DOI:
10.1039/D0RA09924D
(Paper)
RSC Adv., 2021,
11, 3346-3353
Synthesis of green and pure copper oxide nanoparticles using two plant resources via solid-state route and their phytotoxicity assessment
Received
23rd November 2020
, Accepted 21st December 2020
First published on 15th January 2021
Abstract
Among the conventional methods in synthesizing nanoparticles, the methods that use biological resources, as reducing and stabilizing agents, can be considered eco-friendly methods. In this study, the leaf tissue of green tea (Camellia sinensis L.) and lavender (Lavandula anguistifolia) were utilized by the solid-state method as a one-step and low-cost method for the biosynthesis of copper oxide nanoparticles (CuO NPs). The results of the X-ray Diffraction (XRD), field emission scanning electron spectroscopy (FESEM) and transmission electron microscopy (TEM) showed that lavender is more productive in the synthesis of pure and uniform CuO NPs (50 nm). Comparing biogenic synthesized CuO NPs with chemically synthesized CuO NPs in terms of induction of phytotoxicity, exposed in treatments with concentrations of 40, 400 and 4000 μg ml−1, green CuO NPs had less inhibitory effects on the seed germination factors (i.e., germination percentage, germination rate, shoot and root length, etc.) of lettuce (Lactuca sativa L.), and tomato (Solanum lycopersicum L.) seeds. However, both green/chemically synthesized CuO NPs at their lowest concentrations (4 μg ml−1), had an effective role in root and shoot expansion of lettuce and tomato seedlings.
Introduction
Nanotechnology and application of nanoscale materials play an essential role in serving humans.1 The shape and size of nanoparticles as the two main factors determine the unique magnetic, electronic, optical and catalytic properties of nanoparticles.2 Copper oxide nanoparticles (CuO NPs) due to their unique electric, thermal, mechanical, catalytic and magnetic properties, are used in various fields such as agricultural, environmental, industrial and medical.3,4 In general, all nanoparticles synthesis protocols are sorted into three main categories; physical, chemical and biological; and the naming of each of these methods is based on the reducing agent or electron source involved in the nanoparticle synthesis process.5 So that, in the physical method, the electron source is a physical source such as the electric current; in the chemical method, it is a chemical source (e.g., sodium borohydride and hydrazine); and in the biological method it is an organism or a biomolecule.6–9 The noteworthy, both of the physical and chemical methods are potentially hazardous for the environment and living organisms due to the usage of toxic reducing and stabilizing agents in the synthesis process. Additionally, these methods are time-consuming, costly and consume high amounts of energy.10 Therefore, the usage of biological methods to reduce environmental hazards, costs and energy consumption can be an appropriate alternative to the above-mentioned methods.11 Recently, green synthesis of nanoparticles, as a one-step and eco-friendly method has attracted the attention of researchers and industries. The basis of this low-cost method is the use of plant extracts and organic compounds in the extracts that play the major role as reducing agents.12,13
Various methods were employed in the past to synthesize different copper-based nanoparticles, such as chemical synthesis, electrochemical synthesis, solvothermal route and phytosynthesis.14–17 In this regard, several studies have reported the usage of herbal extracts in the green synthesis of Cu NPs such as Garcinia mangostana leaf extract, Rubus glaucus Benth, fruit and leaf extract, extract of seedless dates, leaves extract of Ocimum sanctum L., Punica granatum peel extract, root and leaf extract of Asparagus adscendens Roxb., fruit extract of Ziziphus spina-christi L. and leaf aqueous extract of Nerium oleander.2,13,18–23 In particular, the green synthesis of CuO NPs and their various applications in the biomedical, agricultural and environmental fields have been recently reviewed.24,25
Considering this background into account, in this work, we investigate the formation of CuO NPs using Lavandula anguistifolia and Camellia sinensis L. under solid-phase procedures as a very facile, inexpensive and eco-friendly method. Although no report has been published on the synthesis of CuO NPs in the solid-phase procedures, successful reports of the use of this method in the synthesis of Ag NPs have persuaded us to use this method in the synthesis of CuO NPs.26,27
Lavender (Lavandula anguistifolia), also known as medicinal lavender, is a shrub of the family Lamiaceae, native to the Mediterranean region, but is grown in many other climates of the world.28 This plant is a natural source of organic compounds such as coumarin, coumaric acid, ursolic acid, valeric acid, anthocyanins, essential oils, tannins, phytosterols, herniarin and glycolic acid.29 Tea (Camellia sinensis L.), belongs to the family Theacea, is the oldest caffeine-containing non-alcoholic beverage in the world.30 The biochemical compositions of tea leaves, such as alkaloids (theobromine, caffeine, etc.) and polyphenols (catechins and flavonoids), polysaccharides, volatile oils, vitamins and inorganic elements, have antimicrobial, antioxidant, anti-inflammatory and antiallergic activity.31 According to previous reports, the use of lavender in the biosynthesis of CuO NPs in this study is innovative and the only use of the tea plant in the biosynthesis of copper nanoparticles has been just in the aqueous phase, not in the solid phase.4,32–34 In the following, through phytotoxicity assessment, green synthesized CuO NPs were evaluated in comparison with the chemically synthesized CuO NPs. Hence, evaluating the phytotoxicity effectes of both green and chemically synthesized nanoparticles was performed using both seed germination and seedling growth assay of two plant species lettuce (Lactuca sativa L.), and tomato (Solanum lycopersicum L.).
Materials and methods
Chemicals
For the synthesis of CuO NPs, copper sulfate pentahydrate (CuSO4·5H2O) was purchased from Sigma-Aldrich and the absolute ethanol was commercially obtained from Merck (Germany).
Plant materials
The dry specimens of lavender (L. anguistifolia) and green tea (C. sinensis) were purchased from local market of Karaj, Iran. Then, unwanted impurities were removed from the samples and the leaves of both plants were separated from the prepared heterogeneous samples. Finally, in order to obtain the desired powder sample, the leaf samples were crushed via a mortar and pestle.
Synthesis of CuO NPs
For the synthesis of green CuO NPs using the solid-state route, the dry specimens of lavender and green tea leaves were thoroughly powdered usingamortar and pestle. In the following, different amounts of CuSO4·5H2O were added to the certain mass of lavender powder and different samples with different ratios (mCuSO4·5H2O
:
mlavender powder = 1
:
1, 1
:
2.5 and 1
:
5) were prepared, which were coded as Cu–L1, Cu–L2.5, and Cu–L5. Then the resulting mixtures were well-grounded again, attaining homogenized powder samples. Similarly, three additional samples were prepared by green tea leave powder so that the copper sulfate was mixed with green tea in different weight ratios (mCuSO4.5H2O
:
mgreen tea powder = 1
:
1, 1
:
2.5 and 1
:
5) and named Cu–GT1, Cu–GT2.5 and Cu–GT5. Next, all the as-prepared samples were placed in a furnace and heated up to 600 °C, and after 4 h, they were allowed to cool down to ambient temperature. The precipitates were collected and suspended in absolute ethanol using vortex for washing. Then, synthesized CuO NPs were separated using a centrifuge at 5000 rpm for 4 min, the supernatant was discarded and the pellet was re-suspended in deionized water. The washing process with water was repeated three times and the black CuO NPs precipitates were dried at 80 °C and stored for further utilization.
Characterization of CuO NPs
To monitor particle size and chemical composition of the current green-synthesized CuO NPs, X-ray diffraction (XRD, Philips model: PW1730, λ = 1.54056 Å (Cu Kα irradiation), 2θ = 20–70°) was employed. For calculation of the crystallite sizes (D) of the samples Scherrer's equation were employed:3
D = Kλ/(β × cos θ) |
where, K is the shape factor constant (0.9), λ is the wavelength of the X-ray (0.1546 nm), β is the broadening of the diffraction line at half-maximum in radians, and θ is the Bragg's diffraction angle. Moreover, field emission scanning electron microscopy (FESEM, a TESCAN MIRA II, with the 20 kV voltage) and transmission electron microscopy (TEM, EM208S Philips microscope with accelerating voltage of 100 kv) were utilized for evaluating the surface topology and morphology of the green-synthesized CuO NPs. For depicting the particle size histogram, the size of more than 100 particles in the TEM image was estimated.
Phytotoxicity study of CuO NPs
Tests were conducted using two plant species seeds, lettuce (Lactuca sativa L.) and tomato (Solanum lycopersicum L.), purchased from a local nursery. Test seeds were screened to remove broken, small and immature seeds. In the following, to remove the biological contamination, the seeds were sterilized using 5% sodium hypochlorite solution and rinsed thrice using distilled water. Nanoparticles were suspended directly in distilled water and discrete by ultra-sonic vibration for 30 min. Concentrations of 0, 4, 40, 400, 4000 μg ml−1 were prepared from green and chemically synthesized nanoparticles and distributed in the plates containing 25 seeds and the plates were placed in a germinator at 22 °C. Treatments were arranged in a complementary randomized design (CRD) with three replications. In order to evaluate the potential of nanoparticles toxicity on two studied plant species, six important attributes including germination percentage, germination rate, abnormal seedling, stem length (mm) and root length (mm) were recorded.
Statistical analysis
The experiments were conducted based on a completely randomized design (CRD) with three replications and the data were statistically analyzed using ANOVA to determine significant differences (defined as p ≤ 0.05) by using SAS software package. Fitting standard errors of the means (±SEM) were calculated for presentation of graphs. Fisher's least significant difference (LSD) was applied to compare the potential significant differences between treatments.
Results and discussion
Characterization of green as-synthesized CuO NPs
The microstructure and purity of the nanoparticles, the samples were characterized by XRD analysis (Fig. 1). XRD patterns of the samples produced using both plants in the mCuSO3·5H2O
:
mplant ratio of (1
:
5) (i.e., Cu–L5, Cu–GT5) showed diffraction peaks around 2θ = 32.7°, 35.8°, 39.1°, 49.2°, 53.9°, 58.2°, 61.7°, 66.8° and 68.7° corresponding to the planes (110), (111), (200), (202), (020), (202), (113), and (022), respectively, and all revealed clearly the synthesis of the pure crystalline particles of monoclinic CuO NPs.3,4 While decreasing the weight ratio of copper salt to plant powder up to 2.5 resulted in no significant changes in the XRD pattern of Cu–L2.5 sample, but it caused presence of some additional peaks in 2θ range between 20 to 30° in the case of Cu–GT2.5 sample, which were attributed to Cu2O impurity.35,36 When the ratio of mCuSO4·5H2O
:
mplant was fixed at (1
:
1), XRD pattern of Cu–GT1 displayed diffraction peaks at 2θ = 26.8 and 29.7 corresponding to Cu2O, and 2θ = 31.2 attributing to Cu(OH)2, along with CuO NPs signals. The XRD pattern of Cu–L1 sample similarly showed the impurity peaks, but the peak's intensities of the impurities were lower than Cu–GT1 sample. Moreover, according to the Scherrer's equation, the crystalline sizes were found to be 94.94, 124.34, 152.01, 52.44, 25.56, and 104.91 nm for Cu–GT5, Cu–GT2.5, Cu–GT1, Cu–L5, Cu–L2.5, and Cu–L1, respectively. Altogether, XRD results confirmed that with increasing the amount of plant powder, more pure CuO NPs could be produced, and besides lavender powder could led to the more pure CuO NPs compared to the green tea plant.
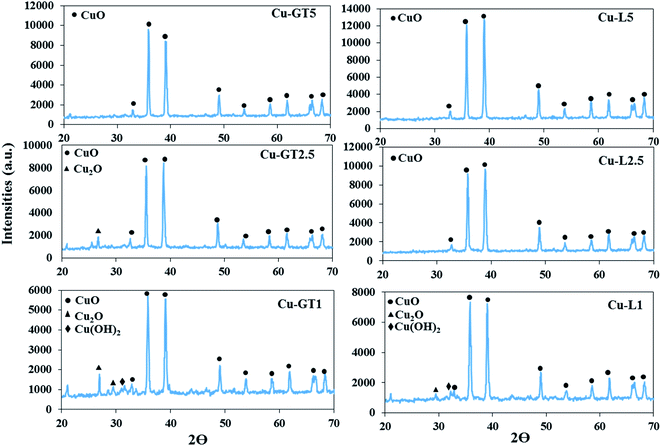 |
| Fig. 1 XRD patterns of CuO NPs synthesized using different amounts of lavender and green tea leaf powders via solid-state method. | |
In the next step, SEM analyses were employed to compare and contrast the dispersity and morphology of the as-synthesized CuO NPs (Fig. 2). SEM images showed that both plant resource type and the ratio of mCuSO4·5H2O
:
mplant played crucial roles in the morphology of the samples. For example, Cu–GT1 sample mostly consisted of large, rod shape stacked particles, but in Cu–GT2.5 sample, aggregated particles with polygonal shapes could be seen with the average particle size of 100 to 300 nm. In contrast, spherical CuO NPs (50 to 100 nm) were observed in the SEM image of the Cu–GT2.5 sample, which were to some extent agglomerated. SEM images of the samples produced using lavender plant (Cu–L2.5 and Cu–L5) showed tiny nanoparticles tightly stuck together. Interestingly, Cu–L1 sample displayed flower-like CuO NPs in its SEM image, in which growing rod shape particles probably formed a designed structure like flowers.
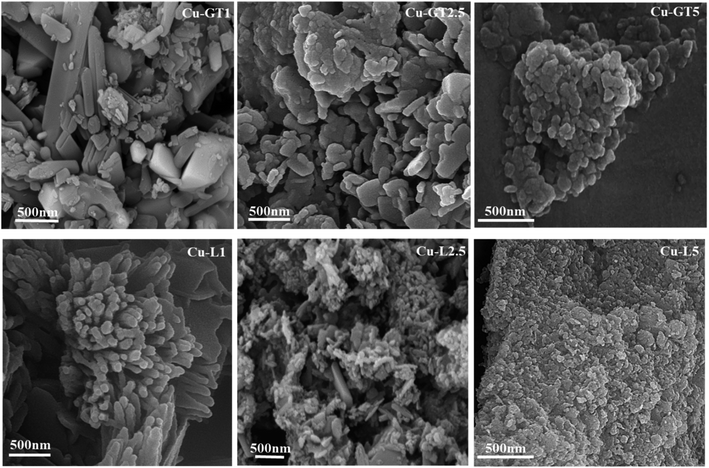 |
| Fig. 2 FESEM images of CuO NPs synthesized using different amounts of lavender and green tea leaf powders via solid-state method. | |
Moreover, for better clarification of the morphology of CuO NPs, TEM analysis was utilized. Based on the XRD and SEM results, Cu–L2.5 sample was selected for TEM analysis. The TEM images of this sample confirmed that CuO NPs were well-formed using lavender leaf powder through solid-state route (Fig. 3). It could be evidently seen uniform and spherical CuO NPs which were aggregated to some extent.
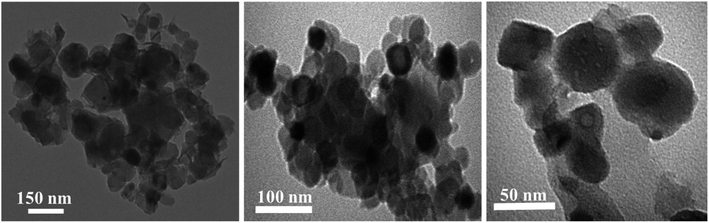 |
| Fig. 3 TEM images of Cu–L2.5 sample in different magnifications. | |
Phytotoxicity of green and chemically synthesized CuO NPs
The phytotoxicity assessment results showed that both green and chemically synthesized CuO NPs at the lowest concentration were effective on root and shoot development of seedlings of both plants specious but at higher concentrations had a limiting effect on all investigated germination factors. The results of the phytotoxicity assessment are presented in the form of pictures and diagrams (Fig. 4 and 5).
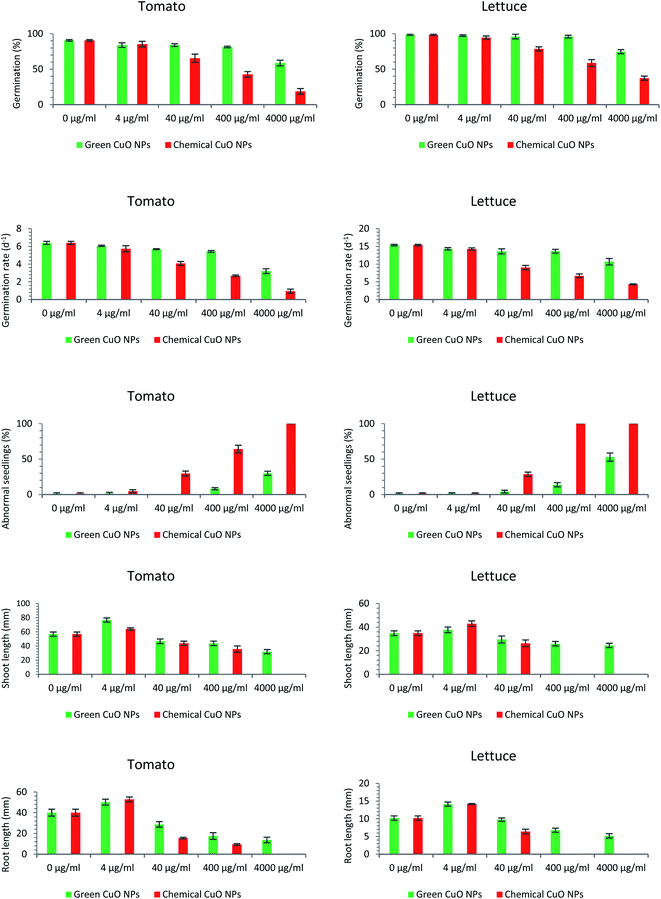 |
| Fig. 4 Effect of green and chemically synthesized copper oxide nanoparticles on five factors of seed germination of L. sativa and S. lycopersicum seeds (from top to bottom, germination percentage, germination rate, seedling length, root length and abnormal seedling percentage). | |
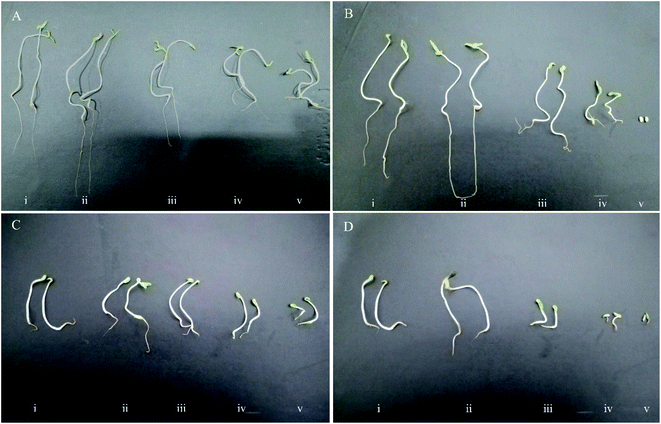 |
| Fig. 5 Effect of green CuO NPs (left) and chemically synthesized CuO NPs (right) on seed germination of S. lycopersicum (A and B) and L. sativa (C and D) under different concentrations of 0 (i), 4 (ii), 40 (iii), 400 (iv) and 4000 (v) μg ml−1. | |
Germination percentage (GP)
Following the evaluation of the phytotoxicity criteria of the green and chemically synthesized CuO NPs in this study, it was observed that germination percentage, as the first inspected factor, was affected by the toxicity of the nanoparticles. Taking tomato into account, the negative effect of green CuO NPs on the metric quantity was only visible at the highest concentration (4000 μg ml−1). However, the lower concentrations (4, 40 & 400 μg ml−1) did not have a significant negative effect on the germination percentage. With respect to chemically synthesized nanoparticles, decreasing oscillations began at a lower concentration (40 μg ml−1) and at concentrations of 40, 400 & 4000 μg ml−1 GP values of 65.33, 42.66 and 18.67%, were acquired, respectively (Fig. 4). The effect of nanoparticles on the pattern of germination of the lettuce seeds was similar to that of previous plant. The only effective concentration of green CuO NPs on germination was observed at concentrations of 4000 μg ml−1, while in relation to chemically synthesized CuO NPs concentrations of 40, 400 & 4000 μg ml−1, the germination percentage was reduced to 78.67, 58.67 and 37.33%, respectively (Fig. 4). When comparing the germination of seeds of two plants, it was noted that the tomato seeds showed more sensitivity to nanoparticle toxicity. Comparing nanoparticles in terms of their effects on seed germination, observations disclosed that the chemically synthesized CuO NPs have a greater inhibitory effect than green CuO NPs.
Germination rate
Observations showed that the germination rate of tomato and lettuce seeds was also considerably affected by both kinds of the CuO NPs. Regarding the first plant species, tomato, increasing the concentration of the chemically synthesized CuO NPs caused a downward trend in the germination rate. So that, the germination rate decreased from 6.4 (in 0 μg ml−1 CuO NPs) to 0.95 (in 4000 μg ml−1 CuO NPs). Green CuO NPs except at 4000 μg ml−1 concentration had no striking effect on germination rate of tomato seeds. In fact, chemically and green synthesized CuO NPs had a similar effect on the germination rate of both plant species. In lettuce, the calculated value of 15.39 in control treatment was abridged to 14.27, 9.04, 6.71 & 4.32 under the influence of increasing the concentration of chemically synthesized CuO NPs to 4, 40, 400 & 4000 μg ml−1, respectively (Fig. 4). However, the only substantial inhibitory effect of green CuO NPs was recorded at the concentration of 4000 μg ml−1, which reduced the index value to 10.68.
Abnormal seedling
Similar to the germination percentage and the germination rate criteria, it was found that a large proportion of the tomato and lettuce seedlings were more negatively influenced by the high concentrations of chemically synthesized CuO NPs more than green CuO NPs. The abnormal seedlings mainly showed the symptoms of short and necrotic roots as well as short and springy shoots (Fig. 5). The green CuO NPs suspensions at concentrations of 400 & 4000 μg ml−1 induced abnormal seedlings with abundance 8.25% and 30% in tomato seedlings, whereas these quantities in lettuce seedlings were 13.84 and 52.93%, respectively. The observations suggested that chemically synthesized CuO NPs encompass more side effects on the appearance of abnormal seedlings. So that, the increment abnormal symptoms began under the influence of chemically synthesized CuO NPs at a concentration of 40 μg ml−1 in both of the plant species seedlings. Interestingly, no normal tomato seedlings were observed in the last treatment (i.e., 4000 μg ml−1) and actually, the extremely toxic treatments for lettuce seedlings were 400 and 4000 μg ml−1 (Fig. 4).
Shoot and root length
Remarkably, it was inferred that both green and chemically synthesized CuO NPs at their lowest concentration (4 μg ml−1), stimulated the shoot and root elongation in the seedlings of both plant species (Fig. 5). Contrary to this positive effect, the results indicated that higher concentrations of both green and chemically synthesized CuO NPs were toxic for seedling destruction. As the concentration of both nanoparticles increased, the recorded lengths of root and shoot for both plants declined dramatically. Finally, the higher toxicity of the chemically synthesized CuO NPs compared to the green form as well as the higher vulnerability of the root compared to the shoot against the inhibitory effect of nanoparticles on elongation were clearly visible (Fig. 4).
The effect of nanoparticles on plants can be very diverse based on NPs characteristics such as type, size, concentration, chemical and physical properties as well as plant-specific features.37 Some reports indicated an influential role of metallic nanoparticles in damaging living organism's DNA, but with unknown molecular mechanisms.38 The most prominent observations in this study were the lower phytotoxic effects of green synthesized CuO NPs compared to the chemically synthesized CuO NPs, on the germination indices. In the last two decades, a number of reports have also presented the inhibitory effects of copper nanoparticles on plant growth factors; For example, the results of a study revealed the growth of Triticum aestivum and Phaseolus radiatus seedlings was limited under treatments of 200–1000 mg l−1 of Cu NPs.39 The 15 nm sized Cu NPs synthesized by polyols process method reduced stem and root elongation in Eruca sativa plant under the treatment of Murashige and Skoog (MS) medium containing 30 μg ml−1 Cu NPs.40 In another study, the root and shoot elongation of two important plant species, Glycine max and Cicer arietinum, significantly decreased in concentration of >100 ppm of copper oxide nanoparticles.41 According to the copper oxide NPs phytotoxicity results, Glycine max seedlings were negatively affected by concentrations more than 50 mg l−1. However, the roots showed superior sensitivity to copper oxide NPs toxicity compared with the shoots.42
Despite the reports of the mentioned researches, comparing the toxic effect of green and chemically synthesized CuO NPs on plant growth characteristics has not been reported so far.
Similar to the results of this study, there are reports of the positive effect of nanoparticles on the growth attributes of some plant species. In a recent study, 25 and 50 mg l−1 copper nanoparticles treatments significantly stimulated the soybean primary roots elongation.43 Furthermore, it has been reported that concentrations of less than 1 ppm (0.2–1.0 ppm) of copper nanoparticles in wheat seedling medium led to a significant effect on increasing root dry weight and leaf area.44 However, the massive accumulation of nanoparticles in shoots and roots could lead to phytotoxicity, the lower concentration of nanoparticles induced activity of antioxidant enzyme system, rubisco and chloroplast; therefore, the activity of these biosynthetic systems under low concentrations of nanoparticles may be effective in stimulating plant growth.44–47
In general, the toxicity of a nanoparticle can be attributed to the properties of the nanoparticle (size, surface area and intrinsic catalytic activity) and the reducing and stabilizing agents used in its synthesis and since the green nanoparticles at least lacks potential toxic reducing and stabilizing agents, these behave more gently in inducing phytotoxicity. Comparing green CuO NPs to chemically synthesized CuO NPs, some research reported that the green NPs induced less phytotoxicity whereas the chemically synthesized NPs showed more severe inhibitory effects. The results of trial using green and chemically synthesized Ag NPs with concentrations of 0, 100, 200, 400 and 600 ppm on the seed germination percentage and seedling length of Matricaria chamomilla and Ocimum basilicum revealed that, the green Ag NPs was significantly less toxic than chemically synthesized Ag NPs.26 According to another report, the green Ag NPs nanoparticles synthesized by Laminaria japonica algal extract had no significant effect on the germination of Triticum aestivum and Phaseolus mungo seeds at the concentration of 0–80 ppm, whereas the non-toxic effect of nanoparticles on shoot and root elongation was factual at concentrations of below 30 ppm.48
Generally, CuO NPs have potentially inhibitory effects on germination features (e.g., germination percentage, shoot and root elongation, etc.). Nevertheless, depending on their synthesis process whether green or chemically synthesized, different intensities of induced toxicity can be observed. Thus, green CuO NPs with the effects of toxicity much less than the chemically synthesized CuO NPs could be recognized as more eco-friendly NPs. Interestingly, CuO NPs can have a dual performance depending on their concentration, a nanotoxicant at high concentrations or a nanonutrient at low concentrations. At a constant concentration, each of the germination indices compared to the other exhibits various responses from inattention to sensitivity or stimulation. Different plant species could show variability in response patterns in the same CuO NPs treatments.
Conclusions
From the outcome of our investigation, it is possible to conclude that, lavender as an organic and green source has been fruitful in synthesis of CuO NPs. Comparison of biosynthesized CuO NP with chemically synthesized CuO in relation to phytotoxicity through seed germination test disclosed the biosynthesized CuO NP has little inhibitory effects on germination factors. However, in contrast, the adverse effects of chemically synthesized CuO NP on seed germination factors were more severe. Despite the phytotoxicity and limiting effects on seed germination and seedling growth due to the use of the CuO NPs, it was observed that both green and chemical CuO NPs at low concentrations (4 μg ml−1) effectively stimulated root and shoot elongation of lettuce and tomato seedlings. The use of lavender as a biogenic reducing and stabilizing agent, solid-state route as a low cost, safe and convenient method and the low toxicity effect of green CuO NPs made in this research can be considered as an eco-friendly research. The proposed method can be readily used in practice and can be successfully used for a number of fields related to nanotechnology.
Conflicts of interest
There are no conflicts to declare.
Acknowledgements
The authors would like to acknowledge the University of Tehran for the financial support of this work.
References
- E. K. Elumalai, T. N. Prasad, J. Hemachandran, S. V. Therasa, T. Thirumalai and E. David, J Pharm Sci Res, 2010, 2, 549–554 CAS
. - Y. T. Prabhu, K. V. Rao, V. S. Sai and T. Pavani, J. Saudi Chem. Soc., 2017, 21, 180–185 CrossRef CAS
. - S. Sukumar, A. Rudrasenan and D. Padmanabhan Nambiar, ACS Omega, 2020, 5, 1040–1051 CrossRef CAS
. - J. Sarkar, N. Chakraborty, A. Chatterjee, A. Bhattacharjee, D. Dasgupta and K. Acharya, Nanomaterials, 2020, 10, 312 CrossRef CAS
. - S. Shende, A. P. Ingle, A. Gade and M. Rai, World J. Microbiol. Biotechnol., 2015, 31, 865–873 CrossRef CAS
. - R. Zhou, X. Wu, X. Hao, F. Zhou, H. Li and W. Rao, Nucl. Instrum. Methods Phys. Res. Sect. B Beam Interact. Mater. Atoms, 2008, 266, 599–603 CrossRef CAS
. - Q. M. LIU, D. B. ZHOU, Y. Yamamoto, R. Ichino and M. Okido, Trans. Nonferrous Metals Soc. China, 2012, 22, 117–123 CrossRef CAS
. - X. Su, J. Zhao, H. Bala, Y. Zhu, Y. Gao, S. Ma and Z. Wang, J. Phys. Chem. C, 2007, 111, 14689–14693 CrossRef CAS
. - R. Cuevas, N. Durán, M. C. Diez, G. R. Tortella and O. Rubilar, J. Nanomater., 2015, 2015 Search PubMed
. - A. K. Mittal, Y. Chisti and U. C. Banerjee, Biotechnol. Adv., 2013, 31, 346–356 CrossRef CAS
. - K. Vithiya and S. Sen, Int. J. Pharm. Sci. Res., 2011, 2, 2781 CAS
. - P. Parikh, D. Zala and B. A. Makwana, Open Access Library, 2014, 1, 1–15 Search PubMed
. - M. Gopinath, R. Subbaiya, M. M. Selvam and D. Suresh, Int. J. Curr. Microbiol. Appl. Sci., 2014, 3, 814–818 Search PubMed
. - S. P. Mondal, K. Das, A. Dhar and S. K. Ray, Nanotechnology, 2007, 18, 095606 CrossRef
. - D. Mo, J. Liu, H. J. Yao, J. L. Duan, M. D. Hou, Y. M. Sun, Y. F. Chen, Z. H. Xue and L. Zhang, J. Cryst. Growth, 2008, 310, 612–616 CrossRef CAS
. - J. S. Jang, U. A. Joshi and J. S. Lee, J. Phys. Chem. C, 2007, 111, 13280–13287 CrossRef CAS
. - R. C. Kasana, N. R. Panwar, R. K. Kaul and P. Kumar, Environ. Chem. Lett., 2017, 15, 233–240 CrossRef CAS
. - B. Kumar, K. Smita, L. Cumbal, A. Debut and Y. Angulo, J. Saudi Chem. Soc., 2017, 21, S475–S480 CrossRef CAS
. - E. A. Mohamed, Heliyon, 2020, 6, e03123 CrossRef
. - S. Usha, K. T. Ramappa, S. Hiregoudar, G. D. Vasanthkumar and D. S. Aswathanarayana, Int. J. Curr. Microbiol. Appl. Sci., 2017, 6, 2219–2228 CrossRef
. - P. Kaur, R. Thakur and A. Chaudhury, Green Chem. Lett. Rev., 2016, 9, 33–38 CrossRef CAS
. - S. Thakur, S. Sharma, S. Thakur and R. Rai, Int. J. Curr. Microbiol. Appl. Sci., 2018, 7, 683–694 CrossRef
. - R. Khani, B. Roostaei, G. Bagherzade and M. Moudi, J. Mol. Liq., 2018, 255, 541–549 CrossRef CAS
. - S. A. Akintelu, A. S. Folorunso, F. A. Folorunso and A. K. Oyebamiji, Heliyon, 2020, 6, e04508 CrossRef
. - K. S. Siddiqi and A. Husen, Biomater. Res., 2020, 24, 1–15 CrossRef
. - J. Nasiri, M. Rahimi, Z. Hamezadeh, E. Motamedi and M. R. Naghavi, J. Cleaner Prod., 2018, 192, 514–530 CrossRef CAS
. - M. R. Moghadas, E. Motamedi, J. Nasiri, M. R. Naghavi and M. Sabokdast, Heliyon, 2020, 6, e04730 CrossRef
. - R. Prusinowska and K. B. Śmigielski, Herba Pol., 2014, 60, 56–66 Search PubMed
. - K. B. Śmigielski, R. Prusinowska, K. Krosowiak and M. Sikora, J. Essent. Oil Res., 2013, 25, 291–299 CrossRef
. - Y. S. Lin, Y. J. Tsai, J. S. Tsay and J. K. Lin, J. Agric. Food Chem., 2003, 51, 1864–1873 CrossRef CAS
. - A. B. Sharangi, Food Res. Int., 2009, 42, 529–535 CrossRef CAS
. - M. I. Din, F. Arshad, Z. Hussain and M. Mukhtar, Nanoscale Res. Lett., 2017, 12, 638 CrossRef
. - P. Sutradhar, M. Saha and D. Maiti, J. Nanostruct. Chem., 2014, 4, 86 CrossRef
. - A. H. Keihan, H. Veisi and H. Veasi, Appl. Organomet. Chem., 2017, 31, e3642 CrossRef
. - R. Borah, E. Saikia, S. J. Bora and B. Chetia, RSC Adv., 2016, 6, 100443–100447 RSC
. - F. Nishino, M. Jeem, L. Zhang, K. Okamoto, S. Okabe and S. Watanabe, Sci. Rep., 2017, 7, 1–11 CrossRef CAS
. - T. A. Shalaby, Y. Bayoumi, N. Abdalla, H. Taha, T. Alshaal, S. Shehata, M. Amer, É. Domokos-Szabolcsy and H. El-Ramady, Nanoscience in Food and Agriculture, 2016, 1, 283–312 CrossRef
. - D. H. Atha, H. Wang, E. J. Petersen, D. Cleveland, R. D. Holbrook, P. Jaruga, M. Dizdaroglu, B. Xing and B. C. Nelson, Environ. Sci. Technol., 2012, 46, 1819–1827 CrossRef CAS
. - W. M. Lee, Y. J. An, H. Yoon and H. S. Kweon, Environ. Toxicol. Chem., 2008, 27, 1915–1921 CrossRef CAS
. - M. Zaka, B. H. Abbasi, L. U. Rahman, A. Shah and M. Zia, IET Nanobiotechnol., 2016, 10, 134–140 CrossRef
. - T. Adhikari, S. Kundu, A. K. Biswas, J. C. Tarafdar and A. S. Rao, J. Agric. Sci. Technol. A, 2012, 2, 815 CAS
. - P. M. G. Nair and I. M. Chung, Biol. Trace Elem. Res., 2014, 162, 342–352 CrossRef CAS
. - T. H. Pham, C. M. Nguyen, L. Le Quynh, K. B. Ninh, V. M. Chau, H. C. Nguyen, Q. B. Ngo, T. H. Dao, T. V. Nguyen, T. H. Le Thi and M. L. Tran, Int. J. Agric. Biol., 2018, 20, 1562–1568 Search PubMed
. - A. Hafeez, A. Razzaq, T. Mahmood and H. M. Jhanzab, J Nanosci Adv Technol, 2015, 1, 6–11 Search PubMed
. - G. F. Nekrasova, O. S. Ushakova, A. E. Ermakov, M. A. Uimin and I. V. Byzov, Russ. J. Ecol., 2011, 42, 458 CrossRef CAS
. - F. Gao, F. Hong, C. Liu, L. Zheng, M. Su, X. Wu, F. Yang, C. Wu and P. Yang, Biol. Trace Elem. Res., 2006, 111, 239–253 CrossRef CAS
. - F. Hong, J. Zhou, C. Liu, F. Yang, C. Wu, L. Zheng and P. Yang, Biol. Trace Elem. Res., 2005, 105, 269–279 CrossRef CAS
. - D. Y. Kim, R. G. Saratale, S. Shinde, A. Syed, F. Ameen and G. Ghodake, J. Cleaner Prod., 2018, 172, 2910–2918 CrossRef CAS
.
|
This journal is © The Royal Society of Chemistry 2021 |
Click here to see how this site uses Cookies. View our privacy policy here.