet-deposition onto the CeO2 support.
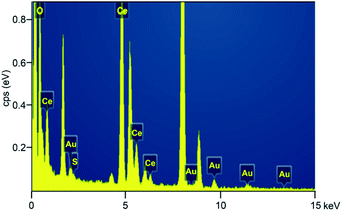 |
| Fig. 2 EDX analysis of the Au25/CeO2 catalysts. | |
Further, these as-prepared Au25/CeO2 samples were evaluated by the BET analyses and powder X-ray diffraction (XRD). Five prominent diffraction lines at 28.7, 33.3, 47.8, 56.8 and 59.6° were found in the XRD patterns of all the Au25/CeO2-P, Au25/CeO2-R, and Au25/CeO2-C samples (Fig. 3a), which were assigned to the CeO2 facets of (111), (200), (220), (311) and (222) (PDF #34-0394). It implied that these nanocube-, nanorod- and nanopolyhedra-like ceria materials exhibited the same phase composition. No diffraction is found for the gold clusters, which is mainly due to the small-sized particles and the low gold loading concentration (Au: 0.23–0.31 wt%). Next, Fig. 3b shows that the BET value for Au25/CeO2-R, Au25/CeO2-P and Au25/CeO2-C is 86.0, 72.5 and 36.2 m2 g−1, respectively. Au25/CeO2-R and Au25/CeO2-P showed a larger surface area than Au25/CeO2-C, which is caused by the size of CeO2 oxides and mainly by the hydroxyl species on ceria (vide infra).
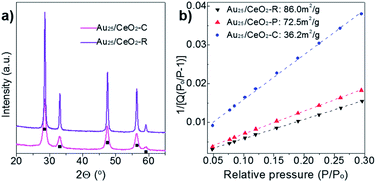 |
| Fig. 3 (a) XRD patterns and (b) specific surface areas of Au25/CeO2-R, Au25/CeO2-P and Au25/CeO2-C. | |
Catalytic performance in the CO oxidation
These prepared CeO2-supported Au cluster catalysts were evaluated in the CO oxidation in the presence of 1 v% CO and 20 v% O2, as shown in Fig. 4. Interestingly, the Au25/CeO2-C catalyst was inert from r.t. to 60 °C and started to give very low CO conversion at 80 °C (8.4%) and 120 °C (64%). In contrast, Au25/CeO2-P and Au25/CeO2-R showed a similar catalytic activity (Fig. 4a, black and red lines), which is much better than that of Au25/CeO2-C. Of note, both Au25/CeO2-P and Au25/CeO2-R show some activity (∼3.8% CO conversion) even at room temperature. Hence, the conversion rate is in the order of Au25/CeO2-R ≈ Au25/CeO2-P > Au25/CeO2-C, based on the CO conversion, which is different from the reported results over the naked Au NP system in the water–gas shift reactions (the rate follows the order of Au/CeO2-R > Au/CeO2-P > Au25/CeO2-C).32 It may be because the surface of Au25 clusters is capped by the thiolate ligands, except the interfacial perimeter sites. Of note, the plain CeO2 can only show activity at higher temperature (>200 °C), and its activity is negligible (almost zero conversion of CO) in the present temperature range of r.t. to 120 °C. Notably, Au25/CeO2-R showed good durability at 100 °C over 13 h (Fig. 4c). The rate of conversion converged to ∼95% with no appreciable loss of activity, implying that Au25/CeO2-R is robust and promising for prolonged periods of time. It should be noted that the Au25 nanoclusters supported on the ceria surface should be intact under such mild reaction conditions (≤120 °C).26
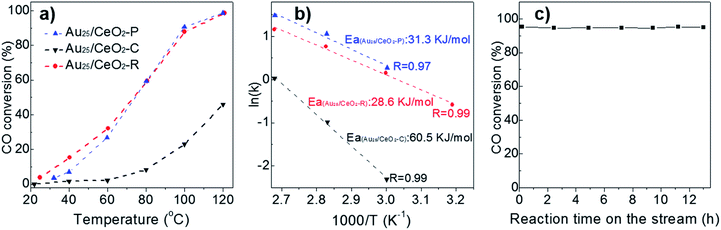 |
| Fig. 4 (a) Catalytic performance of Au25/CeO2 in the CO oxidation. (b) The apparent activation energies over the Au25/CeO2-R, Au25/CeO2-P and Au25/CeO2-C. (c) The durability of the Au25/CeO2-R catalyst at 100 °C for ∼13 h. Reaction conditions: 50 mg catalyst, reactant gas mixture consisting of 1 v% CO, 20 v% O2, and 79 v% N2 with a 15 000 mL g−1 h−1 GHSV. | |
Further, the apparent activation energies were calculated and determined to be 28.6, 31.3 and 60.5 kJ mol−1 over Au25/CeO2-R, Au25/CeO2-P and Au25/CeO2-C, respectively (Fig. 4b). The activation energy over Au25/CeO2-C is two fold that of Au25/CeO2-R and Au25/CeO2-P. It is well known that the CO and dioxygen molecules are activated over the gold particles and ceria surface respectively in the CO oxidation catalyzed by Au/CeO2. And in our previous studies, the CO oxidation over the ceria-supported gold clusters (capped with thiolates) occurred at the perimeter interface between Au particles and the supports, as the surface of gold clusters are well protected by the remaining organic ligands after thermal annealing. Therefore, the chemical state of gold atoms and the lattice facet of ceria play pivotal roles in the CO oxidation over the Au/CeO2 system.
XPS and CO-IR profiles
Therefore, the chemical states of the gold, cerium, and oxygen species were examined by XPS analysis. As shown in Fig. 5a, the binding energies (BEs) of Ce 3d are almost superimposable, indicating that the cerium species is Ce4+. With regard to the Au species, all the Au25/CeO2-R, Au25/CeO2-P and Au25/CeO2-C samples gave the BEs at 87.1 (Au 4f5/2) and 83.5 eV (Au 4f7/2) as shown in Fig. 5b, indicating that the chemical states of gold species were the same as Auδ+ (where, 0 < δ < 1).33 Moving to the oxygen species on the surface of ceria, two sets of BE peaks are found as shown in Fig. 5c, which are assigned to adsorbed oxygen species (OA, O 1s BE at 531.2 eV) and lattice oxygen (OL, 528.7 eV).34 After detailed calculations, the ratio of the OA species to OL is decreased as follows: Au25/CeO2-R (1.28) > Au25/CeO2-P (0.60) > Au25/CeO2-C (0.30). Therefore, both the BET and XPS analyses showed that Au25/CeO2-R and Au25/CeO2-P exhibited more hydroxyl species than the Au25/CeO2-C catalyst.
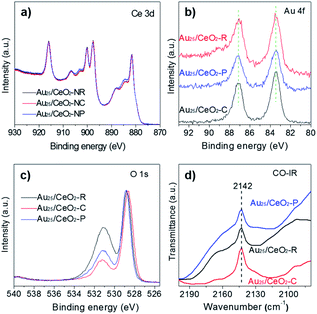 |
| Fig. 5 XPS analysis of the Au25/CeO2 samples: (a) Ce 3d, (b) Au 4f, and (c) O 1s. (d) Operando-DRIFTS of CO adsorption on Au25/CeO2 catalysts, which is investigated at −150 °C (liquid nitrogen). | |
Au25/CeO2 samples were investigated by in situ infrared CO adsorption experiments at a low temperature of −150 °C (liquid nitrogen), as shown in Fig. 5d. These samples were pretreated with He flow, and then they were introduced into a CO atmosphere for 5 min. The samples were finally treated with He flow to remove the excess CO. A set of intense bands at 2142 cm−1 was seen in all Au25/CeO2-R, Au25/CeO2-P and Au25/CeO2-C samples, which can be attributed to CO adsorbed on the Auδ+ species (0 < δ < 1), as the value is between the bands of CO–Au0 (∼2115 cm−1) and the CO–Au+ (∼2161 cm−1).35,36 These results matched well with the XPS analyses. Therefore, based on the results of the CO adsorption operando-DRIFTS characterization, gold active sites should be associated with the partially oxidized Auδ+ (0 < δ < 1) species.
Conclusions
In conclusion, well-defined CeO2 nanocubes (exposing CeO2(200) facet) and nanorods and nanopolyhedra (CeO2(002) and (111)) were adapted to anchor the atomically precise Au25(PET)18 clusters in this study. Au particles were not aggregated during the annealing at 120 °C to rule out the size-hierarchy of nanogold in the CO oxidation. The gold species in the Au25/CeO2 system are the same (Auδ+, 0 < δ < 1). It is found that the catalytic activity decreases in the order of Au25/CeO2-R ≈ Au25/CeO2-P > Au25/CeO4-C, and correspondingly the apparent activation energy of Au25/CeO2-C is two-fold that of the Au25/CeO2-R and Au25/CeO2-P catalysts. Au25/CeO2-R exhibited excellent durability in the CO oxidation over 13 h. To sum up, the catalytic activity can be drastically tailored by the adsorbed oxygen species on the facet of ceria, which can provide guidelines for the design of new oxide-supported nanogold catalysts for aerobic oxidations.
Conflicts of interest
There are no conflicts to declare.
Acknowledgements
We acknowledge the financial support by the National Natural Science Foundation of China (22065029 and 2217020878), Young Talents of Science and Technology in Universities of Inner Mongolia Autonomous Region (NJYT-20-B20), and Liaoning Natural Science Foundation of China (2020-MS-024).
Notes and references
- S. Tsubota, D. Cunningham, Y. Bando and M. Haruta, Stud. Surf. Sci. Catal., 1993, 77, 325–328 CrossRef CAS.
- Z. Li, C. Brouwer and C. He, Chem. Rev., 2008, 108, 3239–3265 CrossRef CAS PubMed.
- A. Corma and H. Garcia, Chem. Soc. Rev., 2008, 37, 2096–2126 RSC.
- G. Li and R. Jin, Nanotechnol. Rev., 2013, 5, 529–545 Search PubMed.
- G. Zhang, R. Wang and G. Li, Chin. Chem. Lett., 2018, 29, 687–693 CrossRef CAS.
- R. Zanella, S. Giorgio, C. R. Henry and C. Louis, J. Phys. Chem. B, 2002, 106, 7634–7642 CrossRef CAS.
- P. D. Vaidya and A. E. Rodrigues, Chem. Eng. J., 2006, 117, 39–49 CrossRef CAS.
- A. Taketoshi and M. Haruta, Chem. Lett., 2014, 43, 380–387 CrossRef CAS.
- D. Widmann and R. J. Behm, Acc. Chem. Res., 2014, 47, 740–749 CrossRef CAS PubMed.
- Y. Li and W. Shen, Chem. Soc. Rev., 2014, 43, 1543–1574 RSC.
- Q. Q. Shi, Y. Li, Y. Zhou, S. Miao, N. Ta, E. S. Zhan, J. Y. Liu and W. J. Shen, J. Mater. Chem. A, 2015, 3, 14409–14415 RSC.
- Q. Q. Shi, G. C. Ping, X. J. Wang, H. Xu, J. M. Li, J. Q. Cui, H. Abroshan, H. J. Ding and G. Li, J. Mater. Chem. A, 2019, 7, 2253–2260 RSC.
- F. Kollhoff, J. Schneider, G. Li, B. Sami, W. Shen, T. Berger, O. Diwald and J. Libuda, Phys. Chem. Chem. Phys., 2018, 20, 24858–24868 RSC.
- G. Yi, Z. Xu, G. Guo, K. Tanaka and Y. Yuan, Chem. Phys. Lett., 2009, 479, 128–132 CrossRef CAS.
- Z.-A. Qiao, Z. Wu and S. Dai, ChemSusChem, 2013, 6, 1821–1833 CrossRef CAS PubMed.
- C. Zhang, Y. Chen, H. Wang, Z. Li, K. Zheng, S. Li and G. Li, Nano Res., 2018, 11, 2139–2148 CrossRef CAS.
- N. Ta, J. Liu and W. Shen, Chin. J. Catal., 2013, 34, 838–850 CrossRef CAS.
- W. Li, C. Liu, H. Abroshan, Q. Ge, X. Yang, H. Xu and G. Li, J. Phys. Chem. C, 2016, 120, 10261–10267 CrossRef CAS.
- J. Zhang, Z. Li, J. Huang, C. Liu, F. Hong, K. Zheng and G. Li, Nanoscale, 2017, 9, 16879–16886 RSC.
- R. Jin, C. Zeng, M. Zhou and Y. Chen, Chem. Rev., 2016, 116, 10346–10413 CrossRef CAS PubMed.
- Q. Yao, T. Chen, X. Yuan and J. Xie, Acc. Chem. Res., 2018, 51, 1338–1348 CrossRef CAS PubMed.
- G. Li and R. Jin, Acc. Chem. Res., 2013, 46, 1749–1758 CrossRef CAS PubMed.
- C. Liu, C. Yan, J. Lin, C. Yu, J. Huang and G. Li, J. Mater. Chem. A, 2015, 3, 20167–20173 RSC.
- C. Liu, H. Abroshan, C. Yan, G. Li and M. Haruta, ACS Catal., 2016, 6, 92–99 CrossRef CAS.
- Z. Li, C. Liu, H. Abroshan, D. R. Kauffman and G. Li, ACS Catal., 2017, 7, 3368–3374 CrossRef CAS.
- Z. Li, W. Li, H. Abroshan, Q. Ge, Y. Zhou, C. Zhang, G. Li and R. Jin, Nanoscale, 2018, 10, 6558–6565 RSC.
- S. Yang and L. Gao, J. Am. Chem. Soc., 2006, 128, 9330–9331 CrossRef CAS PubMed.
- J. Li, N. Ta, Y. Li and W. Shen, Chin. J. Catal., 2008, 29, 823–830 CAS.
- H. X. Mai, L. D. Sun, Y. W. Zhang, R. Si, W. Feng, H. P. Zhang, H. C. Liu and C. H. Yan, J. Phys. Chem. B, 2005, 109, 24380–24385 CrossRef CAS PubMed.
- J. Lin, W. Li, C. Liu, P. Huang, M. Zhu, Q. Ge and G. Li, Nanoscale, 2015, 7, 13663–13670 RSC.
- G. Li and R. Jin, J. Am. Chem. Soc., 2014, 136, 11347–11354 CrossRef CAS PubMed.
- R. Si and M. Flytzani-Stephanopoulos, Angew. Chem., Int. Ed., 2008, 47, 2884–2887 CrossRef CAS PubMed.
- O. F. Odio, L. Lartundo-Rojas, P. Santiago-Jacinto, R. Martínez and E. Reguera, J. Phys. Chem. C, 2014, 118, 2776–2791 CrossRef CAS.
- N. Sutradhar, A. Sinhamahapatra and S. Pahari, J. Phys. Chem. C, 2011, 115, 7628–7637 CrossRef CAS.
- S. Wei, X. Fu, W. Wang, Z. Jin, Q. Song and C. Jia, J. Phys. Chem. C, 2018, 122, 4928–4936 CrossRef CAS.
- T. Venkov, K. Fajerwerg, L. Delannoy, H. Klimev, K. Hadjiivanov and C. Louis, Appl. Catal., A, 2006, 301, 106–114 CrossRef CAS.
Footnote |
† Z. L. and X. Z. contributed equally to this work. |
|
This journal is © The Royal Society of Chemistry 2021 |
Click here to see how this site uses Cookies. View our privacy policy here.