DOI:
10.1039/D0RA09247A
(Paper)
RSC Adv., 2021,
11, 3216-3220
Synthesis of 2-ethoxycarbonylthieno[2,3-b]quinolines in biomass-derived solvent γ-valerolactone and their biological evaluation against protein tyrosine phosphatase 1B†
Received
30th October 2020
, Accepted 24th December 2020
First published on 14th January 2021
Abstract
A series of 2-ethoxycarbonylthieno[2,3-b]quinolines were synthesized in the bio-derived “green” solvent γ-valerolactone (GVL) and evaluated for their inhibitory activities against PTP1B, the representative compound 6a displayed an IC50 value of 8.04 ± 0.71 μM with 4.34-fold preference over TCPTP. These results provided novel lead compounds for the design of inhibitors of PTP1B as well as other PTPs.
Introduction
Protein tyrosine phosphatase 1B (PTP1B) is a prototypic member of the protein tyrosine phosphatase (PTP) family that appears to be involved in the regulation of several cellular functions, including insulin cascade,1 and other important pathways related to human breast and ovarian cancers.2 Consequently, the inhibition of PTP1B is considered to be a potential therapeutic for the treatment of type 2 diabetes and cancers. Various PTP1B inhibitors have been developed over the past decade.3 However, only two candidates have entered clinical trials while no commercial drugs have been approved to date due mainly to limited bioavailability.3 Therefore, the rapid development of a potent and bioavailable PTP1B specific inhibitor remains necessary.
Thienoquinolines are an important subset of the quinoline family and consequential structural units in the domain of medicinal chemistry due to their myriad bioactivities.4–6 This class of compounds are well documented with urea transporter inhibitory (compound 1),7 anti-inflammatory (compound 2),8 anti-oxidant (compound 3)8 activities (Fig. 1).
 |
| Fig. 1 Biologically active thienoquinolines. | |
Our efforts to develop modulators of protein tyrosine phosphatases started from 1H-2,3-dihydroperimidines.9–14 Using the scaffold hopping strategy,15,16 we developed 3-aryl-1-oxa-2,8-diazaspiro[4.5]dec-2-enes11 as PTP1B inhibitors, bis-aryl amides9 and benzo[c][1,2,5]thiadiazoles as SH2-Containing Protein Tyrosine Phosphatase-2 (SHP2) inhibitors.13 We noticed that the scaffold of thieno[2,3-b]quinolines showed high similarity with that of 1H-2,3-dihydroperimidines and might provide novel scaffold to develop novel PTP1B inhibitors (Fig. 2).
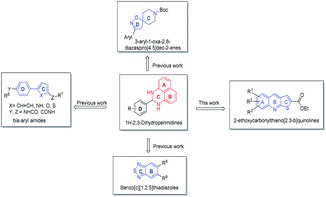 |
| Fig. 2 The scaffolds of modulators of protein tyrosine phosphatases derived from 1H-2,3-dihydroperimidines. | |
In the pharmaceutical industry, there is a strong desire to embed sustainable principles earlier in the drug discovery process,17,18 in which the development of more environmentally friendly methods for the isolation and purification of active molecules continues to represent a major challenge.17 There are many examples of Green Chemistry principles successfully applied in medicinal chemistry,17–21 such as using microwave chemistry22,23 and high-throughput screening18,24 to provide drug candidates consuming less material, with less waste, and in less time. In recent years there has been a trend towards the use of bio-derived, green solvents as replacements for hazardous and/or environmentally-damaging solvents for oxidation, nitration, rearrangement, phosphonylation, amide condensation, urea formation, and metal catalyzed cross-coupling.21,25–37 Increasing attention is directed to γ-valerolactone (GVL),38–44 which is a naturally occurring chemical found in fruits.38 Due to the low toxicity and the outstanding physicochemical properties of biodegradability and low vapor pressure,38 GVL has been recognized as sustainable dipolar aprotic solvents and has been developed as a greener alternative to classical solvents used in metal-catalyzed cross-coupling reactions,44 transformation of CO2 to formamides,38 and membrane preparation.41 With our interest in the area of sustainable synthesis in discovery-phase medicinal chemistry,45 we developed an environmentally benign and sustainable protocol for the synthesis of 2-ethoxycarbonylthieno[2,3-b]quinolines by condensation of 2-chloro-3-formylquinolines with ethyl mercaptoacetate at 90 °C in γ-valerolactone as a solvent, in which the simple addition of water allowed for complete removal of GVL without the need for extensive isolation and purification.
The obtained 2-ethoxycarbonylthieno[2,3-b]quinolines were evaluated for their inhibitory activities against PTP1B, several derivatives were identified as PTP1B inhibitors, the representative compound 6a was also subjected to selectivity analyses to determine whether its biological properties made it suitable for further development.
Results and discussion
To optimize the experimental parameters (Table 1), 2-chloroquinoline-3-carbaldehyde (4a), which was prepared as ref. 7, was chosen as model substrate using precipitate procedure by simple addition of water (Table 1). A series of bases, such as Na2CO3, DIPEA, DBU, and Et3N, were investigated at six equivalents compared to 2-chloroquinoline-3-carbaldehyde. Among them, Et3N showed best result with 66% yield (entry 4). Increase or decrease of the equivalent of Et3N showed no positive effects on the yield (entries 5 and 6). After investigation of the equivalent of ethyl mercaptoacetate (entries 7 and 8), the results demonstrated that 1.2 equivalent was the optimal (entry 4). The reaction temperature also was investigated (entries 9–11), and the results displayed that the temperature obviously affected the yield and the suitable temperature was 90 °C (entry 4). After an increase in concentration (entries 12–14), the yield was improved remarkably and the suitable concentration was 0.5 mol L−1 (entry 13). Prolonged the reaction time from one hour (entry 13) to two hours (entry 15) did not benefit the reaction yield.
Table 1 Optimization of synthesis of 2-ethoxycarbonylthieno[2,3-b]quinoline (6a) in GVL

|
Entry |
Base (equiv.) |
5a (equiv.) |
Concentration 4a (mol L−1) |
Temp. (oC) |
Time (h) |
Yield (%) |
Precipitation and washing. |
1 |
Na2CO3 (6) |
1.2 |
0.05 |
90 |
1 |
49a |
2 |
DIPEA (6) |
1.2 |
0.05 |
90 |
1 |
21a |
3 |
DBU (6) |
1.2 |
0.05 |
90 |
1 |
56a |
4 |
Et3N (6) |
1.2 |
0.05 |
90 |
1 |
66a |
5 |
Et3N (4) |
1.2 |
0.05 |
90 |
1 |
59a |
6 |
Et3N (8) |
1.2 |
0.05 |
90 |
1 |
59a |
7 |
Et3N (6) |
1 |
0.05 |
90 |
1 |
62a |
8 |
Et3N (6) |
1.4 |
0.05 |
90 |
1 |
65a |
9 |
Et3N (6) |
1.2 |
0.05 |
30 |
1 |
Trace |
10 |
Et3N (6) |
1.2 |
0.05 |
60 |
1 |
53a |
11 |
Et3N (6) |
1.2 |
0.05 |
120 |
1 |
63a |
12 |
Et3N (6) |
1.2 |
0.1 |
90 |
1 |
73a |
13 |
Et3N (6) |
1.2 |
0.5 |
90 |
1 |
82a |
14 |
Et3N (6) |
1.2 |
0.8 |
90 |
1 |
76a |
15 |
Et3N (6) |
1.2 |
0.5 |
90 |
2 |
82a |
Based on the optimized reaction conditions, a series of 2-ethoxycarbonylthieno[2,3-b]quinolines were synthesized from compounds 4a–4n, which were prepared as ref. 7. The results showed that this protocol could be applied to various of 2-chloroquinoline-3-carbaldehydes with electro-withdrawing and electro-donating group on phenyl ring, and had good substrate compatibility in moderate to excellent yields from 51% to 92% (Table 2).
Table 2 Synthesis of 2-ethoxycarbonylthieno[2,3-b]quinolines under optimal conditions
Protein tyrosine phosphatase 1B inhibitory activities and structure–activity relationships
The inhibitory activities of all synthesized compounds against PTP1B were measured using 6,8-difluoro-4-methylumbelliferyl phosphate (DiFMUP) as the substrate and NSC-87877 as positive control,46–48 and the results were detailed in Table 3. As for compounds 6a–6b, compound 6a with chloride at 8 position showed 98.8 ± 0.1% inhibition at the concentration of 50 μM, exhibited obviously better inhibitory activity against PTP1B compared to the compound 6b with chloride at the 9 position. This result indicated that the position of chloride on the phenyl ring significantly affected the inhibitory activity. As for compounds 6a, 6c–6f with substitutes on the 8 position, compounds 6a with chloride and compound 6c with bromide showed excellent inhibitory activities against PTP1B at the concentration of 50 μM, compound 6e with trifluoromethyl group exhibited moderate inhibitory activity, while compound 6d with fluoride and compound 6f with H atom obviously lose inhibitory activity. These results indicated that the electronic property and substitute size played important role on the inhibitory activity. Among compounds (6g–6i) with methyl group on the phenyl ring, compound 6g showed better inhibitory activity than the compound 6h and 6i. These results were similar with that from compounds with chloride (compounds 6a and 6b) and further demonstrated that the position of substrates on phenyl ring affected the inhibitory activities. Among compounds with methoxyl group (6j–6n), all of them did not show obviously inhibitory activities. These results implied that electro-donating group on the phenyl ring was detrimental to the inhibitory activity. In general, the biological activities of 2-ethoxycarbonylthieno[2,3-b]quinolines were affected by three issues of the substitute on phenyl ring A, including position, electronic property and substitute size.
Table 3 Protein tyrosine phosphatase 1B inhibitory activities of compounds 6a–6n
Comp. |
Inhibition (%) at 50 μM |
IC50a |
Comp. |
Inhibition (%) at 50 μM |
IC50a |
IC50 values were determined by regression analyses and expressed as means ± SD of three replications. NT means not tested. |
6a |
98.8 ± 0.1 |
8.04 ± 0.71 |
6h |
12.7 ± 6.5 |
NTb |
6b |
45.7 ± 9.5 |
NTb |
6i |
22.5 ± 1.4 |
NTb |
6c |
98.2 ± 0.1 |
8.96 ± 1.22 |
6j |
20.8 ± 1.9 |
NTb |
6d |
26.1 ± 6.5 |
NTb |
6k |
21.6 ± 3.2 |
NTb |
6e |
71.6 ± 3.0 |
21.21 ± 1.50 |
6l |
23.0 ± 0.4 |
NTb |
6f |
4.5 ± 2.6 |
NTb |
6m |
35.5 ± 2.1 |
NTb |
6g |
52.1 ± 4.8 |
NTb |
6n |
25.2 ± 0.5 |
NTb |
NSC-87877 |
— |
26.18 ± 8.58 |
— |
— |
|
Selectivity against other PTPs
PTP1B shares the close homology with other PTPs, for example, T-cell protein tyrosine phosphatase (TCPTP) shares a structurally very similar active site with PTP1B and about 80% homologous in the catalytic domain, making it difficult to design inhibitors that are specific for PTP1B.46 In addition to the potency exploration, we investigated the selectivity of the representative compounds 6a, 6c against other PTPs (TCPTP, SHP-2) (Table 4). Homogeneous T-cell protein tyrosine phosphatase (TCPTP) inhibitory activities were investigated simultaneously by the same method.47–49 Compounds 6a and 6c exhibited 4.34-fold and 3.43-fold greater selectivity for PTP1B than for TCPTP respectively. Besides TCPTP, we tested the inhibitory activity of 6a and 6c against SHP2, both of them showed similar activities against PTP1B. These results indicated that the scaffold of thieno[2,3-b]quinolines preferred PTP1B compared to the homogeneous scaffolds, such as bis-aryl amides and benzo[c][1,2,5]thiadiazoles fit for SHP2, and the scaffold hopping strategy provided an efficient way to obtain selective modulator for specific PTPs.
Table 4 The IC50 values of compounds 6a and 6c against PTPsa
Comp. |
IC50 (μM) |
TCPTP/PTP1B |
PTP1B |
TCPTP |
SHP2 |
TCPTP, T-cell protein tyrosine phosphatase; SHP-2, SH2-Containing Protein Tyrosine Phosphatase-2; IC50 values were determined by regression analyses and expressed as means ± SD of three replications. |
6a |
8.04 ± 0.71 |
34.93 ± 3.21 |
12.17 ± 3.13 |
4.34 |
6c |
8.96 ± 1.22 |
30.75 ± 2.97 |
7.84 ± 0.98 |
3.43 |
NSC-87877 |
26.18 ± 8.58 |
71.87 ± 3.87 |
5.09 ± 2.03 |
2.74 |
Conclusions
In summary, we have developed a sustainable protocol for synthesis of 2-ethoxycarbonylthieno[2,3-b]quinolines with a simple precipitate procedure by addition of water for the removal of the high boiling solvent GVL, providing an important alternative approach to the current industrial use of toxic solvent DMF and THF. In addition, Biological evaluation demonstrated that some of the synthesized 2-ethoxycarbonylthieno[2,3-b]quinolines showed inhibitory activity against PTP1B, and the representative compound 6a displayed an IC50 value of 8.04 ± 0.71 μM with 4.34-fold preference over TCPTP. These preliminary results provided a possible opportunity for the development of novel PTP1B inhibitors.
Conflicts of interest
The authors have declared no conflict of interest.
Acknowledgements
This work was supported by National Natural Science Foundation of China (21772068), National Science & Technology Major Project “Key New Drug Creation and Manufacturing Program”, China (Number: 2018ZX09711002), Natural Science Foundation of Jiangsu Province (BK20190608).
Notes and references
- D. E. Moller, Nature, 2001, 414, 821–827 CrossRef CAS.
- R. A. Bartolome, Á. Martín-Regalado, M. Jaén, M. Zannikou, P. Zhang, V. Ríos, I. V. Balyasnikova and J. I. Casal, Cancers, 2020, 12, 500 CrossRef CAS.
- H. Hussain, I. R. Green, G. Abbas, S. M. Adekenov, W. Hussain and I. Ali, Expert Opin. Ther. Pat., 2019, 29, 689–702 CrossRef CAS.
- L. Wu, Y. Wang, H. Song, L. Tang, Z. Zhou and C. Tang, Adv. Synth. Catal., 2013, 355, 1053–1057 CrossRef CAS.
- B. M. Kiran, B. P. Nandeshwarappa, V. P. Vaidya and K. M. Mahadevan, Phosphorus, Sulfur Silicon Relat. Elem., 2007, 182, 969–980 CrossRef CAS.
- A. A. Geies, E. A. Bakhite and H. S. El-Kashef, Pharmazie, 1998, 53, 686 CAS.
- Y. Zhao, M. Li, B. Li, S. Zhang, A. Su, Y. Xing, Z. Ge, R. Li and B. Yang, Eur. J. Med. Chem., 2019, 172, 131–142 CrossRef CAS.
- P. Mahajan, M. Nikam, A. Asrondkar, A. Bobade and C. Gill, J. Heterocycl. Chem., 2016, 54, 1415–1422 CrossRef.
- R. Satheeshkumar, R. Zhu, B. Feng, C. Huang, Y. Gao, L. Gao, C. Shen, T. Hou, J. Li, Y. Zhou and W. Wang, Bioorg. Med. Chem. Lett., 2020, 30, 127170 CrossRef CAS.
- W. Wang, C. Huang, L. Gao, C. Tang, J. Wang, M. Wu, L. Sheng, H. Chen, F. Nan, J. Li, J. Li and B. Feng, Bioorg. Med. Chem. Lett., 2014, 24, 1889–1894 CrossRef CAS.
- W. Wang, X. Chen, L. Gao, L. Sheng, J. Li, j. Li and B. Feng, Chem. Biol. Drug Des., 2015, 86, 1161–1167 CrossRef CAS.
- W. Wang, H. Luo, Y. Gao, L. Gao, L. Sheng, Y. Zhou, J. Li, J. Li and B. Feng, Chin. J. Org. Chem., 2016, 36, 2142–2149 CrossRef CAS.
- W. Wang, X. Chen, Y. Gao, L. Gao, L. Sheng, J. Zhu, L. Xu, Z. Ding, C. Zhang, J. Li, J. Li and Y. Zhou, Bioorg. Med. Chem. Lett., 2017, 27, 5154–5157 CrossRef CAS.
- W. Wang, D. Yang, L. Gao, C. Tang, W. Ma, H. Ye, S. Zhang, Y. Zhao, H. Xu, Z. Hu, X. Chen, W. Fan, H. Chen, J. Li, F. Nan, J. Li and B. Feng, Molecules, 2013, 19, 102–121 CrossRef.
- G. Schneider, W. Neidhart, T. Giller and G. Schmid, Angew. Chem., 1999, 38, 2894–2896 CrossRef CAS.
- G. Hessler and K. Baringhaus, Drug Discovery Today: Technol., 2010, 7, e263–e269 CrossRef CAS.
- M. C. Bryan, B. Dillon, L. G. Hamann, G. J. Hughes, M. E. Kopach, E. A. Peterson, M. Pourashraf, I. Raheem, P. Richardson, D. Richter and H. F. Sneddon, J. Med. Chem., 2013, 56, 6007–6021 CrossRef CAS.
- I. Aliagas, R. Berger, K. Goldberg, R. T. Nishimura, J. Reilly, P. Richardson, D. Richter, E. C. Sherer, B. A. Sparling and M. C. Bryan, J. Med. Chem., 2017, 60, 5955–5968 CrossRef CAS.
- J. B. Zimmerman, P. T. Anastas, H. C. Erythropel and W. Leitner, Science, 2020, 367, 397–400 CrossRef CAS.
- J. L. Tucker, Org. Process Res. Dev., 2006, 10, 315–319 CrossRef CAS.
- R. A. Sheldon, Curr. Opin. Green Sustainable Chem., 2018, 18, 13–19 CrossRef.
- B. P. Nandeshwarappa, D. B. Aruna Kumar, H. S. Bhojya Naik and K. M. Mahadevan, J. Sulfur Chem., 2005, 26, 373–379 CrossRef CAS.
- A. Kumar, Y. E. Jad, J. M. Collins, F. Albericio and B. G. D. L. Torre, ACS Sustainable Chem. Eng., 2018, 6, 8034–8039 CrossRef CAS.
- K. D. Collins, T. Gensch and F. Glorius, Nat. Chem., 2014, 6, 859–871 CrossRef CAS.
- A. Widener, C&EN Global Enterprise, 2019, 97, 14 Search PubMed.
- F. Gao, R. Bai, F. Ferlin, L. Vaccaro, M. Li and Y. Gu, Green Chem., 2020, 22, 6240–6257 RSC.
- R. K. Henderson, C. Jiménez-González, D. J. C. Constable, S. R. Alston, G. G. A. Inglis, G. Fisher, J. Sherwood, S. P. Binks and A. D. Curzons, Green Chem., 2011, 13, 854–862 RSC.
- C. J. Clarke, W. Tu, O. Levers, A. Bröhl and J. P. Hallett, Chem. Rev., 2018, 118, 747–800 CrossRef CAS.
- B. H. Lipshutz, F. Gallou and S. Handa, ACS Sustainable Chem. Eng., 2016, 4, 5838–5849 CrossRef CAS.
- Z. Li, K. H. Smith and G. W. Stevens, Chin. J. Chem. Eng., 2016, 24, 215–220 CrossRef CAS.
- H. J. Salavagione, J. Sherwood, M. D. Bruyn, V. L. Budarin, G. J. Ellis, J. H. Clark and P. S. Shuttleworth, Green Chem., 2017, 19, 2550–2560 RSC.
- S. Lawrenson, M. North, F. Peigneguy and A. Routledge, Green Chem., 2017, 19, 952–962 RSC.
- F. G. Calvo-Flores, M. J. Monteagudo-Arrebola, J. A. Dobado and J. Isac-García, Top. Curr. Chem., 2018, 376, 18 CrossRef.
- U. Tilstam, Org. Process Res. Dev., 2012, 16, 1273–1278 CrossRef CAS.
- L. Mistry, K. Mapesa, T. W. Bousfield and J. E. Camp, Green Chem., 2017, 19, 2123–2128 RSC.
- T. W. Bousfield, K. P. R. Pearce, S. B. Nyamini, A. Angelis-Dimakis and J. E. Camp, Green Chem., 2019, 21, 3675–3681 RSC.
- K. L. Wilson, J. Murray, C. Jamieson and A. J. B. Watson, Org. Biomol. Chem., 2018, 16, 2851–2854 RSC.
- J. Song, B. Zhou, H. Liu, C. Xie, Q. Meng, Z. Zhang and B. Han, Green Chem., 2016, 18, 3956–3961 RSC.
- O. A. Musaimi, A. El-Faham, A. Basso, B. G. D. l. Torre and F. Albericio, Tetrahedron Lett., 2019, 60, 151058 CrossRef.
- H. Q. Lê, J. Pokki, M. Borrega, P. Uusi-Kyyny, V. Alopaeus and H. Sixta, Ind. Eng. Chem. Res., 2018, 57, 15147–15158 CrossRef.
- M. A. Rasool and I. F. J. Vankelecom, Green Chem., 2019, 21, 1054–1064 RSC.
- J. Winters, W. Dehaen and K. Binnemans, Green Chem., 2020, 22, 6127–6136 RSC.
- F. Ferlin, L. Luciani, S. Santoro, A. Marrocchi, D. Lanari, A. Bechtoldt, L. Ackermann and L. Vaccaro, Green Chem., 2018, 20, 2888–2893 RSC.
- G. Strappaveccia, E. Ismalaj, C. Petrucci, D. Lanari, A. Marrocchi, M. Drees, A. Facchetti and L. Vaccaro, Green Chem., 2015, 17, 365–372 RSC.
- C. Zhang, R. Zhu, Z. Wang, B. Ma, A. Zajac, M. Smiglak, C. Xia, S. L. Castle and W. Wang, RSC Adv., 2019, 9, 2199–2204 RSC.
- L. F. Iversen, K. B. Moller, A. K. Pedersen, G. H. Peters, A. S. Petersen, H. S. Andersen, S. Branner, S. B. Mortensen and N. P. Moller, J. Biol. Chem., 2002, 277, 19982 CrossRef CAS.
- L. K. Debarba, F. M. V. Vechiato, H. Veida-Silva, B. C. Borges, M. C. Jamur, J. Antunes-Rodrigues and L. L. K. Elias, Mol. Cell. Endocrinol., 2019, 482, 62–69 CrossRef CAS.
- S. Welte, K. Baringhaus, W. Schmider, G. Müller, S. Petry and N. Tennagels, Anal. Biochem., 2005, 338, 32–38 CrossRef CAS.
- N. Krishnan, D. Koveal, D. H. Miller, B. Xue, S. D. Akshinthala, J. Kragelj, M. R. Jensen, C. Gauss, R. Page, M. Blackledge, S. K. Muthuswamy, W. Peti and N. K. Tonks, Nat. Chem. Biol., 2014, 10, 558–566 CrossRef CAS.
Footnotes |
† Electronic supplementary information (ESI) available. See DOI: 10.1039/d0ra09247a |
‡ These authors contributed equally to this work. |
|
This journal is © The Royal Society of Chemistry 2021 |
Click here to see how this site uses Cookies. View our privacy policy here.