DOI:
10.1039/D0RA08395J
(Paper)
RSC Adv., 2021,
11, 3636-3644
Roles of hydroxyl and carbonate radicals in bisphenol a degradation via a nanoscale zero-valent iron/percarbonate system: influencing factors and mechanisms†
Received
1st October 2020
, Accepted 19th December 2020
First published on 18th January 2021
Abstract
In this work, nanoscale-zero-valent iron (nZVI) was applied to activate sodium percarbonate (SPC) to eliminate bisphenol A (BPA), which poses a risk to ecological and human health as a typical endocrine disruptor. The influence of nZVI loading, SPC dosing, initial pH, and the presence of inorganic anions (including Cl−, HPO42−, NO3− and NO2−) and humic acid on BPA removal by the nZVI/SPC system were investigated. Based on the scavenger test results, ˙OH and CO3˙− participated in the degradation of BPA, and ˙OH was illustrated to be the dominant radical. The X-ray diffraction (XRD) and X-ray photoelectron spectroscopy (XPS) analysis suggested that surface iron oxide generation, electron transfer and Fe2+ release were the main processes of the SPC activation by nZVI. Moreover, BPA transformation products were detected by LC-MS allowing the proposal of a possible degradation pathway of BPA. Along with the degradation of the parent compound BPA, the total organic carbon (TOC) gradually decreased, while the bio-toxicity increased at the initial stage of the reaction (0–3 min) and then decreased to a lower level rapidly at 20 min. Overall, this study evidenced the feasibility of the nZVI/SPC system to efficiently degrade BPA, broadening the applications of nZVI in wastewater treatment.
1. Introduction
In recent decades, bisphenol A (BPA), as a precursor in the manufacture of polycarbonate, epoxy resin and polystyrene,1 has resulted in the ubiquitous presence of BPA in mineral water bottles, medical equipment and food packaging. Due to the improper disposal and clearance of this BPA-containing waste, BPA is directly or indirectly released into various water bodies.2,3 It was found that the concentration of BPA in drinking water, surface water and waste landfill leachate could reach 0.1 μg L−1, dozens of μg L−1 and 17.2 mg L−1, respectively.2,4 As a typical endocrine disruptor,5 BPA poses a risk to aquatic wildlife and human beings even at low concentrations.6 However, BPA could not be effectively degraded from wastewater by the conventional strategies including coagulation, filtration, adsorption and biodegradation.7,8 Therefore, it is urgent to develop an efficient method to abate BPA from water and to simultaneously decrease the bio-toxicity of BPA.
Currently, hydroxyl radical (˙OH)-based advanced oxidation processes (AOPs) have been considered as a powerful technology for BPA remediation,9–11 which is based on the activation of liquid H2O2 to generate ˙OH. In the traditional Fenton system, H2O2 could be catalyzed by Fe2+ to form ˙OH for organic contaminants elimination.12 But, H2O2 was unstable and easily decomposed, making it difficult to transportation and storage.13,14 Sodium percarbonate (SPC), as the solid phase carrier of H2O2, is a promising alternative to liquid H2O2 due to the low cost, high stability, and effective activation.13,15 In addition, the by-products of SPC decomposition contain Na2CO3, which can react with ˙OH to form carbonate radical (CO3−) and accelerate the degradation of contaminants with electron-donating moieties (eqn (1)–(3)).16,17 Based on these advantages, increasing attentions have been paid to the homogeneous activation of SPC by Fe2+/Fe3+, which has great power in the remediation of organic contaminants in water. Miao et al.18 demonstrated that perchloroethylene was efficiently oxidized by the Fe2+/SPC system in groundwater, and ˙OH was the main active species, showing better performance than the traditional Fenton process at the similar reaction conditions (eqn (4)–(6)). Nevertheless, there are unavoidable drawbacks such as narrow reaction pH rang, non-recycle of catalyst and precipitation of ferric hydroxide (Fe(OH)3), which results in large amount of iron sludge.19 In order to overcome these limitations, nanoscale zero-valent iron (nZVI), as an non-toxic, and easy recycled catalyst with relatively large specific surface area, shows excellent potential in activating peroxide oxidants, such as persulfate, peroxymonosulfate, bisulfite and hydrogen peroxide, for removing organic contaminants.20–24 In the process of nZVI/hydroperoxide, organic pollutants are mainly destroyed by the generated ˙OH and purified by the Fe0 corrosion products (iron oxides).25 However, there have not been any comprehensive mechanistic studies on the activation of SPC by nZVI for the removal of contaminants of emerging concern.
|
Na2CO3·1.5 H2O2 → Na2CO3 + 1.5 H2O2
| (1) |
|
˙OH + CO32− → CO3˙− + OH−
| (2) |
|
˙OH + HCO3− → CO3˙− + H2O
| (3) |
|
Fe0 + O2 + 2H+ → Fe2+ + H2O2
| (4) |
|
Fe0 + H2O2 + 2H+ → Fe2+ + 2H2O
| (5) |
|
Fe2+ + H2O2 → ˙OH + Fe3+ + OH−
| (6) |
Herein, in the present study, it is aimed to investigate the insight of the mechanisms of SPC activation by nZVI for BPA degradation in wastewater. The operation parameters of the nZVI/SPC system were optimized, such as nZVI dosage, SPC concentration, and initial pH. The influences of the nature water constituents on the BPA degradation were carefully evaluated, including the Cl−, HPO42−, NO2−, NO3− anions, and humic acid (HA). The changes of the chemical compounds on the surface of the pristine and used nZVI were characterized to propose the possible mechanisms for SPC activation. Finally, the transformation products (TPs) were detected to assist the bio-toxicity analysis during the BPA degradation, providing the fundamental knowledge and theoretic support for the future applications of nZVI/SPC method in the wastewater treatment.
2. Materials and methods
2.1 Chemicals
Bisphenol A (BPA, >99.8%), isopropyl alcohol (IPA, ACS, ≥ 99.5%) and phenol (PhOH, ACS) were supplied by Aladdin Chemistry. Sodium percarbonate (Na2CO3·1.5 H2O2, SPC, 98%) was purchased from Sigma-Aldrich. Other analytical grade chemicals provided by Sinopharm Chemical Reagent. No further purification was employed for all reagents. The ultrapure water (18.2 Ω cm) was conducted to prepare reagents.
2.2 Preparation of the nZVI catalyst
The nZVI particle was synthesized by the liquid phase reduction method.26 Detailed process was described in ESI.†
2.3 Catalytic degradation experiments
In this study, unless otherwise noted, all batch experiments were performed at room temperature (25 ± 2 °C) in 250 mL beaker. The initial concentration of BPA was 0.1 mM and the total volume was 100 mL. Following the addition of a desired amount of SPC, the initial pH of mixture was adjusted with 0.1 M H2SO4 as quickly as possible. BPA degradation was initiated by adding certain dosages of nZVI under continuously mechanical agitation. 1.0 mL sample was collected and immediately mixed with 1.0 mL of methanol to quench the residual reactive species at predetermined time intervals. Afterwards, the samples were filtered through 0.22 μm PVDF membranes.
To evaluate the reusability of catalyst, the used nZVI particles were collected by magnetic separation and washed with ultrapure water. Then, the recycled catalysts were utilized for the next reaction under the identified conditions. All the experiments were conducted in triplicate to ensure the accuracy of data.
2.4 Analytical and characterization method
The concentration of BPA was measured using high performance liquid chromatography (HPLC, RIGOL L-3000, China), equipped with a Compass C18 column (5 μm, 4.6 × 250 mm) and UV detector (wavelength at 280 nm for BPA). The mobile phase was consist of acetonitrile and ultrapure water (40
:
60, v/v) with a constant flow rate of 1.0 mL min−1. The injection volume was 20 μL and column temperature was set at 35 °C.
The concentrations of Fe2+ ions were colorimetrically measured using the standard method.27 Briefly, o-phenanthroline was added into the filtered reaction solution and the resulting solution was measured at 510 nm wavelength on a UV-vis light spectrophotometer. The contained Fe3+ ions were reduced to Fe2+ ions by oxammonium hydrochloride to measure the concentrations of total iron ions in the reaction solutions. Total Organic Carbon (TOC) was determined with a TOC/TN analyzer (multi N/C 2100, Jena, German) to assess the mineralization rate of BPA. The transformation products of BPA were determined by an ultrahigh performance Liquid Chromatography (UPLC, UltiMate 3000, Dionex) coupled with a mass spectrometer (MS, Q-Orbitrap, Thermo Scientific). The UPLC-MS was performed with negative electrospray ionization (ESI−) in a full-scan range of 50–350 m/z at 40 °C. The injection volume was 1 μL. The biological toxicity during the BPA degradation process was evaluated by the activated sludge inhibition test (ASIT) as previously reported.28
The structure and crystal phase of nZVI before and after use were analyzed by X-ray diffraction (XRD, D8 Advance, Bruker, Germany) using Cu Kα radiation. The chemical states of Fe and O were analyzed by X-ray photoelectron spectroscopy using monochromatic 100 eV Al-Kα radiation (XPS, ESCALAB 250Xi, Thermo Fisher).
2.5 Kinetics analysis
Based on the BPA removal pattern, the BPA degradation kinetics in nZVI/SPC system should follow a pseudo-first-order reaction, as shown in eqn (7).Where Ct is the concentration of BPA (mM) at reaction time t (min) and C0 is the initial BPA concentration (mM), respectively. kobs represents the observed rate constant (min−1).
3. Results and discussion
3.1 Degradation kinetics of BPA by nZVI/SPC
In this work, various reaction processes were performed to assess the BPA degradation efficiency, including nZVI, SPC, nZVI/SPC, nZVI/H2O2 and Fe2+/SPC systems. As shown in Fig. 1, BPA was slightly removed in the presence of nZVI or SPC alone within 20 min. The results indicated that nZVI was ineffective to adsorb molecular BPA (pka = 9.6–10.2).29 Moreover, SPC has limited oxidation ability to remove BPA without activation. When nZVI combined with SPC, BPA was completely removed within 20 min, suggesting that nZVI has an excellent performance on the SPC activation. However, BPA degradation was deceased in the reaction system of nZVI/H2O2. Because carbonates from SPC could transform to be CO3˙− through eqn (2) and (3), which favor to oxidize the electron-rich organic pollutants,13,16,30 resulting in the improvement of BPA degradation efficiency in the nZVI/SPC system.
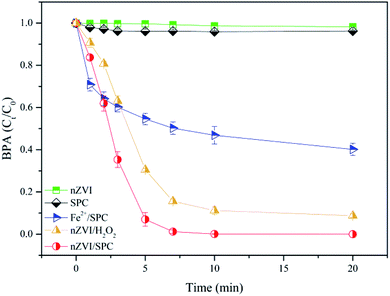 |
| Fig. 1 BPA degradation in various systems. General reaction conditions: [BPA]0 = 0.1 mM, [nZVI]0 = 0.1 g L−1, [Fe2+]0 = 2.3 mg L−1, [H2O2]0 = 4.5 mM, [SPC]0 = 3 mM, pH0 = 4.0. | |
The chemical stability and reusability of prepared nZVI were evaluated by for its future applications in the wastewater treatment. As shown in the Fig. 2, more than 99% of the BPA was degraded in the first two runs, while about 78% of the BPA was removed in the third run. The catalytic activity of nZVI decreased in the continuous operations, which might be ascribed to two main reasons. The first reason might be the surface corrosion and iron ions dissolution, which reduced the active sites on nZVI. The second one might be the iron ions precipitation products that would deposit on the nZVI surface, hindering the activation of SPC.31 Overall, these results confirmed that nZVI has a prominent catalytic ability with limited reusability in the nZVI/SPC system.
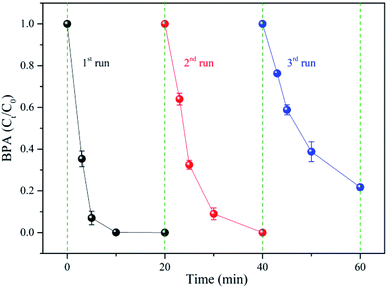 |
| Fig. 2 Reusability of nZVI: [BPA]0 = 0.1 mM, [nZVI]0 = 0.1 g L−1, [SPC]0 = 3 mM, pH0 = 4.0. | |
In order to assess the contribution of leached Fe2+ from nZVI to the SPC activation in the SPC system, the concentration of dissolved Fe2+ was measured and the performance of Fe2+/SPC was compared with nZVI/SPC. As shown in the Fig. 3, the concentration of total Fe (including Fe3+ and Fe2+ ions) gradually increased to 2.3 mg L−1 and then remained relatively stable, which mainly consist of Fe2+. Fe3+ could be rapidly reduced to Fe2+ by nZVI through eqn (8). Since the surface-associated Fe(II) atoms and dissolved Fe2+ ions could also activate H2O2 to decompose the organic contaminants,32,33 2.3 mg L−1 of Fe2+ was added with the SPC to evaluate the performance for BPA removal. As shown in Fig. 1, only about 40% of BPA was eliminated in the Fe2+/SPC system, while more than 99% of BPA was removed in the nZVI/SPC system. Unlike homogeneous catalysis, surface active sites of nZVI could promote catalytic oxidation reaction in heterogeneous processes.33 These results suggested that both heterogeneous and homogeneous catalysis contributed to BPA degradation by nZVI/SPC, but the heterogeneous reactions played a primary role.
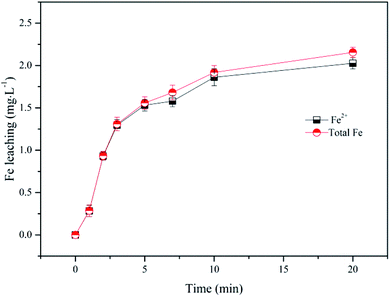 |
| Fig. 3 Fe leaching in nZVI/SPC system: [BPA]0 = 0.1 mM, [nZVI]0 = 0.1 g L−1, [SPC]0 = 3 mM, and pH0 = 4.0. | |
3.2 Effects of operating parameters on BPA degradation
3.2.1 Effects of nZVI loading and SPC dosing on BPA degradation. To explore the effect of catalyst performance, the degradation efficiency of BPA was investigated at different nZVI dosages from 0.05 g L−1 to 0.5 g L−1. As presented in Fig. 4a, BPA removal rate was significantly enhanced with the increase of nZVI dosage from 0.05 g L−1 to 0.2 g L−1. Correspondingly, the observed rate constant (kobs) was enhanced from 0.294 min−1 to 0.694 min−1 (inset in Fig. 4a). More nZVI dosage could provide more active sites for activating SPC, which was conducive to the formation of more free radicals.34 However, with the further increase of nZVI loading (0.2–0.5 g L−1), BPA removal efficiency was significantly inhibited, as the kobs was reduced (inset in Fig. 4a). It was deduced that excess nZVI would lead to agglomeration, which would reduce the effective collision between nZVI and H2O2;34,35 Besides, the superabundant Fe2+ leached from the excess nZVI might scavenge ˙OH through eqn (9).24,25,34 |
Fe2+ + ˙OH → Fe3+ + OH−
| (9) |
 |
| Fig. 4 Influence of nZVI loading (a) and SPC dosing (b) on BPA degradation in nZVI/SPC system: [BPA]0 = 0.1 mM, [nZVI]0 = 0.1 g L−1, [SPC]0 = 3 mM, pH0 = 4.0. | |
The influence of SPC concentration (0.5 mM, 1 mM, 3 mM and 6 mM) in nZVI/SPC system was studied to evaluate the contribution of oxidant on BPA removal. As illustrated in Fig. 4b, BPA degradation efficiency was enhanced when SPC concentration increased from 0.5 mM to 3 mM. However, BPA degradation was slightly restrained with further increase of SPC dose from 3 mM to 6 mM. Moreover, kobs in nZVI/SPC system exhibited a consistent trend (inset in Fig. 4b). These results revealed that SPC with the moderate concentration was beneficial to form sufficient active species, promoting the elimination of BPA.19,35 However, excessive H2O2, disaggregated from SPC in the bulk solution, could play as a scavenger of ˙OH or other radicals as shown in eqn (10) and (11),19,36 causing the decreasing of BPA degradation efficiency.
|
H2O2 + ˙OH → H2O + O2˙−+ H+
| (10) |
|
O2˙− + ˙OH → OH− + O2
| (11) |
3.2.2 Effect of initial pH on BPA degradation. According to the previous work,12 solution pH was a crucial factor in organic pollutants remediation in Fenton or Fenton-like systems. A series of tests were conducted to investigate the effect of initial pH (pH0 at 3.0, 4.0, 5.0 and 6.0) on BPA removal. Fig. 5a showed that BPA oxidation efficiency in nZVI/SPC system was closely related to pH0, implying that BPA degradation was a pH dependent process. It was observed that BPA could be completely removed during 10 min reaction at pH0 = 3.0 and 4.0, especially showing a higher removal rate (85.3%) at the initial reaction (0–1 min). The results were in accordance with the conclusion that acidic pH condition was beneficial to release Fe2+ (Fig. 5b), promoting the generation of ˙OH.37 In contrast, the degradation efficiency of BPA gradually declined with the rise of pH0. For instance, BPA removal rate decreased from 46.0% to 24.1% when the pH0 increased from 5.0 to 6.0. This phenomenon could be explained in three aspects: first, when the solution pH0 increased from 3.0 to 4.0, 5.0 and 6.0, the concentration of Fe2+ gradually dropped from 17.80 mg L−1 to 2.31 mg L−1, 0.14 mg L−1, 0.05 mg L−1 after reaction, respectively. Less Fe2+ was detected under higher pH, leading to the lower production of reactive species. Second, the elevation of pH0 (pH0 > 4) would benefit the precipitation of Fe(OH)3,38 inhibiting the availability of catalytic sites on the surface of nZVI.39 Third, ˙OH has a higher oxidation potential at a lower pH, which was 2.65–2.80 V at pH 3.0 and 1.90 V at pH 7.0.40 Therefore, acid condition was preferred to BPA degradation in the nZVI/SPC system.
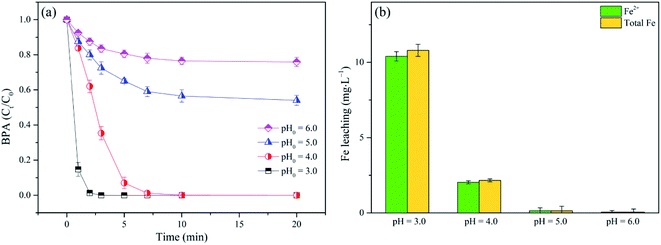 |
| Fig. 5 (a) Effect of pH0 on BPA degradation; (b) Fe leaching in the nZVI/SPC system. General reaction conditions: [BPA]0 = 0.1 mM, [nZVI]0 = 0.1 g L−1, [SPC]0 = 3 mM. | |
3.3 Effect of water matrix on BPA degradation
Inorganic anions such as Cl−, HPO42−, NO3− and NO2− inherently exist in surface and ground water, which might react with free radicals and play an inhibited impact on contaminant degradation in AOPs.13,41,42 In this study, BPA removal by adding different content of Cl−, HPO42−, NO3− and NO2− into nZVI/SPC system was discussed separately. As depicted in Fig. 6a, Cl− at the level of 1–10 mM showed inhibitory effect on BPA removal. It was probably because Cl− could compete with BPA to consume ˙OH to form Cl2 and HOCl with weak oxidation capacity.43 Similarly, Fig. 6b showed that HPO42− exhibited significant negative influence on BPA degradation. With the increase of HPO42− concentration from 1.0 to 5.0 and 10.0 mM, BPA removal decreased from 68.5% to 33.2% and 23.0%, respectively. HPO42− would complex with Fe2+ to block the active sites on the nZVI surface and suppress the released Fe2+, which might inhibit ˙OH generation.6 Additionally, the presence of HPO42−, as one of ˙OH scavengers, could consume ˙OH by the following reaction (eqn (12)).6 |
HPO42− + ˙OH → HPO4˙− + OH−
| (12) |
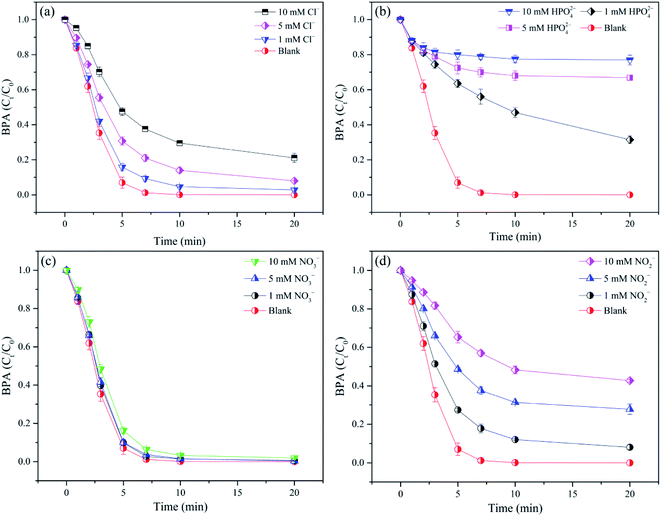 |
| Fig. 6 Effect of anions on BPA degradation in nZVI/SPC system. (a) Cl−; (b) HPO42−; (c) NO3−; (d) NO2−. React conditions: [BPA]0 = 0.1 mM, [nZVI]0 = 0.1 g L−1, [SPC]0 = 3 mM, pH0 = 4.0. | |
In case of NO3−, BPA degradation was slightly inhibited by the addition of 1, 5, and 10 mM NO3− (Fig. 6c), because NO3− was unable to complex with Fe2+ or Fe3+, and also non-reactive to ˙OH.13 Conversely, a strong inhibitory effect on BPA removal was observed in the presence of NO2− (Fig. 6d). This phenomenon could be attributed to the scavenging reaction between NO2− and ˙OH (kNO2−,˙OH = 1.2 × 1010 M−1 s−1), leading to the generation of
NO2⋅ with low oxidation potential.44,45
In addition, natural organic matters (NOMs), the common substances in water bodies, should be considered to investigate the influence on BPA removal in the nZVI/SPC process. Therefore, humic acid (HA), as a kind of representative NOMs, was selected to explore the effect of NOMs on BPA degradation. As shown in Fig. 7, when HA concentration increased to 5 mg L−1 and 10 mg L−1, respectively, BPA removal rate was slightly inhibited. With the further increase of HA concentration to 20 mg L−1, BPA removal efficiency still reached to 83% at 20 min. HA had the ability to consume a certain amount of ˙OH, and compete with target compounds, leading to the lower efficiency in AOPs.36,46 These results indicated that the nZVI/SPC system has potential to resist appropriate amount of NOM interference on BPA degradation.
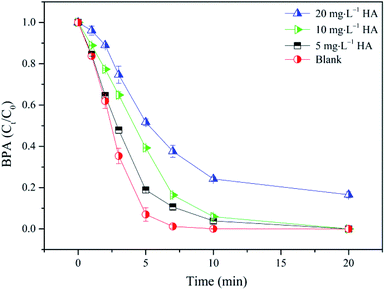 |
| Fig. 7 Effect of HA on BPA degradation in the nZVI/SPC system: [BPA]0 = 0.1 mM, [nZVI]0 = 0.1 g L−1, [SPC]0 = 3 mM, pH0 = 4.0. | |
3.4 Identification of reactive species
As mentioned in previous literatures, ˙OH, O2˙− and CO3˙−were expected in the SPC system.16,17,36 In this work, isopropanol (IPA) was used to study the existence of ˙OH, because it has a high reaction rate with ˙OH (kIPA, ˙OH = 3.9 × 109 M−1 s−1) and a low reaction rate with O2˙− (kIPA, O2˙− = 1 × 106 M−1 s−1) and CO3˙− (kIPA, CO3˙− = 4 × 104 M−1 s−1).47–49 Meanwhile, Phenol (PhOH) was employed as ˙OH and CO3˙− scavenger, which reacts rapidly with ˙OH (kPhOH, ˙OH = 6 × 108 M−1 s−1) and CO3˙− (kPhOH, CO3˙− = 1.2 × 109 M−1 s−1),16,50 and slowly with O2˙− (kPhOH, O2˙− = 5.8 × 102 M−1 s−1).51 BPA degradation performance in the absence and presence of quenching agents was presented in Fig. 8. Both IPA and PhOH could prevent BPA removal in the nZVI/SPC system, and the inhibitory effect of PhOH was more significant. Specifically, in the absence of IPA or PhOH, BPA could be completely degraded in 10 min, whereas BPA removal rate decreased to 18.8% and 0.9% with the addition of 30 mM IPA and PhOH, respectively. This result implied that both ˙OH and CO3˙− participated in the degradation of BPA, and ˙OH was the main reactive oxygen radical for BPA removal in nZVI/SPC system. But the contribution of O2˙− was negligible. This is due to the fact that HCO3− can scavenge O2˙− to be CO3˙−, which selectively react with electron-rich compounds, like phenols.50,52
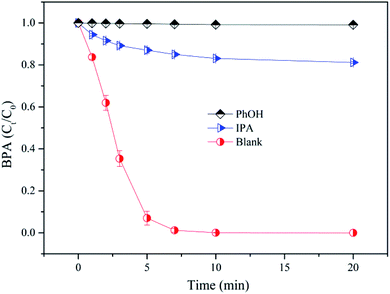 |
| Fig. 8 The effects of IPA and PhOH on BPA degradation in nZVI/SPC system. General reaction conditions: [BPA]0 = 0.1 mM, [nZVI]0 = 0.1 g L−1, [SPC]0 = 3 mM, pH0 = 4.0. | |
3.5 Mechanistic insight of the nZVI/SPC system
As presented in Fig. 9, the XRD patterns of nZVI before use were corresponded to the standard peaks of Fe0 (JCPDS no. 36-0696).53 After 20 min reaction, the new peaks at 26.6°, 35.6° and 60.6° are observed in used nZVI, which are relevant to the crystalline structures of Fe3O4 (JCPDS no. 89-6466), FeO (JCPDS no. 89-0690) and FeOOH (JCPDS no. 70-0410), respectively. These results suggested that iron oxides and oxide-hydroxides were formed on the surface of nZVI during the activation of SPC.
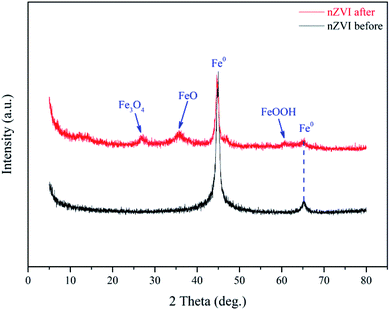 |
| Fig. 9 XRD of nZVI before and after use. | |
To better understand the roles of Fe and O species on the nZVI surface in SPC activation, the XPS spectra of nZVI before and after reactions were recorded. As illustrated in Fig. 10a, the peaks of Fe 2p3/2 at 706.8 eV, 710.3 eV, and 712.7 eV indicated the existence of Fe0, Fe3O4 and FeOOH on the nZVI surface before reaction, respectively.21,53–55 Based on the Fe 2p3/2 spectrum, the Fe0, Fe(II) and Fe(III) content of the nZVI before use were 10.6%, 57.1% and 23.3%, but account for 6.5%, 52.3% and 41.2% for the nZVI after use, respectively. After the reactions, the decrease of Fe0 and Fe(II) indicated that electron transfer occurred on the nZVI surface and Fe2+ was released from the catalyst,20 resulting in the increase of Fe(III) fraction and ˙OH production, according to the eqn (4).
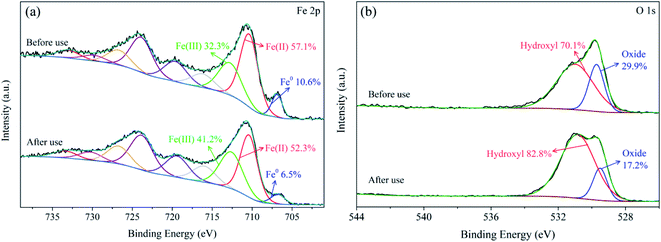 |
| Fig. 10 XPS spectra on Fe 2p (a) and O 1s (b) of nZVI catalyst before and after use. | |
In addition, the O 1s spectrum is shown in Fig. 10b. The two main peaks at 529.7 eV and 531.0 eV were assigned to iron oxide and surface hydroxyl species before reaction, correspondingly.53,55 After reactions, with the decrease of the fraction of iron oxide, an iron hydroxide layer was formed, making the composition of surface hydroxyl species increased. The results further proved that the formation of iron oxides was the important step in activating SPC by nZVI.
Overall, based on the above analysis, the chemical reaction mechanism between nZVI and SPC was proposed as shown in Fig. 11. First, nZVI was corroded to generate Fe(II)-contained oxides on the surface and Fe2+ ions that leached into the reaction solution (eqn (4) and (5)). Then, Fe2+ ions could activate H2O2 directly, generated from SPC, to engender ˙OH and Fe3+. The formed Fe3+ would be transformed to Fe(III)-contained oxides, deposited on the surface of nZVI.53 On the other hand, Fe2+ could be regenerated from the reduction of Fe3+,34,48 further promoting the activation of SPC. In addition, CO32− and HCO3−, as by-products of SPC decomposition, could transform to be CO3˙− through the reaction with ˙OH as shown in eqn (2) and (3). Finally, BPA was degraded to TPs by ˙OH and CO3˙− via direct radical attachment and electron transfer, respectively.
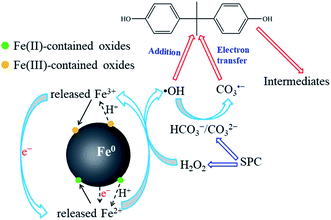 |
| Fig. 11 Proposed mechanism of BPA degradation in the nZVI/SPC system. | |
3.6 BPA degradation pathways
In this study, the TPs of BPA in the nZVI/SPC system were identified by LC-MS. Four compounds were detected with identified m/z values, namely B1 (monohydroxylated BPA, m/z = 243.101), B2 (dihydroxylated BPA, m/z = 259.096), B3 (p-isopropylphenol, m/z = 151.075) and B4 (1-(2,4-dihydroxyphenyl)ethan-1-one, m/z = 151.038). Detailed information was supplied in ESI.† The possible degradation pathway of BPA (Fig. 12) was proposed based on the monitored TPs and the previous studies.56,57 First, ˙OH might attack the aromatic ring of BPA through hydroxylation to produce B1, and further hydroxylation to be B2. On the other hand, BPA could be converted into phenolic-BPA radical cation under the attack of CO3˙− by capturing electrons.16 After further interacted with water, hydroxybenzene TPs (B1) was generated. Then, B1 was hydroxylation into B2 following the same mechanism.16 Second, ˙OH could attack the electron-rich alkyl carbon of BPA to remove a benzene ring, resulting in the formation of alkyl hydroxylation product (B3). Subsequently, B3 was gradually converted to B4 with further oxidized by ˙OH. At last, these BPA TPs would be transformed to the ring-cleavage products via hydroxylation and oxidation,58 and be further mineralized into CO2 and H2O.
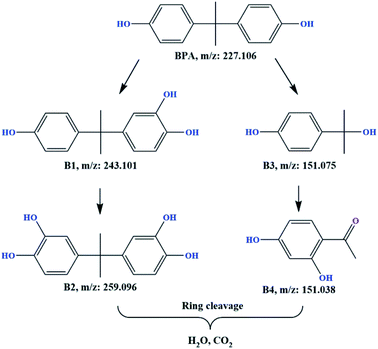 |
| Fig. 12 Possible degradation pathway of BPA in the nZVI/SPC system. Reaction conditions: [BPA]0 = 0.1 mM, [nZVI]0 = 0.1 g L−1, [SPC]0 = 3 mM, pH0 = 4.0. | |
3.7 Mineralization and toxicity assessment during BPA degradation
The mineralization efficiency of BPA in the nZVI/SPC system was assessed through monitoring the change of TOC. As illustrated in Fig. 13, BPA mineralization rate was continuously increased along with the decomposition of BPA. After 20 min, 45.0% of the TOC was removed under the optimized reaction conditions, which implied that BPA was gradually transformed into smaller molecules. However, the bio-toxicity of BPA and TPs showed a different trend compared to the TOC result. The oxygen uptake inhibition rate increased from 47.8% to 62.0% in the first 3 min of reaction, and gradually decreased to 19.6% in the remaining reaction time (3–20 min). Some TPs that were generated during the initial reaction stage (0–3 min) caused higher bio-toxicity than the parent compound, such as B3 and B4.3,59 These toxic TPs were finally disassembled and mineralized to low or non-toxic products in the nZVI/SPC system.
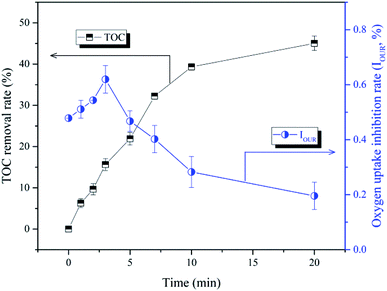 |
| Fig. 13 Changes of TOC and bio-toxicity during BPA treatment in the nZVI/SPC system. Reaction conditions: [BPA]0 = 0.1 mM, [nZVI]0 = 0.1 g L−1, [SPC]0 = 3 mM, pH0 = 4.0. | |
4. Conclusions
In this study, the catalytic efficiency of nZVI particles in activating SPC to remove BPA was investigated for wastewater treatment. BPA degradation efficiency was enhanced with increased nZVI loading and SPC dosing in the adequate range at low initial pH. Furthermore, the Cl−, HPO42−, NO2− anions and HA in aqueous solution inhibited BPA removal in the selected range. In the nZVI/SPC system, ˙OH was the dominant active species, while CO3˙− slightly contributed to the degradation of BPA. In addition, surface corrosion, Fe2+ releasing, and iron species conversion play significant roles in the activation of SPC by nZVI. The possible degradation pathways of BPA were proposed based on the TPs detected by LC-MS. This work supports that the nZVI/SPC system can be used as an efficient, cost effective, and environmental-friendly technology for the remediation of BPA in wastewater treatment, owing to its high catalytic activity, good reusability, and treatment safety.
Conflicts of interest
The authors declare no conflict of interest.
Acknowledgements
This work was financially supported by the National Key Research and Development Program of China (2017YFC0212602), the Open Research Fund of Hubei Key Laboratory of Mine Environmental Pollution Control & Remediation, Hubei Polytechnic University (2020105), the China Postdoctoral Science Foundation (2019M662064).
References
- Y. Xie, P. Li, Y. Zeng, X. Li, Y. Xiao, Y. Wang and Y. Zhang, Chem. Eng. J., 2018, 335, 728–736 CrossRef CAS.
- Y. Wang, S. Zhao, W. Fan, Y. Tian and X. Zhao, Environ. Sci.: Nano, 2018, 5, 1933–1942 RSC.
- J. Deng, M. Xu, C. Qiu, Y. Chen, X. Ma, N. Gao and X. Li, Appl. Surf. Sci., 2018, 459, 138–147 CrossRef CAS.
- J. Liu, L. Zhang, G. Lu, R. Jiang, Z. Yan and Y. Li, Ecotoxicol. Environ. Saf., 2021, 208, 111481 CrossRef CAS.
- S. Yang, P. Wu, J. Liu, M. Chen, Z. Ahmed and N. Zhu, Chem. Eng. J., 2018, 350, 484–495 CrossRef CAS.
- L. Liu, X. Xu, Y. Li, R. Su, Q. Li, W. Zhou, B. Gao and Q. Yue, Chem. Eng. J., 2020, 382, 122780 CrossRef CAS.
- X. Xu, S. Zong, W. Chen and D. Liu, Chem. Eng. J., 2019, 369, 470–479 CrossRef CAS.
- J. Sharma, I. M. Mishra, D. D. Dionysiou and V. Kumar, Chem. Eng. J., 2015, 276, 193–204 CrossRef CAS.
- M. Chen, Z. Zhang, L. Zhu, N. Wang and H. Tang, Chem. Eng. J., 2019, 361, 1190–1197 CrossRef CAS.
- N. Wang, Q. Hu, X. Du, H. Xu and L. Hao, Adv. Powder Technol., 2019, 30, 2369–2378 CrossRef CAS.
- N. Wang, X. Sun, Q. Zhao and P. Wang, Chem. Eng. J., 2021, 406, 126734 CrossRef CAS.
- A. Mirzaei, Z. Chen, F. Haghighat and L. Yerushalmi, Chemosphere, 2017, 174, 665–688 CrossRef CAS.
- M. M. Sablas, M. D. G. de Luna, S. Garcia-Segura, C.-W. Chen, C.-F. Chen and C.-D. Dong, Sep. Purif. Technol., 2020, 250, 117269 CrossRef CAS.
- Y. Li, Y. Zhu, D. Wang, G. Yang, L. Pan, Q. Wang, B.-J. Ni, H. Li, X. Yuan, L. Jiang and W. Tang, Water Res., 2020, 174, 115626 CrossRef CAS.
- Z. Miao, X. Gu, S. Lu, M. L. Brusseau, N. Yan, Z. Qiu and Q. Sui, J. Hazard. Mater., 2015, 300, 530–537 CrossRef CAS.
- J. Gao, X. Duan, K. O'Shea and D. D. Dionysiou, Water Res., 2020, 171, 115394 CrossRef CAS.
- Y. Huang, M. Kong, D. Westerman, E. G. Xu, S. Coffin, K. H. Cochran, Y. Liu, S. D. Richardson, D. Schlenk and D. D. Dionysiou, Environ. Sci. Technol., 2018, 52, 12697–12707 CrossRef CAS.
- Z. W. Miao, X. G. Gu, S. G. Lu, X. K. Zang, X. L. Wu, M. H. Xu, L. B. B. Ndong, Z. F. Qiu, Q. Sui and G. Y. Fu, Chemosphere, 2015, 119, 1120–1125 CrossRef CAS.
- S. Sajjadi, A. Khataee, R. Darvishi Cheshmeh Soltani, N. Bagheri, A. Karimi and A. Ebadi Fard Azar, J. Ind. Eng. Chem., 2018, 68, 406–415 CrossRef CAS.
- C. Kim, J.-Y. Ahn, T. Y. Kim, W. S. Shin and I. Hwang, Environ. Sci. Technol., 2018, 52, 3625–3633 CrossRef CAS.
- J. Cao, L. Lai, B. Lai, G. Yao, X. Chen and L. Song, Chem. Eng. J., 2019, 364, 45–56 CrossRef CAS.
- H. Dong, K. Hou, W. Qiao, Y. Cheng, L. Zhang, B. Wang, L. Li, Y. Wang, Q. Ning and G. Zeng, Chem. Eng. J., 2019, 359, 1046–1055 CrossRef CAS.
- R. Chen, H. Yin, H. Peng, X. Wei, X. Yu, D. Xie, G. Lu and Z. Dang, Environ. Pollut., 2020, 260, 113983 CrossRef CAS.
- W. Zhang, H. Gao, J. He, P. Yang, D. Wang, T. Ma, H. Xia and X. Xu, Sep. Purif. Technol., 2017, 172, 158–167 CrossRef CAS.
- R. Cheng, C. Cheng, G.-h. Liu, X. Zheng, G. Li and J. Li, Chemosphere, 2015, 141, 138–143 CrossRef CAS.
- A. Liu, J. Liu, J. Han and W.-x. Zhang, J. Hazard. Mater., 2017, 322(part A), 129–135 CAS.
- J. Du, J. Bao, C. Lu and D. Werner, Water Res., 2016, 102, 73–81 CrossRef CAS.
- J. Du, J. Bao, Y. Liu, H. Ling, H. Zheng, S. H. Kim and D. D. Dionysiou, J. Hazard. Mater., 2016, 320, 150–159 CrossRef CAS.
- Y. M. Yoon, P. Westerhoff, S. A. Snyder and M. Esparza, Water Res., 2003, 37, 3530–3537 CrossRef CAS.
- M. L. Dell'Arciprete, J. M. Soler, L. Santos-Juanes, A. Arques, D. O. Mártire, J. P. Furlong and M. C. Gonzalez, Water Res., 2012, 46, 3479–3489 CrossRef.
- H. Liu, J. Yao, L. Wang, X. Wang, R. Qu and Z. Wang, Chem. Eng. J., 2019, 358, 1479–1488 CrossRef CAS.
- W. Jiang, D. D. Dionysiou, M. Kong, Z. Liu, Q. Sui and S. Lyu, Chem. Eng. J., 2020, 380, 122537 CrossRef CAS.
- J. A. Donadelli, L. Carlos, A. Arques and F. S. García Einschlag, Appl. Catal., B, 2018, 231, 51–61 CrossRef CAS.
- L. Wang, J. Yang, Y. Li, J. Lv and J. Zou, Chem. Eng. J., 2016, 284, 1058–1067 CrossRef CAS.
- M. Danish, X. Gu, S. Lu, A. Ahmad, M. Naqvi, U. Farooq, X. Zhang, X. Fu, Z. Miao and Y. Xue, Chem. Eng. J., 2017, 308, 396–407 CrossRef CAS.
- H. Cui, X. Gu, S. Lu, X. Fu, X. Zhang, G. Y. Fu, Z. Qiu and Q. Sui, Chem. Eng. J., 2017, 309, 80–88 CrossRef CAS.
- I. Hussain, M. Y. Li, Y. Q. Zhang, Y. C. Li, S. B. Huang, X. D. Du, G. Q. Liu, W. Hayat and N. Anwar, Chem. Eng. J., 2017, 311, 163–172 CrossRef CAS.
- M. Danish, X. G. Gu, S. G. Lu, M. H. Xu, X. Zhang, X. R. Fu, Y. F. Xue, Z. W. Miao, M. Naqvi and M. Nasir, Res. Chem. Intermed., 2016, 42, 6959–6973 CrossRef CAS.
- R. Li, X. Jin, M. Megharaj, R. Naidu and Z. Chen, Chem. Eng. J., 2015, 264, 587–594 CrossRef CAS.
- A. A. Burbano, D. D. Dionysiou, M. T. Suidan and T. L. Richardson, Water Res., 2005, 39, 107–118 CrossRef CAS.
- X. Y. Yu and J. R. Barker, J. Phys. Chem. A, 2003, 107, 1313–1324 CrossRef CAS.
- X. Y. Lou, L. X. Wu, Y. G. Guo, C. C. Chen, Z. H. Wang, D. X. Xiao, C. L. Fang, J. S. Liu, J. C. Zhao and S. Y. Lu, Chemosphere, 2014, 117, 582–585 CrossRef CAS.
- Y. Yang, J. J. Pignatello, J. Ma and W. A. Mitch, Environ. Sci. Technol., 2014, 48, 2344–2351 CrossRef CAS.
- C. Chen, Z. Wu, S. Zheng, L. Wang, X. Niu and J. Fang, Environ. Sci. Technol., 2020, 54, 8455–8463 CrossRef CAS.
- J. Li, M. Xu, G. Yao and B. Lai, Chem. Eng. J., 2018, 348, 1012–1024 CrossRef CAS.
- X. Fu, X. Gu, S. Lu, Z. Miao, M. Xu, X. Zhang, Z. Qiu and Q. Sui, Chem. Eng. J., 2015, 267, 25–33 CrossRef CAS.
- P. Yan, Q. Sui, S. Lyu, H. Hao, H. F. Schröder and W. Gebhardt, Sci. Total Environ., 2018, 640–641, 973–980 CrossRef CAS.
- X. Fu, X. Gu, S. Lu, V. K. Sharma, M. L. Brusseau, Y. Xue, M. Danish, G. Y. Fu, Z. Qiu and Q. Sui, Chem. Eng. J., 2017, 309, 22–29 CrossRef CAS.
- T. Liu, K. Yin, C. Liu, J. Luo, J. Crittenden, W. Zhang, S. Luo, Q. He, Y. Deng, H. Liu and D. Zhang, Water Res., 2018, 147, 204–213 CrossRef CAS.
- T. Zhao, P. Li, C. Tai, J. She, Y. Yin, Y. a. Qi and G. Zhang, J. Hazard. Mater., 2018, 346, 42–51 CrossRef CAS.
- Y. Tsujimoto, H. Hashizume and M. Yamazaki, Int. J. Biochem., 1993, 25, 491–494 CrossRef CAS.
- Y. Liu, X. He, X. Duan, Y. Fu, D. Fatta-Kassinos and D. D. Dionysiou, Water Res., 2016, 95, 195–204 CrossRef CAS.
- C. Tan, Y. Dong, D. Fu, N. Gao, J. Ma and X. Liu, Chem. Eng. J., 2018, 334, 1006–1015 CrossRef CAS.
- J. Wu, B. Wang, L. Blaney, G. Peng, P. Chen, Y. Cui, S. Deng, Y. Wang, J. Huang and G. Yu, Chem. Eng. J., 2019, 361, 99–108 CrossRef CAS.
- C. Ding, S. Xiao, Y. Lin, P. Yu, M.-e. Zhong, L. Yang, H. Wang, L. Su, C. Liao, Y. Zhou, Y. Deng and D. Gong, Chem. Eng. J., 2019, 360, 104–114 CrossRef CAS.
- R. A. Torres, F. Abdelmalek, E. Combet, C. Petrier and C. Pulgarin, J. Hazard. Mater., 2007, 146, 546–551 CrossRef CAS.
- X. Li, Z. H. Wang, B. Zhang, A. I. Rykov, M. A. Ahmed and J. H. Wang, Appl. Catal., B, 2016, 181, 788–799 CrossRef CAS.
- Z. Dong, Q. Zhang, B.-Y. Chen and J. Hong, Chem. Eng. J., 2019, 357, 337–347 CrossRef CAS.
- R. Mtibaà, D. R. Olicón-Hernández, C. Pozo, M. Nasri, T. Mechichi, J. González and E. Aranda, Ecotoxicol. Environ. Saf., 2018, 156, 87–96 CrossRef.
Footnote |
† Electronic supplementary information (ESI) available. See DOI: 10.1039/d0ra08395j |
|
This journal is © The Royal Society of Chemistry 2021 |
Click here to see how this site uses Cookies. View our privacy policy here.