DOI:
10.1039/D0RA09846A
(Review Article)
RSC Adv., 2021,
11, 2112-2125
Recent progress in application of nanocatalysts for carbonylative Suzuki cross-coupling reactions
Received
19th November 2020
, Accepted 15th December 2020
First published on 21st January 2021
Abstract
In the past few decades, cross-coupling of aryl halides and arylboronic acids in the presence of carbon monoxide (CO), also called carbonylative Suzuki coupling, to form two new carbon–carbon bonds in the production of synthetically and biologically important biaryl ketones, has been widely studied. Consequently, various catalytic systems have been extensively investigated in order to maximize the efficiency of this appealing area of biaryl ketone synthesis. As evidenced in the literature, nanometal-based systems are among the most powerful catalysts for this transformation as their large surface area to volume ratio and reactive morphologies allow faster reaction rates under milder CO pressure even at very low catalyst loadings. This review aims to provide an overview of the recent advances and achievements in the application of nano-sized metal catalysts for carbonylative Suzuki cross-coupling reactions, which may serve as an inspiration to researchers in their future work.
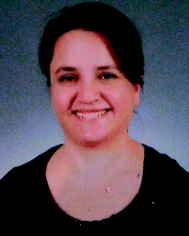 Inci Söğütlü | Inci Söğütlü was born in 1977 in Hatay Turkey. She completed her primary school education in K.Maraş and her secondary and high school education in Hatay. In 1995, she won Yüzüncü Yıl University, Faculty of Arts and Sciences, Department of Chemistry and graduated in 1999. In 2000, she started her Master's degree at Yüzüncü Yıl University, Institute of Health Sciences, Department of Veterinary Biochemistry. On November 12, 2001, she passed the Research Assistant exam at the Faculty of Veterinary Medicine, Department of Biochemistry. She completed her doctorate education in 2007. She started to work in the food supplement department in the Ministry of Agriculture and Forestry in 2007 and still continues to work. |
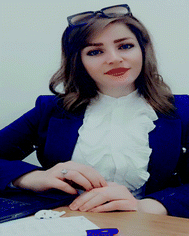 Evan Abdulkarim Mahmood | Evan Abdulkarim Mahmood was born in Erbil, Kurdistan Region, Iraq, in 1990. She is a Biochemistry lecturer. She lives in Iraq/Kurdistan Region. About her educational background, she got a Bachelor degree of Chemistry from University of Sulaimaniyah, College of Science. She completed her Master Degree in Biochemistry at Yüzüncü Yıl University at Van city, Turkey. She is an Assistant Lecturer at University of Human Development, College of Health Science. Also, she worked as a Head of Pharmacy department at National Institute in Sulaimaniyah/Iraq. Her research interests include health, environment, chemistry, clinical biochemistry science. |
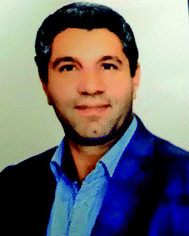 Saeid Ahmadizadeh | Saeid Ahmadizadeh Shendy was born in Tabriz, Iran, in 1973. He received his B.S. degree in pure chemistry from University of Tabriz, Iran, and his M.S. degree in apply chemistry from Azad University, Tabriz, Iran, in 2007 under the supervision of Prof. L. Ejlali. He completed his Ph.D. degree in 2019 under the supervision of Prof. M. Babazadeh. Now he is working at Azad University of Tabriz as Assistant Professor in organic chemistry. His research interests include medicinal chemistry, polymer science, green chemistry, nano chemistry. |
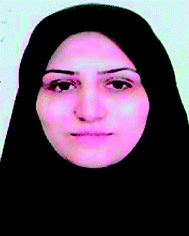 Saeideh Ebrahimiasl | Saeideh Ebrahimiasl was born in Tabriz, Iran, in 1973. She received her PhD degree in nanomaterials and nanotechnology from the Institute of Advanced Materials, University Putra Malaysia in 2010. Professor Majid Monajjemi, Professor Wan Zin Wan Yunus, Professor Zulkarnain Zainal, Professor Mohd Zobir in physical chemistry, nanotechnology and electrochemistry were her most famous supervisors and examiners. Now she is Head of the Industrial nanotechnology Research Center in Tabriz and is an academic member of the Islamic Azad University of Ahar. Her research interests include synthesis and application of nano-materials in different sciences. |
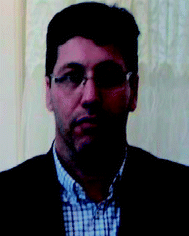 Esmail Vessally | Esmail Vessally was born in Sharabiyan, Sarab, Iran, in 1973. He received his B.S. degree in pure chemistry from University of Tabriz, Tabriz, Iran, and his M.S. degree in organic chemistry from Tehran University, Tehran, Iran, in 1999 under the supervision of Prof. H. Pirelahi. He completed his Ph.D. degree in 2005 under the supervision of Prof. M. Z. Kassaee. Now he is working at Payame Noor University as a full Professor in organic chemistry. His research interests include theoretical organic chemistry, new methodologies in organic synthesis and spectral studies of organic compounds. |
1. Introduction
Biaryl ketones as ubiquitous structural motifs found in a variety of FDA-approved drugs and biologically active natural products.1–7 For example (Fig. 1), fenofibrate with the brand name Tricor is an antilipemic agent marketed in a number of countries worldwide for the treatment of high blood lipid levels. The drug works by increasing the enzyme lipoprotein lipase that breaks down fats in the blood. Mebendazole (Vermox) is a promising gastrointestinal antibiotic used to treat intestinal infections caused by worms such as pinworm, roundworm, and hookworm. It works by preventing the worms from absorbing sugars which they need to survive. Tiaprofenic acid sold under the trade name Surgam among others is a non-steroidal anti-inflammatory drug used to ease pain and reduce inflammation in rheumatic conditions.
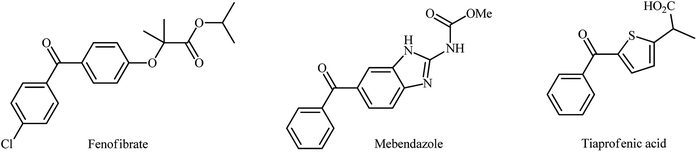 |
| Fig. 1 Selected examples of biaryl ketone containing drugs. | |
Biaryl ketones are also versatile building blocks for the synthesis of numerous complex chemical structures8 and photosensitizers in photochemical reactions.9 Because of their wide synthetic usefulness and biological importance, many efforts have been devoted to the development of their efficient synthetic methods. Traditionally, biaryl ketone systems have been synthesized via the Friedel–Crafts acylation of aromatic compounds (Fig. 2, path a),10 the Houben–Hoesch reaction of nitriles and arenes (Fig. 2, path b),11 the Fries rearrangement of aryl esters (Fig. 2, path c),12 and the reaction of Grignard reagents and acyl halides (Fig. 2, path d).13 However, most of these strategies, if not all, suffer from certain disadvantages. For example, since superacid is necessary for the Houben–Hoesch ketone synthesis, it suffers from compatibility issues with many functional groups. Similarly, Friedel–Crafts acylation utilizes stoichiometric amounts of Lewis acid and therefore leads to the generation of large amounts of waste, thus leading to poor atom economy. Moreover, the formation of ortho and para isomers with poor regioselectivity, their separation and accessing biaryl ketones with meta substitution are additional problems associated with this name reaction. In the case of Fries rearrangement, there are three major problems which limit its range of applications. First, the reaction requires drastic conditions and accordingly only esters with relatively stable acyl components can be used in this synthetic strategy. Second, poor yields are observed for substrates bearing deactivating or meta-directing groups. Third, owing to its poor regioselectivity, a mixture of ortho- and para-regioisomers is usually obtained.
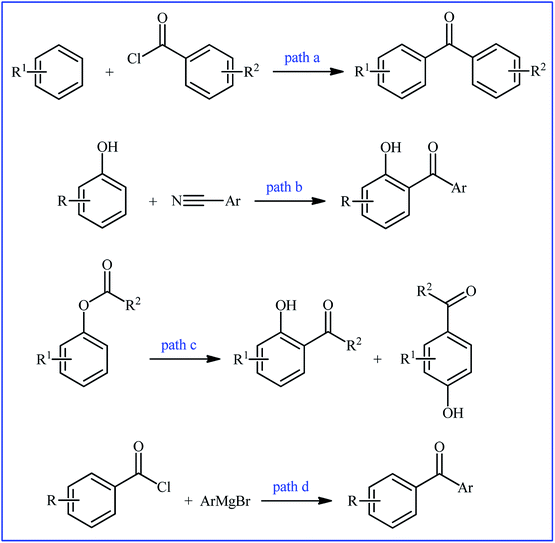 |
| Fig. 2 Traditional synthetic routes to biaryl ketones. | |
In the last few decades, transition-metal-catalyzed carbonylative cross-coupling reactions have emerged as one of the most straightforward and powerful methodologies for the preparation of titled compounds.14 Among the various carbonylative cross-coupling reactions, carbonylative Suzuki cross-coupling of readily accessible, non-toxic, and stable arylboronic acids with aryl halides has attracted highest attention of the synthetic chemistry community on the last years. However, the high catalyst and ligand loadings (more than 10 mol%), harsh reaction conditions, and the need for high pressure of CO remain important challenges in this synthetic strategy.15 In order to bypass these limitations, nanometal-based systems were recently used as highly efficient and reusable catalysts for this appealing reaction which due to their large ratio of surface area to mass and reactive morphologies allow for faster reactions under milder conditions even at very low catalyst loadings and in the absence of any ligand. Since several significant developments in this domain have taken place over the past few years, a comprehensive review on this fast-growing research arena seems to be timely. In connection with our recent works on modern cross-coupling reactions16 and application of nanocatalysts in organic synthesis,17 herein, we will attempt to highlight the most important discoveries and developments in the application of nanomaterial-based catalysts for carbonylative Suzuki cross-coupling reactions (Fig. 3), by hoping that it will serve as an inspiration to researchers in their future works.
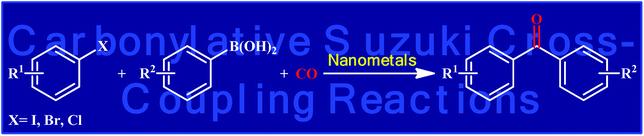 |
| Fig. 3 Nanometal-catalyzed carbonylative Suzuki cross-coupling of aryl halides and arylboronic acids. | |
The nanocatalysts generally play main role in all the chemistry fields.18–30 Here, we summarize the various nanocatalyst such as palladium, nickel, copper and bimetal in carbonylative Suzuki cross-coupling reactions.
2. Palladium nanoparticles-based catalysts
2.1. Single element Pd nanoparticles
One of the earliest reports deals with the use of single element palladium nanoparticles (PdNPs) as catalysts for carbonylative Suzuki coupling reactions can be found in a 2013 paper by Han and co-workers.31 In this report, a wide range of bi(hetero)aryl ketones 3 were synthesized in good to almost quantitative yields through the reaction of (hetero)aryl iodides 1 with (hetero)arylboronic acids 2 under carbon monoxide (CO) atmosphere using in situ generated PdNPs (2.6 nm) by decomposition of Pd(OAc)2 in PEG-400 at room temperature (Scheme 1). It is noteworthy that aryl iodides having either electron-donating or electron-withdrawing substituents were compatible with this synthetic strategy. In addition, the challenging double carbonylative coupling of diiodobenzenes (e.g., 1,2-diiodobenzene, 1,3-diiodobenzene, 1,4-diiodobenzene) was possible, affording diketone products in high yields. Moreover, the scope of boronic acids that underwent reaction was broad enough to include aryl, heteroaryl, and naphthylboronic acids. On the other hand, other aryl halides such as aryl bromides were not reactive, leading to negligible product yields. Of note, this procedure was successfully applied on the gram-scale with little effect on the yield. Additionally, the catalyst can be reused at least nine consecutive reaction runs without detrimental loss of catalytic performance (from 90% in the first run to 80% in the ninth run). According to the authors, the active catalyst is very likely to be homogeneous in nature as determined by the total inhibition of the reaction in the presence of stoichiometric amounts of CS2 and negative Hg(0) poisoning tests.
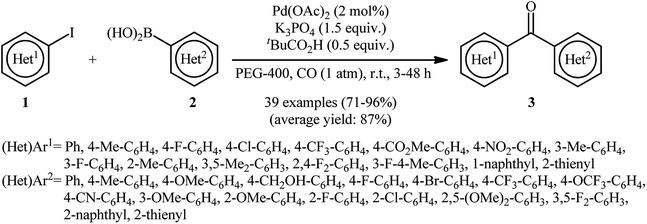 |
| Scheme 1 Han's synthesis of bi(hetero)aryl ketones 3. | |
With the objective of designing a more environmentally friendly procedure to biaryl ketones through the PdNPs-catalyzed carbonylative Suzuki coupling reaction, the group of Trzeciak were able to demonstrate that a series of unsymmetrical biaryl ketones 6 could be obtained via the three-component reaction of (hetero)aryl iodides 4, arylboronic acids 5, and CO (1 atm) in water employing Pd(1-BI)2Cl2 (1-BI = 1-butylimidazole) as a precursor of PdNPs and Na2CO3 as a cost-effective base (Scheme 2).32 The results demonstrated that the steric effects of the substituents on the aryl iodides had little impact on the facility of the reaction. However, the process strongly depended on the electronic nature of the substrates, with the best yields were obtained with electron-rich aryl iodides. Under similar conditions, the double carbonylation proceeded sluggishly, even with longer reaction times. Unfortunately, no comment was made by the authors regarding the recoverability and reusability of the catalyst.
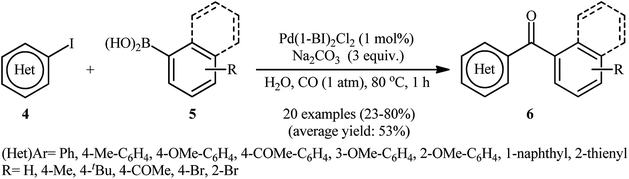 |
| Scheme 2 PdNPs-catalyzed carbonylative Suzuki coupling in water. | |
2.2. Magnetically separable Pd nanocatalysts
In recent years, magnetic nanoparticles have been extensively used as support materials to improve the recyclability and catalytic performance of the solid catalysts.33 Magnetic-nanoparticle-supported palladium catalysts are among the most widely used magnetically separable metal-nanocatalyst systems for various kinds of cross-coupling reactions.34
One of the earliest reports on the utilization of magnetically separable Pd nanocatalysts in the carbonylative Suzuki coupling reaction between aryl halides with arylboronic acids was published by Li and Ma along with their co-workers in 2014,35 who showed that the treatment of functionalized aryl iodides 7 with arylboronic acids 8 in the presence of a catalytic amount of palladium nanoparticles immobilized on thiol-modified hollow magnetic mesoporous silica spheres (HMMS-SH–PdII) and over-stoichiometric amounts of K2CO3 as a base under balloon pressure of CO in anisole, resulted in the formation of the corresponding biaryl ketones 9 in excellent yields along with small amounts of biaryl side products (Table 1, entry 1). Remarkably, the catalyst can be easily recovered from the reaction mixture by using an external magnetic field, washing with distilled water and ethanol, and air-dried, and then be reused at least five consecutive reaction runs without significant decline deactivation in its catalytic activity. The catalytic performance of HMMS-SH–Pd was also compared with MCM-41-2N–Pd, MCM-41-2P–Pd, ImmPd–IL and Pd/C catalysts in the reaction of 4-iodoanisole with phenylboronic acid. The results revealed the superior catalytic activity of the former. A mechanism that explains this transformation involves the initial formation of aryl–Pd–I complex A through the oxidative addition of aryl iodide 7 to HMMS-SH–Pd0 catalyst, which after migratory insertion of carbon monoxide affords aryl-CO–PdII–I complex B. Next, transmetalation of this intermediate with arylboronic acids 8 provides aryl-CO–PdII–aryl complex C. Finally, reductive elimination of this complex produces the target biaryl ketone 9 (Scheme 3). Subsequently, the same research group reported the preparation of a Fe3O4/PPy–PdII nanocomposite (PPy = polypyrrole) which exhibited similar catalytic activity to their HMMS-SH–PdII catalyst in the carbonylative coupling of the same set of aryl iodides and arylboronic acids (Table 1, entry 2).36 In a subsequent extension of the catalytic system of the methodology, it was shown that superparamagnetic Fe3O4@PANI–PdII nanocomposites (PANI = polyaniline) could effectively catalyze the titled reaction to give the desired biaryl ketones in yield up to 98% (Table 1, entry 3).37 Shortly afterwards, this innovative research team synthesized dopamine-functionalized magnetite nanoparticles (Fe3O4/DA) through a facile solvothermal method and then immobilized Pd0 and PdII onto it by refluxing with PdCl2 in EtOH in the presence or absence of NaBH4, respectively.38 Catalytic activities of the developed nanocatalysts (Fe3O4/DA–Pd0 and Fe3O4/DA–PdII) were thoroughly assessed using carbonylative cross-coupling reactions of various aryl iodides 7 with arylboronic acids 8 (Table 1, entries 4 and 5) which clearly revealed that PdII exhibited superior catalytic activity in comparison to Pd0. Low catalytic performance of the Pd0 is due to agglomeration of Pd nanoparticles which was seen via transmission electron microscopy (TEM). Drawing inspiration from these works, Qi and colleagues studied the similar carbonylative coupling of aryl iodides 7 and arylboronic acids 8 using Pd(II) complex immobilized on ethylenediamine-functionalized core–shell magnetic nanoparticles [Fe3O4@SiO2-2N–PdII] as a reusable catalyst in combination with K2CO3 in anisole (Table 1, entry 6).39 Just like previous works, the expected biaryl ketones 9 were obtained in good to excellent yields along with small amounts of biaryl side-products. Noteworthy, the catalyst was also successfully utilized in the aerobic oxidation of a library of alcohols to the corresponding aldehydes.
Table 1 Three-component coupling between aryl iodides 7, arylboronic acids 8, and CO catalyzed by magnetically separable Pd nanocatalysts
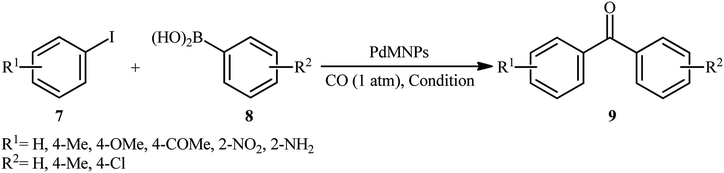
|
Entry |
Pd nanocatalyst |
Conditions |
Number of examples |
Yield (%) |
Ref. |
Range |
Average |
1 |
HMMS-SH–PdII |
Anisole, K2CO3, 80 °C, 7–12 h |
18 |
85–99 |
92 |
22 |
2 |
Fe3O4/PPy–PdII |
Anisole, K2CO3, 80 °C, 6–12 h |
18 |
80–90 |
90 |
23 |
3 |
Fe3O4/PANI–PdII |
Anisole, K2CO3, 80 °C, 6–12 h |
18 |
87–98 |
94 |
24 |
4 |
Fe3O4/DA–Pd0 |
Anisole, K2CO3, 80 °C, 6–8 h |
15 |
79–96 |
90 |
25 |
5 |
Fe3O4/DA–PdII |
Anisole, K2CO3, 80 °C, 6–8 h |
15 |
62–85 |
75 |
25 |
6 |
Fe3O4@SiO2-2N–PdII |
Anisole, K2CO3, 80 °C, 6–12 h |
18 |
72–99 |
90 |
26 |
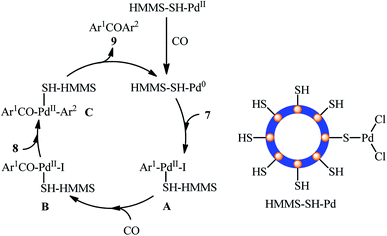 |
| Scheme 3 Plausible reaction mechanism for the HMMS-SH–Pd(II)-catalyzed carbonylative Suzuki coupling of aryl iodides 7 with arylboronic acids 8. | |
Recently, the Cai laboratory designed and synthesized a novel bidentate phosphino-functionalized magnetic nanoparticle-immobilized palladium(II) complex (Fe3O4@SiO2-2P–PdCl2) through a simple procedure via condensation of easily accessible silica-coated Fe3O4 (Fe3O4@SiO2) with N,N-bis((diphenylphosphino)methyl)-3-(triethoxysilyl)propan-1-amine in refluxing toluene followed by reaction with PdCl2 in refluxing acetone for 72 h (Fig. 4).40 The prepared nanocomposite system was applied as an efficient catalyst for high yielding synthesis of a wide variety of bi(hetero)aryl ketones 12 through carbonylative Suzuki coupling of (hetero)aryl iodides 10 with (hetero)arylboronic acids 11 using formic acid (HCO2H) as the carbon monoxide source and dicyclohexylcarbodiimide (DCC) as dehydrating reagent (Scheme 4). The results demonstrated that aryl iodides possessing electron-withdrawing groups afforded relatively higher yields compared to the electron-rich aryl iodides and the relative reaction rates of (hetero)arylboronic acids followed the order: electron-rich > electron-poor > heteroaryl. Unfortunately, replacing aryl iodides with aryl bromides led to no desired product even at higher temperatures. The recycling test established that the catalyst could be recovered by simply applying an external magnet and reused for ten consecutive runs without any loss of activity.
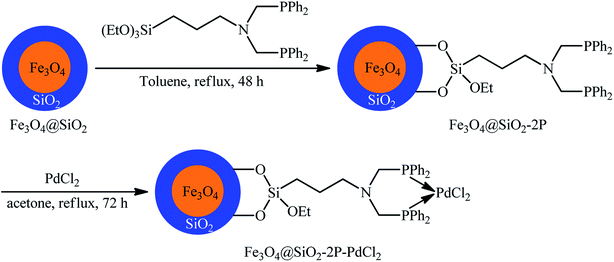 |
| Fig. 4 Schematic diagram showing the formation of Fe3O4@SiO2-2P–PdCl2 nanocomposite. | |
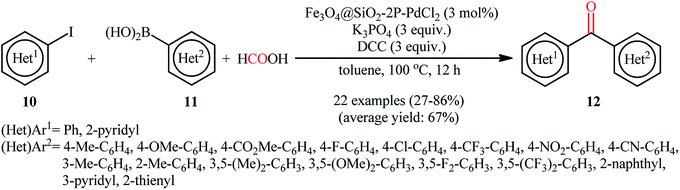 |
| Scheme 4 Fe3O4@SiO2-2P–PdCl2-catalyzed synthesis of biaryl ketones 12 via carbonylative Suzuki coupling of aryl iodides 10 with arylboronic acids 11 using HCO2H as the CO source. | |
2.3. Metal–organic frameworks supported Pd nanocatalysts
During the recent years, metal–organic frameworks (MOFs) have attracted extensive attention as supports for metal nanoparticles due to their huge surface area, large porosity, recyclability, and tunable functionality.41,42 In this new class of heterogeneous catalysts, metal–organic framework-supported Pd nanoparticles are becoming the most popular one for their excellent catalytic performance and reusable property.43 In this part, attention focuses on use of MOF-supported Pd NPs as catalysts in Suzuki carbonylative reactions.
In 2014, Seayad and colleagues reported a ZIF-8 (zeolitic imidazolate framework) supported palladium nanoparticle (Pd/ZIF-8) catalyst for carbonylative Ullmann-type C–N bond formation couplings.44 The catalytic activity of synthesized Pd/ZIF-8 nanocatalyst was also explored for carbonylative coupling of iodobenzene with p-methoxyphenylboronic acid using Et3N as a base in refluxing toluene under CO atmosphere and achieved the corresponding biaryl ketone in 78% isolated yield. Although, only one example was disclosed, it could be an inspiration for further researchers. Based on the above catalyst, the Trzeciak research team developed an improved metal–organic framework (MOF)-supported Pd NPs catalyst {Pd@[Ni(H2BDP-SO3)2]} by using a facile impregnation process with solvent in situ reduction method.45 The prepared material exhibits a very large surface area of over 583 m2 g−1 and uniform dispersion and size (4–8 nm) distribution of 5.6 wt% Pd NPs. Various iodoanisole 13 and arylboronic acids 14 were studied to obtain moderate yields of products 15 in the presence of catalytic amounts of the prepared material and 3 equiv. of K2CO3 under 10 atm pressure of CO at 105 °C (Scheme 5). Interestingly, the outcome of this reaction was strongly dependent on the substitution pattern of the aryl halides. The decreasing order of reactivity for iodoanisoles is 3-iodoanisole > 4-iodoanisole ≥ 2-iodoanisole. The authors explained this observation by reduced pore availability of Pd@[Ni(H2BDP-SO3)2] for a 3-iodoanisole molecule which facilitate the reaction on the surface of Pd@[Ni(H2BDP-SO3)2].
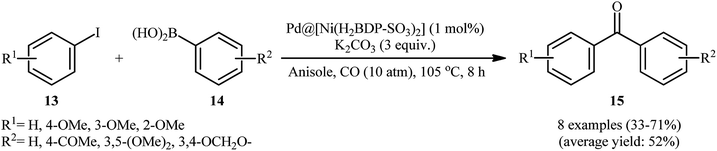 |
| Scheme 5 Pd@[Ni(H2BDP-SO3)2]-catalyzed synthesis of biaryl ketones 15 developed by Seayad. | |
2.4. Graphene oxide supported Pd nanocatalysts
After pioneering work by Yinghuai and co-workers on carbonylative cross-coupling of iodobenzene and phenylboronic acid utilizing graphene oxide (GO)-supported PdNPs around 4.9 nm in size as catalyst and K2CO3 as a base in anhydrous anisole,46 the first general report of the synthesis of biaryl ketones via graphene oxide-stabilized PdNPs-catalyzed Suzuki carbonylative cross-coupling reactions was published in 2019 by Bhanage et al.47 In this investigation, amine-functionalized graphene oxide-stabilized Pd nanoparticles (Pd@APGO) was synthesized by a simple sonication treatment of as-prepared GO with 3-aminopropyltrimethoxysilane (APTMS) in EtOH and then treatment with PdCl2 aqueous solution in the presence of NaBH4. As shown in TEM images (Fig. 5), the produced spherical shaped Pd NPs are small and crystalline in nanometric size (ranges from 4 to 10 nm) and are well-distributed on the surface of the amine-functionalized GO. The composite nano-Pd@APGO was found to be a significantly efficient and recyclable catalyst for three-component reaction between (hetero)aryl iodides 16, (hetero)arylboronic acids 17, and CO at 2 atm pressure. As shown in Scheme 6, cross-coupling reaction leads to prepare nine bi(hetero)aryl ketones 18 with medium to excellent yields within 8 h. The comparison of the catalytic activity of Pd@APGO with a variety of supported catalysts such as Pd@GO, Pd@AP–GO, Pd0–AmPMCF, and ASNTs@Pd established its superior comparability with them in terms of product yield.
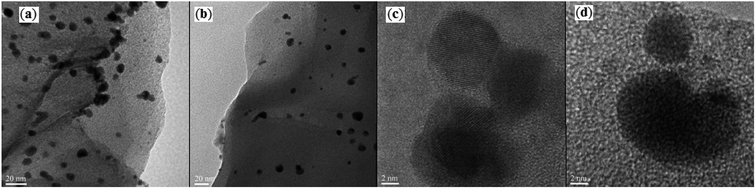 |
| Fig. 5 (a and b) TEM images of the synthesized Pd@APGO catalyst and (c and d) closure view of PdNPs. | |
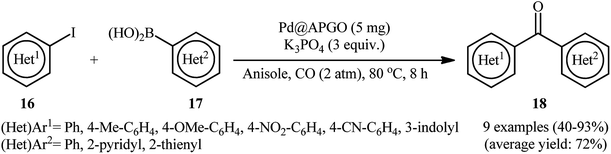 |
| Scheme 6 Pd@APGO-catalyzed carbonylative coupling of (hetero)aryl iodides 16 with (hetero)arylboronic acids 17 reported by Bhanage. | |
Concurrently, the group of Trzeciak investigated the preparation of Pd NPs supported on titanium-dioxide-modified graphene oxide (Pd/GO–TiO2) through immobilizing Pd(II) on to GO–TiO2 surface using precipitation deposition method.48 TEM and ICPMS (inductively coupled plasma mass spectrometry) experiments revealed the presence of Pd NPs of different diameters (4–89 nm) and a loading of 1.21 weight% on the support. The obtained composite exhibited good activity in the carbonylative Suzuki coupling reactions of electron rich aryl iodides 19 with arylboronic acids 20 (Scheme 7). The reactions were carried out in anisole at 100 °C under atmospheric pressure of CO and provided moderate to high yields of the desired coupling products 21.
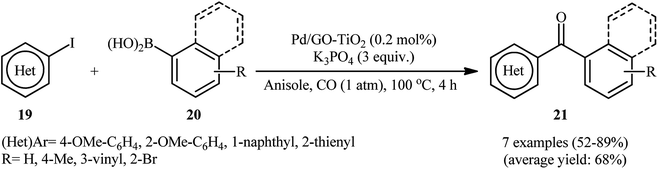 |
| Scheme 7 Carbonylative Suzuki coupling reactions of electron rich aryl iodides 19 with arylboronic acids 20 catalyzed by Pd/GO–TiO2. | |
2.5. Miscellaneous
In 2015, Liu and co-workers reported the preparation of cross linked polymeric materials based on poly(ionic liquid)s [P(DVBX–NDIIL1)] 23 by azobisisobutyronitrile (AIBN)-initiated copolymerization of dication imidazole ionic liquid (NDIIL) 22 with divinylbenzene (DVB) in different DVB/NDIIL molar ratios (Scheme 8).49 The obtained supported ionic liquid-like phases were then treated with Na2PdCl4 to immobilize the PdII species. Next, the materials were reacted with NaBH4, which caused reduction of PdII to Pd0. To evaluate the catalytic activity of the prepared catalysts in carbonylative Suzuki coupling reactions, iodobenzene and phenyl boronic acid were chosen as the model substrates. Among these four catalysts (i.e., [P(DVB0.5–NDIIL1)–Pd], [P(DVB1–NDIIL1)–Pd], [P(DVB3–NDIIL1)–Pd], [P(DVB6–NDIIL1)–Pd]); [P(DVB1–NDIIL1)–Pd] was found to be more effective, which gave a better yield of benzophenone product. The optimization of the reaction conditions also indicated that K2CO3 was more effective than other bases (e.g., Na2CO3, Cs2CO3, Et3N) and compared to other solvents (e.g., anisole, toluene, MeCN, NMF, THF, H2O), toluene was the best choice for the conversion. Under the optimized conditions, the reaction tolerated a variety of functionalized aryl iodides 24 and aryl boronic acids 25 and provided the expected biaryl ketones 26 in good to high yields (Scheme 9). However, when aryl iodide substrates were replaced with aryl bromides and chlorides, the reaction did not afford the target products. The authors explained this fact by high dissociation energy of the C–Cl or C–Br bonds compared with C–I bond (402, 339, and 272 kJ mol−1 for PhCl, PhBr, and PhI, respectively, at 298 K). Of note, hot filtration and mercury poisoning tests proved that the palladium species leached into the solvent during the reaction and returned to the polymers after the reaction was completed. Recycling tests indicated that the catalyst can be reused in up to five consecutive trials, although with slightly decreased yields, which was attributed to leaching of Pd and slight aggregation of the Pd nanoparticles.
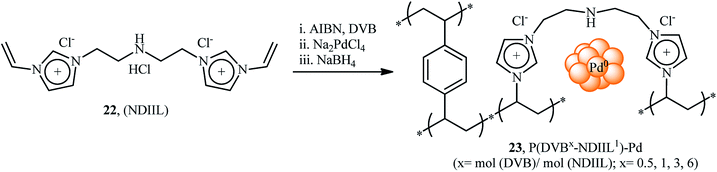 |
| Scheme 8 Synthesis of supported ionic liquid-like phases immobilized Pd nanoparticles. | |
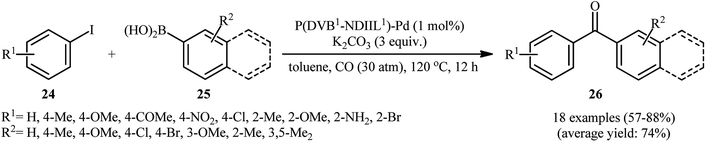 |
| Scheme 9 [P(DVB1–NDIIL1)–Pd]-catalyzed synthesis of biaryl ketones 26. | |
At the same year, Guo's research group disclosed the usefulness of silicon carbide supported Pd nanoparticles (PdNPs/SiC) as the catalyst for carbonylative Suzuki coupling reaction of aryl halides with arylboronic acids.50 Thus, in the presence of a combination of PdNPs/SiC (3 wt%) and K2CO3 (3 equiv.) in anisole, three component coupling reaction of aryl halides 27 (aryl iodides, aryl bromides, and aryl chlorides), arylboronic acids 28, and CO furnished the corresponding biaryl ketones 29 in fair to excellent yields, ranging from 21% to 96% (Scheme 10a). When compared with other aryl halides, aryl iodides were found to be the best halide for this carbonylative reaction. While phenylboronic acid reacted with bromobenzene and chlorobenzene, giving the desired benzophenone in 41 and 21% yields, respectively, the reaction of phenylboronic acid with iodobenzene led to the coupling product in 89% yield. The recyclability of the catalyst towards the coupling of iodobenzene and phenylboronic acid was examined, showing a little but noticeable loss in catalytic activity (from 90% in the first cycle to 76% in the fifth cycle). Furthermore, the leaching tests of the active Pd species, as performed by inductively coupled plasma mass spectrometry (ICPMS) analysis revealed that the Pd loading of the Pd/SiC catalyst decreased after each cycle. Shortly afterwards, Burri and co-workers improved the efficiency of this reaction in the terms of reaction yield and pressure of CO by switching the solvent to 1,4-dioxane (Scheme 10b).51 Importantly, 1,4-dioxane not only acts as a solvating medium, but also as a promoter for this reaction. Mechanistically, the reaction of 1,4-dioxane with arylboronic acids 31 under this condition provided highly reactive aryl dioxaborolanes which coupled with aryl iodides 30 and transform into biaryl ketones 32 in the presence of CO. It should be mentioned that previously the group of Trzeciak disclosed the usefulness of 1,4-dioxane as the reaction medium for PdNPs-catalyzed carbonylative Suzuki cross-coupling reactions.52
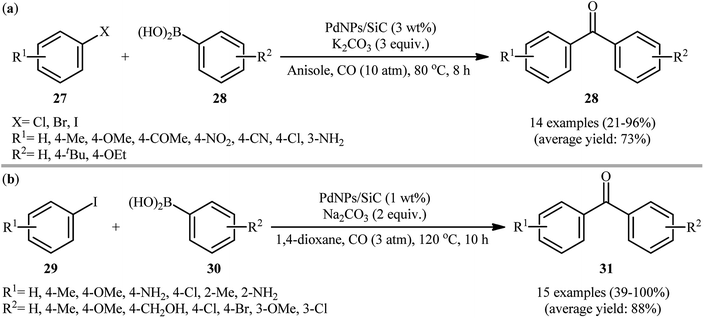 |
| Scheme 10 (a) Guo's synthesis of biaryl ketones 28; (b) Burri's synthesis of biaryl ketones 31. | |
In 2016, Polshettiwar and Bhanage along with their colleagues introduced palladium nanoparticles supported on polyethylenimine (PEI) functionalized fibrous silica (KCC-1), named KCC-1-PEI/Pd as an efficient catalyst for the carbonylative Suzuki cross-coupling of aryl halides.53 The catalyst was prepared by covalent anchoring of PEI on the KCC-1 surface with the help of glycidoxypropyltrimethoxysilane (GTMS) followed by incorporation of Pd through reaction with sodium tetrachloropalladate salt and finally reduction of Pd(II) by NaBH4 to Pd(0). Various biaryl ketones 34 were successfully synthesized in moderate yields from the corresponding aryl iodides 32 and arylboronic acids 33 employing this heterogeneous catalyst (Scheme 11a). High turnover number (TON), high turnover frequency (TOF), broad substrate scope, and reusability of the catalyst were the merits, claimed for this synthetic strategy. Recently, a related carbonylative coupling of aryl halides with arylboronic acids using carbon monoxide as the C1 source was reported by Lei and co-workers.54 They showed that in the presence of only 0.25 mol% of palladium nanoparticles supported on phosphorus-doped porous organic polymer (Pd@POP-Ph3P) as the catalyst and 2 equiv. of K2CO3 as a base, reaction of a variety of functionalized aryl iodides 35 with a wide range of arylboronic acids 36 under a CO atmosphere furnished the respective biaryl ketones 37 in high to almost quantitative yields (Scheme 11b). This protocol tolerated a number of important functional groups, including fluoro, chloro, bromo, methoxy, nitro, amino, and ketone functionalities, and promised its potential applications in the further modifications of the prepared coupling products. For comparison, the catalytic performances of different palladium catalysts [e.g., PdCl2(PPh3)2, Pd/C, Pd(OAc)2/3V-PPh3, Pd@KAPs (Ph-PPh3)] were also tested in the reaction of 4-iodoanisole with phenylboronic acid. The results proved superior catalytic activity of the developed catalyst. Noteworthy, aryl bromides were also successfully applied as electrophilic coupling partners in this procedure. However, a higher reaction temperature (120 °C) and higher loadings of the catalyst (2.5 mol%) were required.
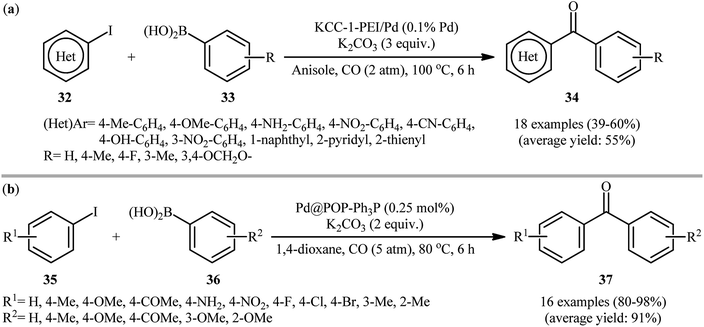 |
| Scheme 11 (a) [KCC-1-PEI/Pd]-catalyzed carbonylative Suzuki cross-coupling of (hetero)aryl iodides 32 and arylboronic acids 33; (b) carbonylative coupling of aryl iodides 35 and arylboronic acids 36 catalyzed by Pd@POP-Ph3P. | |
3. Nickel nanoparticles-based catalysts
Nickel is located in group 10 and just above palladium in the periodic table and compared with palladium is much cheaper and safer. Therefore, it is reasonable to investigate the catalytic ability of this earth abundant metal on the carbonylative Suzuki cross-coupling reactions. The first and only example of NiNPs-catalyzed carbonylative Suzuki reactions of aryl halides with arylboronic acids was described by Han and colleagues in 2014.55 The authors reported that various electron-poor and -rich (hetero)aryl iodides 38 bearing o/m/p substituents could undergo carbonylative coupling with a divers electron-rich and electron-poor (hetero)arylboronic acids 39 under ambient pressure of carbon monoxide using in situ generated NiNPs by decomposition of NiCl2 in PEG-400 at 80 °C to give the corresponding bi(hetero)aryl ketones 40 in good to excellent yields (Scheme 12). However, 2-iodopyrazine was not compatible with the reaction condition employed. Notably, compared with pre-prepared nanoparticles the in situ generated nanocatalysis exhibited higher catalytic activity for this reaction. Some important information of this synthetic strategy are listed below: (i) the use of pivalic acid (PivOH) as an additive was crucial to suppress the competitive direct Suzuki coupling reactions; (ii) electronic and steric nature of both substrates had a little influence on the efficiency of the reactions; (iii) double carbonylation of diiodobenzenes also worked well and delivered the desired diketone products in good yields; and (iv) 4-bromobenzaldehyde did not work well in the reaction and therefore no other aryl bromides were examined in the protocol.
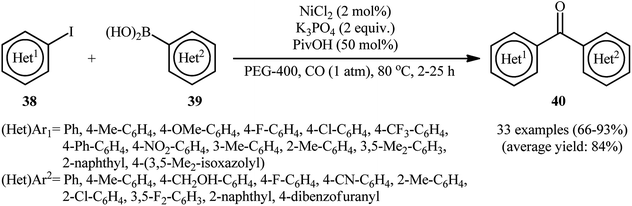 |
| Scheme 12 NiNPs-catalyzed synthesis of biaryl ketones 40 from the reaction of aryl iodides 38, arylboronic acids 39, and CO. | |
4. Copper nanoparticles-based catalysts
The copper nanoparticles catalyzed carbonylative Suzuki reactions has been scarcely investigated; in fact, only one study to date was published on such a reaction. In this report, thirty-four bi(hetero)aryl ketone scaffolds 43 were synthesized in good to excellent yields (71–95%) through the three-component reaction between (hetero)aryl iodides 41, (hetero)arylboronic acids 42, and atmospheric CO employing elemental copper nanoparticles (CuNPs) as a catalyst, PivOH as an additive, and combination of K3PO4/KF as a base mixture in PEG-400 as a green solvent (Scheme 13).56 The catalyst was also demonstrated to be very efficient in double carbonylative coupling of diiodobenzenes. To study the recoverability and reusability of the catalyst, carbonylative coupling of 4-iodonitrobenzene with phenylboronic acid was considered as a model reaction. It was found that the catalyst could be easily recycled by simple extraction from the reaction mixture and reused up to ten consecutive cycles without detrimental loss of catalytic activity (from 94% in the first run to 87% in the tenth run).
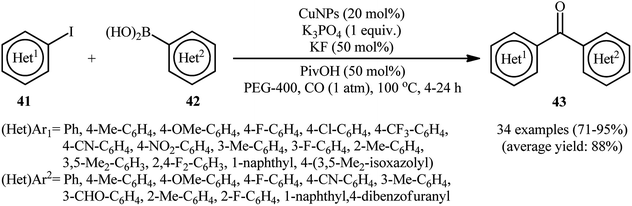 |
| Scheme 13 Carbonylative coupling of (hetero)aryl iodides 41 and (hetero)arylboronic acids 42 catalyzed by elemental copper nanoparticles. | |
5. Bimetalic nanoparticles
Bimetallic nanoparticles, composed of two distinct metallic elements, not only show the combination of the related properties of their monometallic counterparts but also exhibit new and improved properties due to the synergistic effect between the two metals.57 During the past few years, bimetallic nanoparticles have attracted a broad research interest in catalysis community due to their tremendous catalytic activity in many organic reactions.58
In 2017, Liu and Zhang along with their co-workers prepared PdNP–ZnO nanowire arrays by putting the ZnO@Zn nanowire arrays into an aqueous solution of Na2PdCl4 at room temperature.59 The elemental mapping analysis showed that in situ generated PdNPs is localized at the outer layer of the as-prepared ZnO hybrid nanowire and TEM image of the synthesized hybrid nanowire arrays indicated that the average size of the PdNPs was about 2–4 nm (Fig. 6). The catalytic activity of the ensuing nanoparticles was tested for carbonylative coupling of various (hetero)aryl iodides 44 and arylboronic acids 45 using Mo(CO)6 as an efficient solid carbonylating agent under alkaline conditions at 80 °C, providing high to excellent yields of the target bi(hetero)aryl ketones 46 (Scheme 14). Remarkably, the catalyst was also found to be highly efficient for reduction of nitro aryl compounds to the corresponding anilines as well as for the synthesis of biaryl from the respective aryl halides and arylboronic acids. Interestingly, the catalyst can be readily separated from the reaction mixture by centrifugation and reused over ten reaction cycles with negligible loss of catalytic activity. The authors suggested that the persistence of the highly catalytic performance of the collected catalysts is due to a combination of marginable catalyst loss and PdNP coalescence in the course of catalytic reaction.
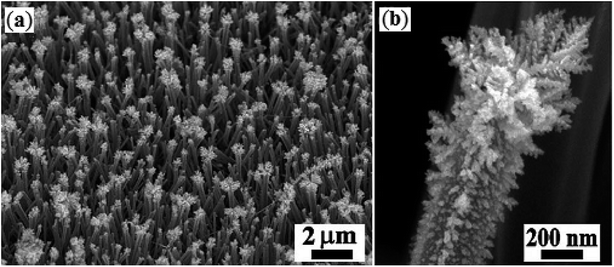 |
| Fig. 6 SEM image of (a) the as-prepared PdNP–ZnO hybrid nanowire arrays; (b) a single hybrid PdNP–ZnO nanowire in the array. | |
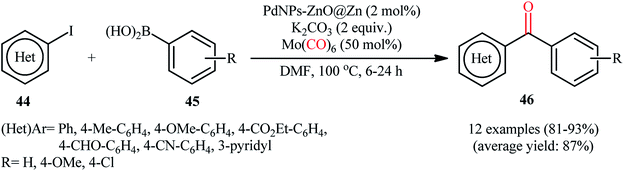 |
| Scheme 14 PdNP–ZnO nanowire array-catalyzed carbonylative Suzuki cross-coupling reaction of (hetero)aryl halides 44 and arylboronic acids 45 using Mo(CO)6 as the carbonyl source. | |
Concurrently, Xu's research group introduced bimetallic platinum–palladium nanodendrites (Pd–Pt NDs) as an efficient heterogeneous catalyst for carbonylative Suzuki cross-coupling reaction of (hetero)aryl halides 47 and (hetero)arylboronic acids 48 using CO as the carbonyl source.60 The reactions were performed in the presence of 2 equiv. of NaHCO3 as a base in DMF under ambient pressure, tolerated a number of sensitive functional groups (e.g., NH2, NO2, CN, CHO, CO2Et, OMe, F, CF3), and provided the target bi(hetero)aryl ketones 49 in relatively poor to excellent yields. As shown in Scheme 15, besides aryl iodides, aryl bromides and aryl chlorides also could be applied as coupling partners in this transformation. The results indicated that the reactivity of aryl bromides was similar to the corresponding aryl iodides under these conditions. However, aryl chlorides were extremely less reactive and generally resulted in low yields of carbonylated products. Noteworthy, the catalyst showed five times reusability without significant of activity.
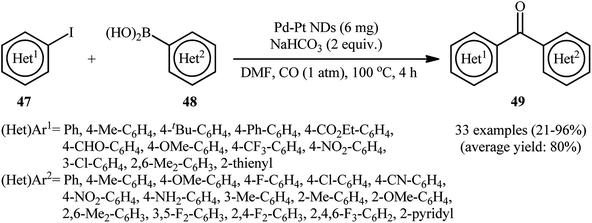 |
| Scheme 15 Xu's synthesis of bi(hetero)aryl ketones 49. | |
6. Conclusion
This review has outlined recent advances and developments in the field of metal nanoparticles catalyzed carbonylative Suzuki cross-coupling reactions of arylboronic acids with aryl halides and shown how efficient this new and fast-growing research field is in terms of high yielding biaryl ketone products and recovery of catalysts. Despite the extraordinary accomplishments over the past few years in this attractive research arena, many significant challenges still remain to be overcome: (i) the majority of reported examples in the field have been performed under toxic CO atmosphere. From a green chemistry point of view, there is a need for replacement of CO with less toxic source of carbonylating agents such as carbon dioxide and methyl formate; (ii) the scope of the electrophilic coupling partners was largely limited to expensive aryl iodides, thus expanding of the substrate scope of this transformation to less expensive aryl bromides and chlorides are necessary; and (iii) almost all of nanocatalysts that have been reported to be effective for carbonylative Suzuki coupling are invariably based on expensive and toxic palladium-based systems. Given cost and sustainability concerns, the development of related processes employing less toxic and inexpensive metal catalysts would be highly desirable.
Conflicts of interest
There are no conflicts to declare.
References
- R. Heel, R. Brogden, T. Speight and G. Avery, Drugs, 1977, 14, 349–366 CrossRef CAS.
- J. Najib, Clin. Ther., 2002, 24, 2022–2050 CrossRef CAS.
- A. E. Guerini, L. Triggiani, M. Maddalo, M. L. Bonù, F. Frassine, A. Baiguini, A. Alghisi, D. Tomasini, P. Borghetti and N. Pasinetti, Cancers, 2019, 11, 1284 CrossRef CAS.
- E. M. Sorkin and R. Brogden, Drugs, 1985, 29, 208–235 CrossRef CAS.
- D. D. Truong, Clin. Interventions Aging, 2009, 4, 109–113 CrossRef CAS.
-
(a) T. G. Kantor, Pharmacotherapy, 1986, 6, 93–102 CrossRef CAS;
(b) S. Shafiei and S. Davaran, Chem. Rev. Lett., 2020, 3, 19–22 Search PubMed;
(c) S. Majedi and S. Majedi, J. Chem. Lett., 2020, 1, 2–8 Search PubMed;
(d) F. Nareetsile, J. T. P. Matshwele, S. Ndlovu and M. Ngaski, Chem. Rev. Lett., 2020, 3, 140–160 Search PubMed.
- S. Q. Wang, Y. Balagula and U. Osterwalder, Dermatol. Ther., 2010, 23, 31–47 CrossRef.
-
(a) E. Kobayashi, A. Kishi and H. Togo, Eur. J. Org. Chem., 2019, 7335–7347 CrossRef CAS;
(b) N. Kise, Y. Hamada and T. Sakurai, Org. Lett., 2014, 16, 3348–3351 CrossRef CAS;
(c) B. Gao, Y. Zhao, M. Hu, C. Ni and J. Hu, Chem.–Eur. J., 2014, 20, 7803–7810 CrossRef CAS;
(d) B. Gao, J. Hu, Y. Zhao and J. Hu, Tetrahedron Lett., 2015, 56, 4180–4183 CrossRef CAS.
- J. Perez-Prieto, R. Galian and M. Miranda, Mini-Rev. Org. Chem., 2006, 3, 117–135 CrossRef CAS.
- G. Sartori and R. Maggi, Chem. Rev., 2006, 106, 1077–1104 CrossRef CAS.
-
(a) Y. Sato, M. Yato, T. Ohwada, S. Saito and K. Shudo, J. Am. Chem. Soc., 1995, 117, 3037–3043 CrossRef CAS;
(b) C. Zhou and R. C. Larock, J. Am. Chem. Soc., 2004, 126, 2302–2303 CrossRef CAS.
- K. J. Singh and D. B. Collum, J. Am. Chem. Soc., 2006, 128, 13753–13760 CrossRef CAS.
-
(a) C. Malanga, L. A. Aronica and L. Lardicci, Tetrahedron Lett., 1995, 36, 9185–9188 CrossRef CAS;
(b) C.-T. Zhang, R. Zhu, Z. Wang, B. Ma, A. Zajac, M. Smiglak, C.-N. Xia, S. L. Castle and W.-L. Wang, RSC Adv., 2019, 9, 2199–2204 RSC.
- J.-J. Brunet and R. Chauvin, Chem. Soc. Rev., 1995, 24, 89–95 RSC.
-
(a) T. Ishiyama, H. Kizaki, N. Miyaura and A. Suzuki, Tetrahedron Lett., 1993, 34, 7595–7598 CrossRef CAS;
(b) E. Maerten, F. Hassouna, S. Couve-Bonnaire, A. Mortreux, J.-F. Carpentier and Y. Castanet, Synlett, 2003, 2003, 1874–1876 Search PubMed;
(c) D. Mingji, B. Liang, C. Wang, Z. You, J. Xiang, G. Dong, J. Chen and Z. Yang, Adv. Synth. Catal., 2004, 346, 1669–1673 CrossRef;
(d) K. M. Bjerglund, T. Skrydstrup and G. A. Molande, Org. Lett., 2014, 16, 1888–1891 CrossRef CAS.
-
(a) A. Hosseinian, S. Farshbaf, L. Z. Fekri, M. Nikpassand and E. Vessally, Top. Curr. Chem., 2018, 376, 23 CrossRef;
(b) A. Hosseinian, S. Ahmadi, F. A. H. Nasab, R. Mohammadi and E. Vessally, Top. Curr. Chem., 2018, 376, 39 CrossRef;
(c) A. Hosseinian, F. A. H. Nasab, S. Ahmadi, Z. Rahmani and E. Vessally, RSC Adv., 2018, 8, 26383–26398 RSC;
(d) A. Hosseinian, R. Mohammadi, S. Ahmadi, A. Monfared and Z. Rahmani, RSC Adv., 2018, 8, 33828–33844 RSC;
(e) W. Peng, E. Vessally, S. Arshadi, A. Monfared, A. Hosseinian and L. Edjlali, Top. Curr. Chem., 2019, 377, 20 CrossRef;
(f) A. Monfared, S. Ebrahimiasl, M. Babazadeh, S. Arshadi and E. Vessally, J. Fluorine Chem., 2019, 220, 24–34 CrossRef CAS;
(g) S. Arshadi, S. Ebrahimiasl, A. Hosseinian, A. Monfared and E. Vessally, RSC Adv., 2019, 9, 8964–8976 RSC;
(h) M. Hamzeloo, A. Hosseinian, S. Ebrahimiasl, A. Monfared and E. Vessally, J. Fluorine Chem., 2019, 224, 52–60 CrossRef;
(i) S. Arshadi, A. Banaei, A. Monfared, S. Ebrahimiasl and A. Hosseinian, RSC Adv., 2019, 9, 17101–17118 RSC;
(j) A. Hosseinian, S. Arshadi, S. Sarhandi, A. Monfared and E. Vessally, J. Sulfur Chem., 2019, 40, 289–311 CrossRef CAS;
(k) Y. Yang, D. Zhang and E. Vessally, Top. Curr. Chem., 2020, 378, 37 CrossRef CAS.
-
(a) E. Vessally, M. Babazadeh, A. Hosseinian, S. Arshadi and L. Edjlali, J. CO2 Util., 2017, 21, 491–502 CrossRef CAS;
(b) K. Nejati, S. Ahmadi, M. Nikpassand, P. D. K. Nezhad and E. Vessally, RSC Adv., 2018, 8, 19125–19143 RSC;
(c) K. Didehban, E. Vessally, A. Hosseinian, L. Edjlali and E. S. Khosroshahi, RSC Adv., 2018, 8, 291–301 RSC;
(d) A. Hosseinian, S. Ahmadi, A. Monfared, P. D. Nezhad and E. Vessally, Curr. Org. Chem., 2018, 22, 1862–1874 CrossRef CAS;
(e) E. Vessally, K. Didehban, R. Mohammadi, A. Hosseinian and M. Babazadeh, J. Sulfur Chem., 2018, 39, 332–349 CrossRef CAS;
(f) A. Monfared, R. Mohammadi, S. Ahmadi, M. Nikpassand and A. Hosseinian, RSC Adv., 2019, 9, 3185–3202 RSC;
(g) J. Wang, P. Su, S. Abdolmohammadi and E. Vessally, RSC Adv., 2019, 9, 41684–41702 RSC;
(h) S. Ebrahimiasl, F. Behmagham, S. Abdolmohammadi, R. N. Kojabad and E. Vessally, Curr. Org. Chem., 2019, 23, 2489–2503 CrossRef CAS;
(i) S. Mohammadi, M. Musavi, F. Abdollahzadeh, S. Babadoust and A. Hosseinian, Chem. Rev. Lett., 2018, 1, 49–55 Search PubMed;
(j) S. Shahidi, P. Farajzadeh, P. Ojaghloo, I. Karbakhshzadeh and A. Hosseinian, Chem. Rev. Lett., 2018, 1, 37–44 Search PubMed;
(k) S. Sarhandi, M. Daghagheleh, M. Vali, R. Moghadami and E. Vessally, Chem. Rev. Lett., 2018, 1, 9–15 Search PubMed;
(l) L. Sreerama, E. Vessally and F. Behmagham, J. Chem. Lett., 2020, 1, 9–18 Search PubMed;
(m) M. Daghagheleh, M. Vali, Z. Rahmani, S. Sarhandi and E. Vessally, Chem. Rev. Lett., 2018, 1, 23–30 Search PubMed;
(n) S. Farshbaf, L. Sreerama, T. Khodayari and E. Vessally, Chem. Rev. Lett., 2018, 1, 56–67 Search PubMed;
(o) S. Majedi, L. Sreerama, E. Vessally and F. Behmagham, J. Chem. Lett., 2020, 1, 25–31 Search PubMed;
(p) S. Majedi, S. Majedi and F. Behmagham, Chem. Rev. Lett., 2019, 2, 187–192 Search PubMed;
(q) E. A. Mahmood, B. Azizi and S. Majedi, Chem. Rev. Lett., 2020, 3, 2–8 Search PubMed;
(r) S. Shahidi, P. Farajzadeh, P. Ojaghloo, A. Karbakhshzadeh and A. Hosseinian, Chem. Rev. Lett., 2018, 1, 37–44 Search PubMed;
(s) M. R. J. Sarvestani, N. Mert, P. Charehjou and E. Vessally, J. Chem. Lett., 2020, 1, 93–102 Search PubMed.
- S. Chen, M. K. Hassanzadeh-Aghdam and R. Ansari, J. Alloys Compd., 2018, 767, 632–641 CrossRef CAS.
- H. Yu, W. Dai, G. Qian, X. Gong, D. Zhou, X. Li and X. Zhou, Nanomaterials, 2020, 10, 897 CrossRef CAS.
- L. He, J. Liu, Y. Liu, B. Cui, B. Hu, M. Wang, K. Tian, Y. Song, S. Wu, Z. Zhang, Z. Peng and M. Du, Appl. Catal., B, 2019, 248, 366–379 CrossRef CAS.
- Q. Liao, W. Wei, H. Zuo, X. Li, Z. Yang, S. Xiao and G. Wu, Compos. Interfaces, 2020, 1–13 CAS.
- H. Guo, K. Qian, A. Cai, J. Tang and J. Liu, Sens. Actuators, B, 2019, 300, 126846 CrossRef CAS.
- J. Liu, C. Wang, H. Sun, H. Wang, F. Rong, L. He, Y. Lou, S. Zhang, Z. Zhang and M. Du, Appl. Catal., B, 2020, 279, 119407 CrossRef CAS.
- Y. Liu, Q. Zhang, M. Xu, H. Yuan, Y. Chen, J. Zhang, K. Luo, J. Zhang and B. You, Appl. Surf. Sci., 2019, 476, 632–640 CrossRef CAS.
- X. Wang, J. Wang, X. Sun, S. Wei, L. Cui, W. Yang and J. Liu, Nano Res., 2018, 11, 988–996 CrossRef CAS.
- X. Li, R. Zhang, X. Zhang, P. Zhu and T. Yao, Chem.–Asian J., 2020, 15, 1175–1179 CrossRef CAS.
- Y. Liu, B. Hu, S. Wu, M. Wang, Z. Zhang, B. Cui, L. He and M. Du, Appl. Catal., B, 2019, 258, 117970 CrossRef CAS.
- M. Wang, M. Hu, B. Hu, C. Guo, Y. Song, Q. Jia, L. He, Z. Zhang and S. Fang, Biosens. Bioelectron., 2019, 135, 22–29 CrossRef CAS.
- M. Wang, L. Yang, B. Hu, J. Liu, L. He, Q. Jia, Y. Song and Z. Zhang, Biosens. Bioelectron., 2018, 113, 16–24 CrossRef CAS.
- Y. Wang, M. Yao, R. Ma, Q. Yuan, D. Yang, B. Cui, C. Ma, M. Liu and D. Hu, J. Mater. Chem. A, 2020, 8, 884–917 RSC.
- Q. Zhou, S. Wei and W. Han, J. Org. Chem., 2014, 79, 1454–1460 CrossRef CAS.
- P. Wójcik, M. Mart, S. Ulukanli and A. Trzeciak, RSC Adv., 2016, 6, 36491–36499 RSC.
-
(a) S. Shylesh, V. Schuenemann and W. R. Thiel, Angew. Chem., Int. Ed., 2010, 49, 3428–3459 CrossRef CAS;
(b) R. N. Baig, M. N. Nadagouda and R. S. Varma, Coord. Chem. Rev., 2015, 287, 137–156 CrossRef;
(c) A. M. Abu-Dief and S. M. Abdel-Fatah, Beni Suef University Journal of Basic and Applied Sciences, 2018, 7, 55–67 CrossRef;
(d) B. I. Kharisov, H. R. Dias and O. V. Kharissova, Arabian J. Chem., 2019, 12, 1234–1246 CrossRef CAS.
- M. Nasrollahzadeh, Molecules, 2018, 23, 2532 CrossRef.
- J. Niu, M. Liu, P. Wang, Y. Long, M. Xie, R. Li and J. Ma, New J. Chem., 2014, 38, 1471–1476 RSC.
- J. Niu, M. Xie, X. Zhu, Y. Long, P. Wang, R. Li and J. Ma, J. Mol. Catal. A: Chem., 2014, 392, 247–252 CrossRef CAS.
- X. Zhu, J. Niu, F. Zhang, J. Zhou, X. Li and J. Ma, New J. Chem., 2014, 38, 4622–4627 RSC.
- Y. Long, K. Liang, J. Niu, X. Tong, B. Yuan and J. Ma, New J. Chem., 2015, 39, 2988–2996 RSC.
- X. Sun, Y. Zheng, L. Sun, Q. Lin, H. Su and C. Qi, Nano-Struct. Nano-Objects, 2016, 5, 7–14 CrossRef CAS.
- S. You, C. Yan, R. Zhang and M. Cai, Appl. Organomet. Chem., 2019, 33, e4650 Search PubMed.
- C. Rösler and R. A. Fischer, CrystEngComm, 2015, 17, 199–217 RSC.
- W. Xiang, Y. Zhang, H. Lin and C.-j. Liu, Molecules, 2017, 22, 2103 CrossRef.
- Q. Yang, F. Yao, Y. Zhong, F. Chen, X. Shu, J. Sun, L. He, B. Wu, K. Hou and D. Wang, Part. Part. Syst. Charact., 2019, 36, 1800557 CrossRef.
- T. T. Dang, A. Chen and A. M. Seayad, RSC Adv., 2014, 4, 30019–30027 RSC.
- A. Augustyniak, W. Zawartka, J. Navarro and A. Trzeciak, Dalton Trans., 2016, 45, 13525–13531 RSC.
- A. O. Biying, V. R. Vangala, C. S. Chen, L. P. Stubs, N. S. Hosmane and Z. Yinghuai, Dalton Trans., 2014, 43, 5014–5020 RSC.
- V. B. Saptal, M. V. Saptal, R. S. Mane, T. Sasaki and B. M. Bhanage, ACS Omega, 2019, 4, 643–649 CrossRef CAS.
- A. M. Trzeciak, P. Wojcik, R. Lisiecki, Y. Gerasymchuk, W. Strek and J. Legendziewicz, Catalysts, 2019, 9, 319 CrossRef.
- N. Jiao, Z. Li, Y. Wang, J. Liu and C. Xia, RSC Adv., 2015, 5, 26913–26922 RSC.
- Y. Cui, X. Guo, Y. Wang and X. Guo, Chin. J. Catal., 2015, 36, 322–327 CrossRef CAS.
- T. Ketike, V. R. K. Velpula, V. R. Madduluri, S. R. R. Kamaraju and D. R. Burri, ChemistrySelect, 2018, 3, 7164–7169 CrossRef CAS.
- P. Wójcik, L. Sygellou, A. Gniewek, A. Skarżyńska and A. Trzeciak, ChemCatChem, 2017, 9, 4397–4409 CrossRef.
- P. Gautam, M. Dhiman, V. Polshettiwar and B. M. Bhanage, Green Chem., 2016, 18, 5890–5899 RSC.
- Y. Wan, F. Song, T. Ye, G. Li, D. Liu and Y. Lei, Appl. Organomet. Chem., 2019, 33, e4714 CrossRef.
- Y. Zhong, X. Gong, X. Zhu, Z. Ni, H. Wang, J. Fu and W. Han, RSC Adv., 2014, 4, 63216–63220 RSC.
- L. Cheng, Y. Zhong, Z. Ni, H. Du, F. Jin, Q. Rong and W. Han, RSC Adv., 2014, 4, 44312–44316 RSC.
-
(a) F. F. Tao, Chem. Soc. Rev., 2012, 41, 7977–7979 RSC;
(b) F. F. Tao, S. Zhang, L. Nguyen and X. Zhang, Chem. Soc. Rev., 2012, 41, 7980–7993 RSC.
-
(a) G. Sharma, A. Kumar, S. Sharma, M. Naushad, R. P. Dwivedi, Z. A. ALOthman and G. T. Mola, J. King Saud Univ., Sci., 2019, 31, 257–269 CrossRef;
(b) D. R. Pye and N. P. Mankad, Chem. Sci., 2017, 8, 1705–1718 RSC.
- Q. Hu, X. Liu, L. Tang, D. Min, T. Shi and W. Zhang, RSC Adv., 2017, 7, 7964–7972 RSC.
- Z.-J. Wang, X.-Y. Wang, X. Wang, Z.-W. Liang and X. Xu, Catal. Commun., 2017, 101, 10–14 CrossRef CAS.
|
This journal is © The Royal Society of Chemistry 2021 |
Click here to see how this site uses Cookies. View our privacy policy here.