DOI:
10.1039/D0QO00456A
(Research Article)
Org. Chem. Front., 2021,
8, 25-31
A [15]paracyclophenone and its fluorenone-containing derivatives: syntheses and binding to nerve agent mimics via aryl-CH hydrogen bonding interactions†
Received
16th April 2020
, Accepted 8th May 2020
First published on 14th May 2020
Abstract
The syntheses and crystal structures of a [15]paracyclophenone and its two fluorenone-containing derivatives I and II are reported. The nerve agent mimics binding behaviors with 2 and I driven by the ‘non-traditional’ aryl-CH hydrogen bonding interactions are demonstrated in the solid state and supported by DFT calculations. The affinity of compound I towards the real nerve agents via this binding mode is also predicted by the computational method.
Introduction
Nerve agents are commonly organophosphorus compounds which inhibit acetylcholinesterase (AChE) in neuromuscular junctions causing the accumulation of acetylcholine, thus resulting in paralysis, convulsion and death.1 Supramolecular recognition of nerve agents based on non-covalent interactions for the removal2,3 and destruction4,5 of these toxic chemicals has attracted much attention in recent years. Many artificial receptors such as cyclodextrins,6 calixarenes,5 deep cavitands7,8 and Badjić's molecular baskets9–11 have been reported to be able to bind some of the nerve agent mimics. In these cases, the hydrophobic effect between the alkyl side-chains of the agents and the hosts is the major driving force.12,13 Other binding strategies are mainly focused on the polarized phosphoryl moiety, which can serve as a Lewis basic center by interacting with transition metals14–17 and hydrogen bond donors.18–23 However, most of the receptors based on hydrogen bonds were constructed utilizing amide or urea NH groups as building blocks. As a ‘non-traditional’ type of hydrogen bonding, aryl-CH hydrogen bonding has been studied in the field of anion recognition, predominantly pioneered by Flood,24–28 Haley and Johnson.29–33 Thus, the use of aryl CH hydrogen bonding interactions in the recognition of neutral guests, especially the highly toxic nerve agents, is of much interest.
[15]Paracyclophane 1 (Scheme 1) involving five phenyl rings bridged by five methylene groups is a charming macrocyclic molecule for its beautiful chemical structure and potential applications in supramolecular chemistry. Although the synthesis of 1 was reported by Gordon more than 30 years ago,34 few of its derivatives have been reported and its related host–guest chemistry has been rarely investigated due to its very complicated synthesis and very low yield (14 steps from commercial materials with the total yield of 0.4%). Fortunately, recently our group developed a new and highly-efficient method, which comprises only 4 steps from commercial p-dimethoxybenzene with a total yield up to 58% that can be run readily on a gram scale under mild conditions, making access to [15]paracyclophane 1 much easier.35 The subsequent π-metalation of [15]paracyclophane 1 granted this pentagon scaffold the capability of binding oxo-anions through anion–π interactions in solution and in the solid state.36 The design for the host molecules studied in this work was originally motivated by the aforementioned macrocyclic CH hydrogen bond donors together with the facile functionalization of 1 at the methylene bridges to generate a five-membered ketonic macrocycle, which was named [15]paracyclophenone 2 (Scheme 1). Oxidation of 1 at the bridge sites not only solves the regioselectivity problem associated with functionalization of the phenyl rings, but also make the resulting macrocycle 2 more electron deficient and adopt a relatively planar conformation. Another consideration is that the fast-rotating nature of the para-phenylene groups makes guest binding more entropically costly. Therefore, further functionalization of 2 was used to ‘lock’ the phenylene groups by forming fluorenone units and preorganizing the aromatic CH groups to achieve binding to guest molecules by aryl-CH hydrogen bonding interactions.
 |
| Scheme 1 Synthetic route to [15]paracyclophenone 2 from [15]paracyclophenone 1 and its fluorenone-containing derivatives I and II. | |
Based on these thoughts, herein, we show the syntheses of novel macrocycle 2 and its fluorenone-containing derivatives I and II, their crystal structures and host–guest binding of I to carbonyl compounds and organophosphorus nerve agent mimics driven by aryl-CH hydrogen bonding interactions. We also predict their binding affinities to different types of nerve agents based on density functional calculations. This is the first study on the recognition of nerve agents and their mimics by [15]paracyclophane derivatives.
Results and discussion
Syntheses and characterizations of [15]paracyclophenone 2 and its derivatives I and II
Direct access to 2 was readily provided by oxidation with chromium(VI) oxide in acetic anhydride (Scheme 1 and section 2 in the ESI†).37 The 1H NMR spectrum of 2 shown in Fig. 1a indicates the five-fold rotational symmetry of the product and the fast-rotation of its p-phenylene rings. In order to construct a preorganized structure, a palladium-catalyzed double C–H bond activation method was employed (Scheme 1 and section 3 in the ESI†).38,39 To our delight, derivatives bearing one or two fluorenone units were isolated from the reaction mixture, namely I (yield 36%) and II (yield 5%), whose 1H NMR spectra confirmed the formation of the fluorenone structure with the breaking of the original symmetry (Fig. 1, spectra b and c).
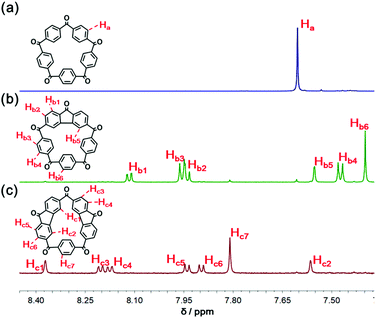 |
| Fig. 1 Partial 1H NMR spectra (600 MHz, CDCl3, 298 K): (a) 2; (b) I; (c) II. | |
Next, the solid-state structures of 2 and the derivatives I and II were determined unambiguously by the single-crystal X-ray diffraction. As shown in Fig. 2a, compared to 1, the structure of 2 is a relative planar pentagon with the average bond angle for the carbonyl bridge of 116.5°, which is less than 120° due to the ring strain of the macrocycle. In the case of compounds I and II, although the rotation of the fluorenone units is hindered by bonding, the rotation of the phenylene groups is not restricted, which is in consistent with the results of the 1H NMR experiments (Fig. 1, spectra b and c). It is worth noting that II self-assembled into a layer-by-layer structure driven by intermolecular π–π stacking interactions, forming a supramolecular framework (Fig. 2c).
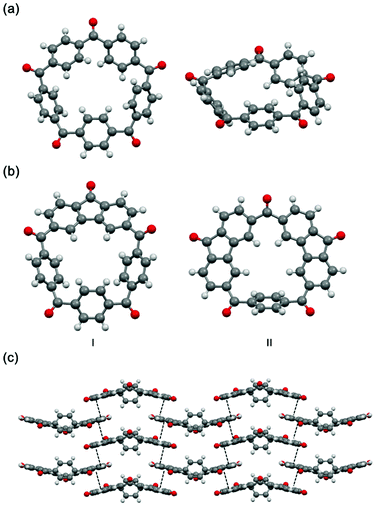 |
| Fig. 2 (a) Top and side views of X-ray crystal structure of 2. (b) X-ray crystal structures of I and II. (c) Packing structure of II. Solvent molecules are omitted for clarity. C grey, H white, O red. Dashed lines show the π–π stacking interactions. | |
Co-crystal structures of I with solvent molecules and carbonyl compounds
Interestingly, as a result of many attempts, during which different solvents were tested, to obtain single crystals of I suitable for the X-ray analysis, a series of co-crystal structures of I with solvent molecules were obtained (Fig. 3). In both [I·acetone] and [II·acetone] (Fig. 3a) one acetone molecule is situated in the center of the host molecule with the electron-rich oxygen atom bonded to four aryl CH hydrogens forming a 1
:
1 host–guest complex. The lengths of d (C–H⋯O) range from 2.42 Å to 2.67 Å, which are shorter than the sum of the van der Waals radius of the interacting atoms.40 The crystal structures of [I·ethyl acetate] and [I·dimethyl sulfoxide] also show similar binding modes (Fig. 3b and c). However, in the case of [I·acetonitrile], an acetonitrile molecule is interacting with Ivia C–H⋯N and C–H⋯π-C
N hydrogen bonding interactions (Fig. 3d). To further investigate the ability of I to bind different types of carbonyl compounds, several carbonyl-containing guests with relatively high melting points were introduced to construct 1
:
1 complexes with I in the solid state. Therefore, another three co-crystal structures were provided by slow evaporation of a chloroform solution of I and excess guest (Fig. 4). In each of these structures, the guest molecule was seated in the center cavity of I, interacting with the host through aryl CH hydrogen bonding interactions.
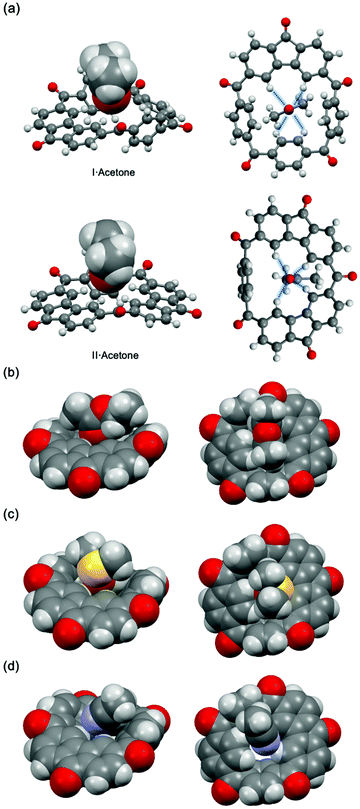 |
| Fig. 3 X-ray crystal structures (space-filling diagrams): (a) [I·acetone] and [II·acetone]; (b) [I·ethyl acetate]; (c) [I·dimethyl sulfoxide]; (d) [I·acetonitrile]. C grey, H white, O red, N blue, S yellow. Dashed lines show the aryl CH hydrogen bonding interactions. | |
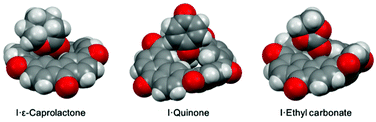 |
| Fig. 4 Single crystal structures (space-filling diagrams) of complexes [I·ε-caprolactone], [I·quinone] and [I·ethyl carbonate]. C grey, H white, O red, N blue, P deep yellow, Cl green. | |
Furthermore, DFT calculations were carried out to further confirm this binding mode driven by the aryl CH hydrogen bonds (Fig. 5). In DFT calculated energy-minimized structures of the complexes [I·acetone], [I·ethyl acetate] and [I·dimethyl sulfoxide] the guest molecules were found to bind to I through four C–H⋯O interactions with distances ranging from 2.29 Å to 2.59 Å. While in the computed structure of [I·ethyl carbonate], only two C–H⋯O interactions were found, with distances of 2.34 Å and 2.51 Å. This is consistent with what was found in the single crystal structure of [I·ethyl carbonate]. In short, these experiments not only support the existence of the aryl CH hydrogen bonding interactions between I and neutral guests in the solid state, but also indicate the adaptive nature of the host molecule.
 |
| Fig. 5 DFT calculated structures of complexes [I·acetone], [I·ethyl acetate], [I·ethyl carbonate] and [I·dimethyl sulfoxide]. C grey, H white, O red, S yellow. Trivial hydrogens are omitted for clarity. | |
Binding to nerve agent mimics
Enlightened by the aforementioned results, we wondered whether I could bind nerve agent mimics with the more Lewis basic phosphoryl group. Despite the chemical shift changes observed in the 1H NMR titrations of [15]paracyclophenone 2 or I with dimethyl methylphosphate (DMMP), dimethyl 4-nitrophenylphosphate (DMNP), dimethyl chlorophosphate (DMCP) and dimethyl fluorophosphates (DMFP) in d-chloroform, the binding constants with these nerve agent mimics are very low (Ka < 10 M−1) probably due to the strong competitive effect of the solvent molecules (section 4 in the ESI†). Fortunately, co-crystal structures obtained by evaporation of the host–guest solutions confirmed the formation of 1
:
1 complexes between I and the nerve agent mimics in the solid state (Fig. 6). In each case as expected, the host molecule serves as the CH hydrogen bond donor, binding to the phosphoryl group of the guest molecule through multiple aryl-CH hydrogen bonding interactions. In the crystal structure of [2·DMFP] the guest molecule was bound to 2 similarly, indicating the ability of 2 to bind nerve agent mimics in the solid state. However, during the 1H NMR titrations of II with nerve agent mimics, no chemical shift was observed even when the guest/host ratio was over 100 (section 4 in the ESI†). Crystallization of II with nerve agent mimics only resulted in single crystals with no guest molecule in the lattice. The most probable reason for this phenomenon may be the strong intermolecular π–π stacking interactions between the II molecules, which prevent the host from binding to the guest even though the rotation of the two fluorenone units of II is highly constrained making the guest binding less entropically costly.
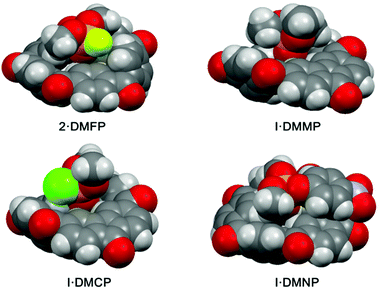 |
| Fig. 6 Single crystal structures of complexes [2·DMFP], [I·DMMP], [I·DMCP] and [I·DMNP]. C grey, H white, O red, N blue, P deep yellow, Cl green, F light green. | |
In order to gain more insight into the binding mode driven by aryl CH hydrogen bonding interactions and the binding affinity to nerve agent mimics, density functional theory (DFT) calculations were performed at the level of B3LYP-D3(BJ)/def2-TZVP in the gas phase. Firstly, the gas phase energy-minimized structures of the host–guest complexes were calculated. As shown in Fig. 6, in each case the oxygen atom of the P
O group is interacting with the aryl CH hydrogens of I through four aryl CH hydrogen bonding interactions. These computational binding modes are consistent with the experimental observations in the solid state. The free energy changes and enthalpy changes of the formation of I-based host–guest complexes with various guests were calculated (section 6 in the ESI†). These results suggested that I binds phosphoryl nerve agent mimics more strongly than the carbonyl compounds as expected (Table 1).
Table 1 DFT-computed free energy changes (ΔG) and enthalpy changes (ΔH) in the gas phase of complexes of host I with various guests
Guest name |
Guest structure |
ΔH (kcal mol−1) |
ΔG (kcal mol−1) |
Acetone |
|
−10.2 |
−0.1 |
Ethyl acetate |
|
−11.6 |
−0.7 |
Ethylene carbonate |
|
−12.2 |
−1.2 |
Dimethyl sulfoxide |
|
−13.4 |
−3.7 |
DMMP |
|
−17.7 |
−6.0 |
DMCP |
|
−15.5 |
−4.5 |
DMFP |
|
−15.7 |
−4.3 |
DMNP |
|
−21.4 |
−8.1 |
On the basis of the observation that 2 binds nerve agent mimic in the solid state, structures of the complexes [2·DMMP], [2·DMNP], [2·DMCP] and [2·DMFP], as well as their free energy and enthalpy changes were also calculated by the DFT calculations. According to the data shown in Table 2, 2 shows slightly weaker affinities for the nerve agent mimics compared with I. There are two possible reasons for this. First, the aforementioned fast rotating nature of the p-phenylene group of 2 makes guest binding more entropically costly. Second, the central cavity size of I is smaller than that of 2, which makes a more suitable binding geometry for the phosphoryl group. What's more, this was further supported by the calculated 2 based host–guest complex structures (Fig. 8). For example, in [2·DMCP] the oxygen atom of the P
O group interacts with two aryl CH hydrogens of 2 with distances of 2.52 Å and 2.60 Å, while the oxygen atom of the –OCH3 group interacts with another aryl CH hydrogen. By contrast, in the case of calculated [I·DMCP] (Fig. 7), the phosphoryl group interacts with four aryl CH hydrogens of I with C–H⋯O distances ranging from 2.26 Å to 2.61 Å, suggesting that I forms more and tighter aryl CH hydrogen bonding interactions with the phosphoryl guest than 2. In short, the binding geometry of I is more suitable than that of 2 to adapt to the nerve agent mimic guest.
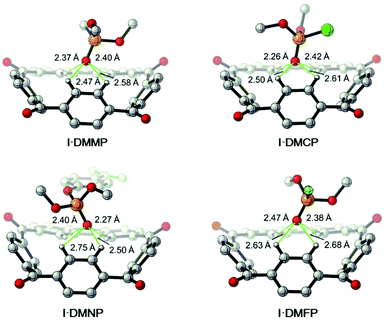 |
| Fig. 7 DFT calculated structures of the complexes [I·DMMP], [I·DMCP], [I·DMNP] and [I·DMFP]. C grey, H white, O red, P deep yellow, Cl green, F light green. Trivial hydrogens are omitted for clarity. | |
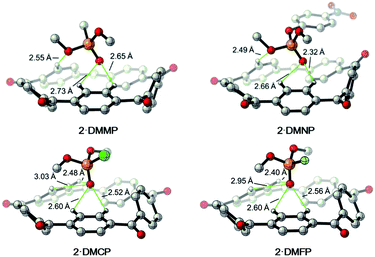 |
| Fig. 8 DFT calculated structures of the complexes [2·DMMP], [2·DMNP], [2·DMCP] and [2·DMFP]. C grey, H white, O red, P deep yellow, Cl green, F light green. Trivial hydrogens are omitted for clarity. | |
Table 2 DFT-computed free energy changes (ΔG) and enthalpy changes (ΔH) in the gas phase of complexes of host 2 with various guests
Guest name |
Guest structure |
ΔH (kcal mol−1) |
ΔG (kcal mol−1) |
DMMP |
|
−14.5 |
−2.2 |
DMCP |
|
−16.4 |
−4.4 |
DMFP |
|
−14.4 |
−2.6 |
DMNP |
|
−17.9 |
−3.5 |
Computational studies on prediction of binding of nerve agents
Although the binding mode and affinity of I towards nerve agent mimics are supported by experimental and theoretical results, it still remains unclear whether this binding ability based on the multiple aryl CH hydrogen bonding interactions is suitable for real nerve agents such as GA (tabun), GD (soman), VX and VG. To our delight, subsequent DFT calculations suggested a relatively strong affinity for these nerve agents with the binding free energies ranging from −2.9 to −6.6 kcal mol−1 (Fig. 9b). What's more, the proposed binding mode through multiple aryl-CH hydrogen bonding interactions was also supported by the IGM analysis (Fig. 9c).41
 |
| Fig. 9 (a) Chemical structures of nerve agents GA, VX, GD and VG. (b) DFT-computed free energy and enthalpy changes of complexes [I·GA], [I·GD], [I·VX] and [I·VG]. Trivial hydrogens are omitted for clarity. (c) Independent Gradient Model (IGM) analysis of host–guest complexes [I·GD] and [I·VG]. Isovalue = 0.005. | |
Conclusions
In conclusion, we designed and synthesized the novel pentagon marcocycle [15]paracyclophenone 2 and its fluorenone-containing derivatives I and II. It was demonstrated that 2 and I bind nerve agent mimics in the solid state through multiple aryl CH hydrogen bonding interactions. The DFT calculations not only confirmed the binding mode, but also predicted the affinities towards the real nerve agents. Therefore, for the first time, here we investigated molecular recognition by [15]paracyclophane derivatives for nerve agents and their mimics. These results will shed light not only on the rarely studied host–guest chemistry of [15]paracyclophane derivatives, but also on the development of new artifical macrocycles, especially metal-free hosts, for the recognition and supramolecualr destruction of nerve agents. Further studies are ongoing and the results will be reported in due course.
Conflicts of interest
There are no conflicts to declare.
Acknowledgements
We are grateful for the financial support from the National Science Foundation of China (21434005, 91527310, F. H.; 21702182, 21873081, X. H.), China Postdoctoral Science Foundation (2019M652056, H. W.), Fundamental Research Funds for the Central Universities (2019QNA3009, H. X.) and Zhejiang University. Calculations were performed on the high-performance computing system at the Department of Chemistry, Zhejiang University.
References
- Y. Jang, K. Kim, O. Tsay, D. Atwood and D. Churchill, Update 1 of: Destruction and Detection of Chemical Warfare Agents, Chem. Rev., 2015, 115, PR1–PR76 CrossRef PubMed.
- S. Border, R. Pavlovic, Z. Lei and J. Badjic, Removal of Nerve Agent Simulants from Water Using Light-Responsive Molecular Baskets, J. Am. Chem. Soc., 2017, 139, 18496–18499 CrossRef CAS PubMed.
- S. Border, R. Pavlovic, Z. Lei, M. Gunther, H. Wang, H. Cui and J. Badjic, Photo-Induced Formation of Organic Nanoparticles Possessing Enhanced Affinities for Complexing Nerve Agent Mimics, Chem. Commun., 2019, 55, 1987–1990 RSC.
- M. Zengerle, F. Brandhuber, C. Schneider, F. Worek, G. Reiter and S. Kubik, Highly Efficient Cyclosarin Degradation Mediated by a β-Cyclodextrin Derivative Containing an Oxime-derived Substituent, Beilstein J. Org. Chem., 2011, 7, 1543–1554 CrossRef CAS PubMed.
- C. Schneider, A. Biewisch, M. Koller, F. Worek and S. Kubik, Detoxification of VX and Other V-Type Nerve Agents in Water at 37 °C and pH 7.4 by Substituted Sulfonatocalix[4]arenes, Angew. Chem., Int. Ed., 2016, 55, 12668–12672 (
Angew. Chem.
, 2016
, 128
, 12859–12863
) CrossRef CAS PubMed.
- S. Letort, S. Balieu, W. Erb, G. Gouhier and F. Estour, Interactions of Cyclodextrins and Their Derivatives with Toxic Organophosphorus Compounds, Beilstein J. Org. Chem., 2016, 12, 204–228 CrossRef CAS PubMed.
- S. Daly, M. Grassi, D. Shenoy, F. Ugozzoli and E. Dalcanale, Supramolecular Surface Plasmon Resonance (SPR) Sensors for Organophosphorus Vapor Detection, J. Mater. Chem., 2007, 17, 1809–1811 RSC.
- C. Tudisco, P. Betti, A. Motta, R. Pinalli, L. Bombaci, E. Dalcanale and G. Condorelli, Cavitand-Functionalised Porous Silicon as Active Surface for Organophosphorus Vapors Detection, Langmuir, 2012, 28, 1782–1789 CrossRef CAS PubMed.
- S. Chen, Y. Ruan, J. Brown, J. Gallucci, V. Maslak, C. Hadad and J. Badjic, Assembly of Amphiphilic Baskets into Stimuli-Responsive Vesicles. Developing a Strategy for the Detection of Organophosphorus Chemical Nerve Agents, J. Am. Chem. Soc., 2013, 135, 14964–14967 CrossRef CAS PubMed.
- S. Chen, Y. Ruan, J. Brown, C. Hadad and J. Badjic, Recognition Characteristics of an Adaptive Vesicular Assembly of Amphiphilic Baskets for Selective Detection and Mitigation of Toxic Nerve Agents, J. Am. Chem. Soc., 2014, 136, 17337–17342 CrossRef CAS PubMed.
- Y. Duan, E. Dalkilic, P. Peterson, A. Pandit, A. Dastan, J. Brown, S. Polen, C. Hadad and J. Badjic, Trapping of Organophosphorus Chemical Nerve Agents in Water with Amino Acid Functionalized Baskets, Chem. – Eur. J., 2014, 20, 4251–4256 CrossRef PubMed.
- M. Sambrook and S. Notman, Supramolecular chemistry and chemical warfare agents: from fundamentals of recognition to catalysis and sensing, Chem. Soc. Rev., 2013, 42, 9251–9267 RSC.
- W. Liu, Z. Chen, L. Yang, H. Au-Yeung and W. Jiang, Molecular Recognition of Organophosphorus Compounds in Water and Inhibition of Their Toxicity to Acetylcholinesterase, Chem. Commun., 2019, 55, 9797–9800 RSC.
- I. Bandyopadhyay, M. Kim, Y. Lee and D. Churchill, Favorable Pendant-Amino Metal Chelation in VX Nerve Agent Model Systems, J. Phys. Chem. A, 2006, 110, 3655–3661 CrossRef CAS PubMed.
- K. Kim, I. Kim, I. Maiti, S. Kwon, D. Bucella, O. Egorova, Y. Lee, J. Kwak and D. Churchill, A Study of Nerve Agent Model Organophosphonate Binding with Manganese-A2B-Corrole and -A2B2-Porphyrin Systems, Polyhedron, 2009, 28, 2418–2430 CrossRef CAS.
- G. Dennison, M. Sambrook and M. Johnston, VX and VG Chemical Warfare Agents Bidentate Complexation with Lanthanide Ions, Chem. Commun., 2014, 50, 195–197 RSC.
- R. Puglisi, A. Pappalardo, A. Gulino and G. Sfrazzetto, Supramolecular Recognition of a CWA Simulant by Metal-Salen Complexes: The First Multi-Topic Approach, Chem. Commun., 2018, 54, 11156–11159 RSC.
- M. Sambrook, J. Hiscock, A. Cook, A. Green, I. Holden, J. Vincent and P. Gale, Hydrogen Bond-Mediated Recognition of the Chemical Warfare Agent Soman (GD), Chem. Commun., 2012, 48, 5605–5607 RSC.
- A. Barba-Bon, A. Costero, M. Parra, S. Gil, R. Matines-Manez, F. Sancenon, P. Gale and J. Hiscock, Neutral 1,3-Diindolylureas for Nerve Agent Remediation, Chem. – Eur. J., 2013, 19, 1586–1590 CrossRef CAS PubMed.
- J. Hiscock, N. Wells, J. Ede, P. Gale and M. Sambrook, Biasing Hydrogen Bond Donating Host Guest Systems Towards Chemical Warfare Agent Recognition, Org. Biomol. Chem., 2016, 14, 9560–9567 RSC.
- S. Ha, M. Lee, H. Seo, S. Song, K. Kim, C. Park, H. Kim, Y. Kim and C. Song, Structural Effect of Thioureas on the Detection of Chemical Warfare Agent Simulants, ACS Sens., 2017, 2, 1146–1151 CrossRef CAS PubMed.
- Y. Chung, S. Ha, T. Woo, Y. Kim, C. Song and S. Kim, Binding Thiourea Derivatives with Dimethyl Methylphosphonate for Sensing Nerve Agents, RSC Adv., 2019, 9, 10693–10701 RSC.
- H. Cave, J. Ede, M. Sambrook, H. Dodd, F. Fucassi, A. Cragg, A. Lansley and P. Cragg, Hydrogen-Bonding Interactions in Crown-(thio)urea Complexes with Anions, Chemical Warfare Agents and Simulants, Supramol. Chem., 2019, 31, 703–712 CrossRef CAS.
- Y. Li and A. Flood, Pure C-H Hydrogen Bonding to Chloride Ions: A Preorganized and Rigid Macrocycle Receptor, Angew. Chem., Int. Ed., 2008, 47, 2649–2652 (
Angew. Chem.
, 2008
, 120
, 2689–2652
) CrossRef CAS PubMed.
- R. Ramabhadran, Y. Hua, Y. Li, A. Flood and K. Raghavachari, From Atomic to Molecular Anion: A Neutral Receptor Captures Cyanide Using Strong C-H Hydrogen Bonds, Chem. – Eur. J., 2011, 17, 9123–9129 CrossRef CAS PubMed.
- S. Lee, C. Chen and A. Flood, A Pentagonal Cyanostar Macrocycle with Cyanostilbene CH Donors Binds Anions and Forms Dialkylphosphate [3]rotaxanes, Nat. Chem., 2013, 5, 704–710 CrossRef CAS PubMed.
- E. Fatila, E. Twum, A. Sengupta, M. Pink, J. Karty, K. Raghavachari and A. Flood, Anions Stabilize Each Other inside Macrocyclic Hosts, Angew. Chem., Int. Ed., 2016, 55, 14057–14062 (
Angew. Chem.
, 2016
, 128
, 14263–14268
) CrossRef CAS PubMed.
- B. Qiao, G. Leverick, W. Zhao, A. Flood, J. Johnson and S. Yang, Supramolecular Regulation of Anions Enhances Conductivity and Transference Number of Lithium in Liquid Electrolytes, J. Am. Chem. Soc., 2018, 140, 10932–10936 CrossRef CAS PubMed.
- O. Berryman, A. Sather, B. Hay, J. Meisner and D. Johnson, Solution Phase Measurement of Both Weak σ and C-H⋯X− Hydrogen Bonding Interactions in Synthetic Anion Receptors, J. Am. Chem. Soc., 2008, 130, 10895–11089 CrossRef CAS PubMed.
- M. Hartle, R. Hansen, B. Tresca, S. Prakel, L. Zakharov, M. Haley, M. Pluth and D. Johnson, A Synthetic Supramolecular Receptor for the Hydrosulfide Anion, Angew. Chem., Int. Ed., 2016, 55, 11480–11484 (
Angew. Chem.
, 2016
, 128
, 11652–11656
) CrossRef CAS PubMed.
- H. Fargher, N. Lau, L. Zakharov, M. Haley, D. Johnson and M. Pluth, Expanding Reversible Chalcogenide Binding: Supramolecular Receptors for the Hydroselenide (HS−) Anion, Chem. Sci., 2019, 10, 67–72 RSC.
- C. Deng, J. Bard, J. Lohrman, J. Barker, L. Zakharov, D. Johnson and M. Haley, Exploiting the Hydrogen Bond Donor/Acceptor Properties of PN-Heterocycles: Selective Anion Receptors for Hydrogen Sulfate, Angew. Chem., Int. Ed., 2019, 58, 3934–3938 (
Angew. Chem.
, 2018
, 131
, 3974–3978
) CrossRef CAS PubMed.
- L. Eytel, H. Fargher, M. Haley and D. Johnson, The Road to Aryl CH⋯Anion Binding Was Paved with Good Intentions: Fundamental Studies, Host Design, and Historial Perspectives in CH Hydrogen Bonding, Chem. Commun., 2019, 55, 5195–5206 RSC.
- G. Gribble and C. Nutaitis, [1.1.1.1.1.]Paracyclophane and [1.1.1.1.1.1]Paracyclophane, Tetrahedron Lett., 1985, 26, 6023–6026 CrossRef CAS.
- P. Liu, Q. Li, H. Zeng, B. Shi, J. Liu and F. Huang, [15]Paracyclophane and [16]Paracyclophane: Facile Syntheses, Cystal Structures and Selective Complexation with Cesium Cations in the Gas Phase, Org. Chem. Front., 2019, 6, 309–312 RSC.
- H. Zeng, P. Liu, G. Feng and F. Huang, π-Metalated [15]Paracyclophane: Synthesis and Binding to Oxo-Anions via Anion-π Interactions, J. Am. Chem. Soc., 2019, 141, 16501–16511 CrossRef PubMed.
- N. Seri, I. Thondorf and S. Biali, Preparation and Conformation of Dioxocalix[4]arene Derivatives, J. Org. Chem., 2004, 69, 4774–4780 CrossRef CAS PubMed.
- P. Gandeepan, C. Hung and C. Cheng, Pd-catalyzed Double C–H Activation of Diaryl Ketones for the Synthesis of Fluorenones, Chem. Commun., 2012, 48, 9379–9381 RSC.
- H. Li, R. Zhu, W. Shi, K. He and Z. Shi, Synthesis of Fluorenone Derivatives through Pd-Catalyzed Dehydrogenative Cyclization, Org. Lett., 2012, 14, 4850–4853 CrossRef CAS PubMed.
- A. Bondi, van der Waals Volumes and Radii, J. Phys. Chem., 1964, 68, 441–451 CrossRef CAS.
- C. Lefebvre, G. Rubez, H. Khartabil, J. Boisson, J. Contreras-Garcia and E. Henon, Accurately Extracting the Signature of Intermolecular Interactions Present in the NCl Plot of the Reduced Density Gradient versus Electron Density, Phys. Chem. Chem. Phys., 2017, 19, 17928–17936 RSC.
Footnotes |
† Electronic supplementary information (ESI) available: Synthetic procedures, characterizations and X-ray crystallographic files (CIF), DFT calculation details and other materials. CCDC 1980663–1980675 and 1987856. For ESI and crystallographic data in CIF or other electronic format see DOI: 10.1039/d0qo00456a |
‡ These authors contributed equally to this work. |
|
This journal is © the Partner Organisations 2021 |
Click here to see how this site uses Cookies. View our privacy policy here.