DOI:
10.1039/D0RA10203B
(Paper)
RSC Adv., 2021,
11, 11266-11272
A luminescent Cd(II) coordination polymer as a multi-responsive fluorescent sensor for Zn2+, Fe3+ and Cr2O72− in water with fluorescence enhancement or quenching†
Received
15th December 2020
, Accepted 23rd February 2021
First published on 17th March 2021
Abstract
A luminescent Cd(II) coordination polymer, namely {[Cd(btic)(phen)]·0.5H2O}n (CP-1) (H2btic = 5-(2-benzothiazolyl)isophthalic acid, phen = 1,10-phenanthroline), was constructed through the mixed-ligand method under solvothermal conditions. CP-1 manifests a chain structure decorated with uncoordinated Lewis basic N and S donors. CP-1 exhibits high sensing towards Zn2+, Fe3+ and Cr2O72− ions with fluorescence enhancement or quenching. CP-1 exhibited a fluorescence enhancement for Zn2+ ions through weak binding to S and N atoms, and a fluorescence quenching for Fe3+ and Cr2O72− ions by an energy transfer process. The binding constants were calculated as 1.812 × 104 mol−1 for Zn2+, 4.959 × 104 mol−1 for Fe3+ and 1.793 × 104 mol−1 for Cr2O72−. This study shows CP-1 as a rare multi-responsive sensor material for the efficient detection of Zn2+, Fe3+ and Cr2O72− ions.
Introduction
Zn2+ and Fe3+ are essential metal ions, which are involved in numerous biological processes in human body.1–6 The abnormal levels of Zn2+ and Fe3+ can cause numerous adverse health effects. Hence, efficient methods for the detection of Zn2+ and Fe3+ are highly desirable. On the other hand, Cr2O72− is a type of environmentally non-biodegradable pollutant, which can cause accumulation in living organisms and lead to the visceral damage and water-borne diseases because of its potent mutagenesis and carcinogenesis.7–9 Therefore, methods for the efficient detection of Cr2O72− are urgent to be explored. Currently, several traditional methods have been developed for the determination of Zn2+, Fe3+ and Cr2O72−, such as atomic absorption spectrophotometry,10–12 electrochemical methods,13–15 and inductively coupled plasma mass spectrometry.16–18 However, these methods are usually expensive, time-consuming, or require complicated sample preparation processes. Therefore, simple and efficient detection methods for such analytes are urgently required.
Coordination polymers (CPs) are coordination compounds with infinite structures (1, 2 or 3 dimensions), which are constructed from metal ions and ligands via coordination bonds.19 CPs as a type of promising functional materials have received considerable attention because of their intriguing structural features as well as applications in luminescence sensing, magnetic property, gas storage, drug delivery, and so on.20–28 Particularly, CPs as fluorescent sensors have drew more concern owing to their superiority in long-term stability, efficiency, and operability. Simultaneously, much effort has been made to synthesize fluorescent CP materials for detecting different types of target analytes, such as metal ions,29,30 anions,31,32 and small molecules.33,34 However, many of these specific target analytes are detected in organic solvents, such as ethanol,35,36 DMF,37–39 CH3CN,40 DMA,41 which are not beneficial for the practical applications of fluorescent CPs. Therefore, detecting analytes in water is still an important challenge in biological and environmental sciences.
Organic ligands are crucial in the syntheses of fluorescent CPs. Aromatic or conjugated π moieties within organic ligands can endow the CPs with excellent optical properties, which can improve the efficient recognition for the target analytes.42–44 Moreover, the Lewis basic sites in fluorescent CPs can interact with certain metal cations, which can enhance the selective sensing capacity towards metal cations.45 Based on the above considerations, we adopted a multidentate ligand 5-(2-benzothiazolyl)isophthalic acid (H2btic) as a main ligand, which contains two aromatic rings. Such ligand was accompanied by an auxiliary ligand with excellent optical properties 1,10-phenanthroline (phen) to fabricate a luminescent CP, namely {[Cd(btic)(phen)]·0.5H2O}n (CP-1). CP-1 magnified a chain structure decorated with uncoordinated Lewis basic N and S donors. The luminescence sensing behavior of CP-1 was studied in water solution. The as-synthesized CP-1 showed a striking sensing capability towards Zn2+, Fe3+ and Cr2O72− ions with fluorescence enhancement or quenching. In addition, the mechanism of luminescence enhancement or quenching has also been discussed.
Experimental section
Materials and instruments
All commercially available chemicals were used as received. Elemental analyses were conducted using a Flash EA 1112 elemental analyzer. IR data were obtained using a BRUKER TENSOR 27 spectrophotometer. PXRD patterns were obtained on a Bruker AXS D8Advance. Thermogravimetric analyses were measured on a NetzschSTA449C thermal analyzer under air atmosphere at a ramp rate of 10 °C min−1. The luminescence properties were studied using a Hitachi F-7000 fluorescence spectrophotometer. XPS data were obtained on a Thermo ESCALAB 250 X-ray photoelectron spectrometer.
Synthesis of the ligand H2btic
Ligand H2btic was synthesized according to the reported procedure.46 The detailed synthetic method is described in the ESI (Section 1†).
Synthesis of {[Cd(btic)(phen)]·0.5H2O}n (CP-1)
A mixture of Cd(NO3)2·4H2O (0.1 mmol), H2btic (0.1 mmol), and phen (0.05 mmol) in 5 mL of the component solvent (DEF
:
H2O = 4
:
1) (DEF = N,N′-diethylformamide) was put into a glass vessel, which was heated at 80 °C until the colorless crystals appeared. Yield: 68% (based on Cd). Anal. calcd for C54H32N6O9S2Cd2 (%): C, 54.15; H, 2.69; N, 7.02; S, 5.35. Found: C, 53.68; H, 2.51; N, 7.10; S, 5.19. IR (cm−1, KBr): 3422(s), 3131(w), 3060(w), 2970(w), 2926(w), 1610(s), 1551(s), 1513(m), 1433(s), 1364(s), 1310(w), 1249(w), 1139(w), 1104(m), 1031(w), 934(w), 887(w), 777(s), 722(s), 686(w), 638(m).
Fluorescence sensing experiments
1 mg of CP-1 was placed separately into 2 mL aqueous solutions containing numerous analytes (10−3 M). After an ultrasonic treatment for 15 min, the photoluminescence responses were recorded with excitation at 278 nm. The fluorescence titrations were conducted through adding analytes into 2 mL suspension of CP-1.
X-ray crystallography
X-ray diffraction data were collected on a Bruker SMART APEX-II CCD diffractometer. The crystal structure was solved and refined using the SHELX-2014 software.47 Hydrogen atoms were added geometrically. Crystallographic parameters for CP-1 are shown in Table 1. Table 2 shows the main bond lengths and bond angles.
Table 1 Crystal data and structure refinement details for CP-1
R1 = ∑‖Fo| − |Fc‖/∑|Fo|. wR2 = [∑w(Fo2 − Fc2)2/∑w(Fo2)2]1/2. |
Compound |
1 |
Formula |
C54H32N6O9S2Cd2 |
fw |
1195.76 |
Crystal system |
Monoclinic |
Space group |
P2/c |
a/Å |
10.1136(10) |
b/Å |
9.7173(10) |
c/Å |
24.005(3) |
α/deg |
90 |
β/deg |
92.928(4) |
γ/deg |
90 |
V/Å3 |
2356.1(4) |
Z |
2 |
2θmax (deg) |
55.082 |
Dc/g cm−3 |
1.686 |
Reflns collected/unique |
48 969/5434 |
R(int) |
0.0699 |
Abs coeff/mm−1 |
1.059 |
F(000) |
1192 |
GOF |
1.054 |
R1 [I > 2σ(I)]a |
0.0415 |
wR2 (all data)b |
0.0791 |
Largest diff. Peak and hole |
0.64 and −0.70 e Å−3 |
Table 2 Selected bond lengths (Å) and bond angles (deg) for CP-1a
Symmetry transformations used to generate equivalent atoms in CP (1): #1 −1 + x, y, z; #2 1 − x, y, 3/2 − z. |
CP-1 |
|
|
|
Cd(1)–O(3)#1 |
2.236(2) |
Cd(1)–N(2) |
2.314(3) |
Cd(1)–O(1)#2 |
2.353(2) |
Cd(1)–N(1) |
2.371(3) |
Cd(1)–O(2)#2 |
2.402(3) |
Cd(1)–O(1) |
2.522(2) |
Cd(1)–O(4)#1 |
2.528(3) |
O(3)#1–Cd(1)–N(2) |
135.13(9) |
O(3)#1–Cd(1)–O(1)#2 |
101.27(10) |
N(2)–Cd(1)–O(1)#2 |
86.18(9) |
O(3)#1–Cd(1)–N(1) |
101.03(11) |
N(2)–Cd(1)–N(1) |
71.08(10) |
O(1)#2–Cd(1)–N(1) |
155.64(9) |
O(3)#1–Cd(1)–O(2) |
82.98(9) |
N(2)–Cd(1)–O(2) |
137.08(9) |
O(1)#2–Cd(1)–O(2) |
107.77(10) |
N(1)–Cd(1)–O(2) |
84.58(11) |
O(3)#1–Cd(1)–O(1) |
129.17(8) |
N(2)–Cd(1)–O(1) |
95.62(8) |
O(1)#2–Cd(1)–O(1) |
75.24(9) |
N(1)–Cd(1)–O(1) |
97.87(9) |
O(2)–Cd(1)–O(1) |
52.41(8) |
O(3)#1–Cd(1)–O(4)#1 |
54.00(8) |
N(2)–Cd(1)–O(4)#1 |
81.42(8) |
O(1)#2–Cd(1)–O(4)#1 |
95.36(9) |
N(1)–Cd(1)–O(4)#1 |
89.88(10) |
O(2)–Cd(1)–O(4)#1 |
134.66(8) |
O(1)–Cd(1)–O(4)#1 |
170.36(9) |
Results and discussion
Crystal structure of CP-1
CP-1 crystallizes in the monoclinic space group P2/c. As depicted in Fig. 1a, the asymmetric unit contained one Cd(II) ion, one btic2− and one phen, and half lattice H2O. Each Cd(II) ion adopted a pentagonal-bipyramidal environment finished by five O atoms of three btic2− and two N atoms of one phen. The Cd–O/N bond lengths varied from 2.236(2)–2.528(3) Å, and O/N–Cd–O/N bond angles were 52.41(8)–170.36(9)°, which coincided with those of the reported Cd(II) CPs.48–50 Different coordination modes were shown by the two carboxylate groups of btic2−, namely μ2-η1:η2 and μ1-η1:η1 modes. Then, Cd(II) ions were connected by carboxylate groups from btic2− ligands to produce a Cd(btic) chain (Fig. 1b). The Cd(btic) chain was made up of dinuclear units Cd2(COO)2. The phen ligands took bidentate chelating fashion coordinating to Cd(II) ions to satisfy the coordination demands of Cd(II) ions in the assembly process. As shown in Fig. 1c, these chains in an offset way were stacked into a 3D supramolecule via weak van der Waals interactions. Further, the π–π interactions in each chain between pyridine rings (N2–C16–C17–C18–C19–C20) of phen ligands stabilized the structure. In CP-1, the N and S donors in ligand btic2− were not involved in the coordination to Cd(II) ions. Therefore, the uncoordinated N and S could function as Lewis bases to recognize numerous analytes.
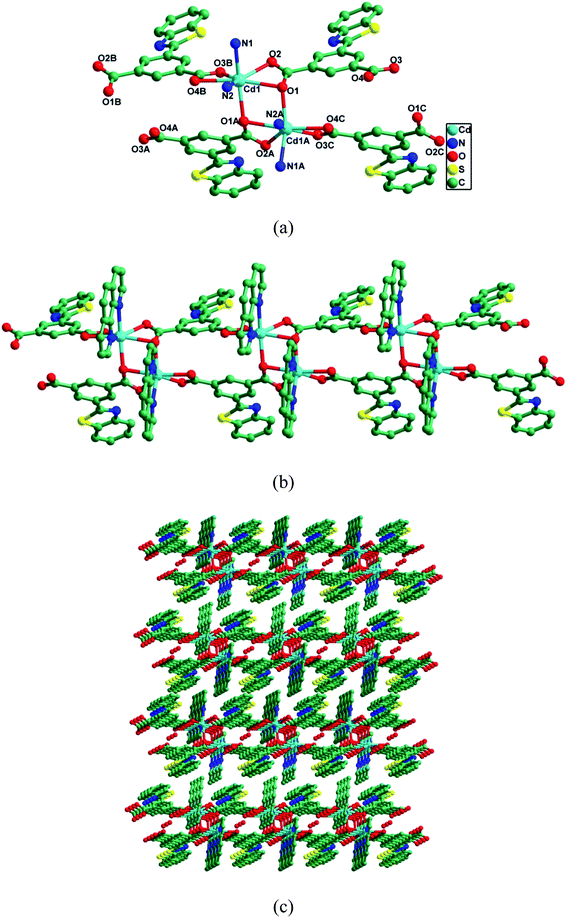 |
| Fig. 1 (a) Coordination environment around the Cd(II) center in CP-1. Hydrogen atoms and solvent molecules are omitted for clarity. Symmetry codes: A = 1 − x, y, 1.5 − z; B = −1 + x, y, z; C = 2 − x, y, 1.5 − z. (b) The chain structure of CP-1. (c) The 3D supramolecular structure of CP-1. | |
PXRD and thermogravimetric analysis
To check the phase purity of CP-1, its PXRD was performed (Fig. S1†). The peak positions of the as-synthesized sample were consistent with those of the simulated ones. The crystals of CP-1 were stable in air. Moreover, it did not dissolve in water or common organic solvents. To check the chemical stability of CP-1, each finely ground powder of CP-1 was immersed in methanol, ethanol, DMF, H2O and THF solvents for 24 h. Then, the PXRD of each sample was analyzed (Fig. S2†). The unchanged PXRD patterns revealed that the crystallinity of CP-1 retained after the solvent treatment, indicating the high chemical stability of CP-1. Thermogravimetric analysis was carried out to understand the thermal stability of CP-1 (Fig. S3†). The TGA curve of CP-1 exhibited an initial weight loss of 2.21% from 108 to 193 °C because of the release of H2O molecules (calcd: 1.51%). The further weight loss from 349–640 °C was ascribed to the disintegration of the structure, leaving CdO as the residue (found, 22.13%; calcd, 21.73%).
Photoluminescence properties
At ambient temperature, the solid-state luminescences of H2btic, phen, and CP-1 were measured (Fig. S4†). When excited at 278 nm, H2btic had an emission maximum at 433 nm as well as phen showed a main peak at 382 nm with two shoulder peaks at 365 nm and 403 nm. The excitation of CP-1 at 278 nm led to a dominant peak at 388 nm with two shoulder peaks at 375 nm and 409 nm, which probably originated from intraligand transitions because similar emission was observed for ligand phen. CP-1 showed a small redshift compared to that of phen, which was probably because of the coordination effect of ligands to Cd(II) ions. Further, a stronger emission band in CP-1 was probably assigned to the enhanced rigidity of the ligand that diminished a radiationless decay through the coordination to metal centers.51–53
Sensing of metal ions
The Lewis basic N and S active sites, good chemical stability and strong luminescence for CP-1 made it a potential candidate as a fluorescent sensor. Thus, we explored the application of CP-1 in detecting metal ions. 1 mg powder of CP-1 was placed separately in 2 mL 10−3 M Mx+ aqueous solutions (Mx+ = Na+, K+, Mg2+, Ca2+, Cr3+, Ag+, Zn2+, Hg2+, Pb2+, Ni2+, Co2+, Cd2+, Ba2+, Al3+, Cu2+, Mn2+, and Fe3+). Then, luminescence responses toward different metal ions were examined. As shown in Fig. 2, these metal ions exhibited different impacts on the fluorescence intensities of CP-1. Notably, Zn2+ ions enhanced the emission intensity by 2.17-fold, Fe3+ ions almost completely quenched the luminescence, while other metal ions had little or moderate quenching effects on the emission, indicating that CP-1 could act as a sensor material with a great response for Zn2+ and Fe3+ ions.
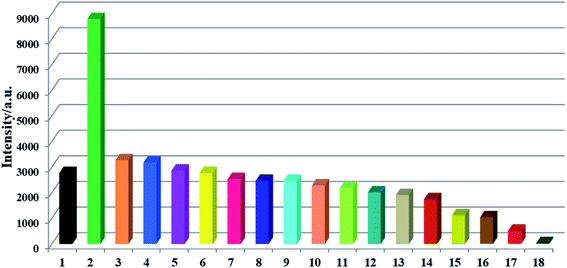 |
| Fig. 2 Luminescence intensity histograms of CP-1 (1 mg) dispersed in aqueous solutions of numerous metal ions (2 mL, 10−3 M) excited at 278 nm (1) blank, (2) Zn2+, (3) Ca2+, (4) Ba2+, (5) Mg2+, (6) K+, (7) Na+, (8) Cd2+, (9) Mn2+, (10) Al3+, (11) Ag+, (12) Cr3+, (13) Ni2+, (14) Co2+, (15) Pb2+, (16) Hg2+, (17) Cu2+, (18) Fe3+. | |
Moreover, to check the sensing sensitivity of CP-1 towards Zn2+, the following experiment was carried out. The sample of CP-1 was dispersed in water (0.5 mg mL−1), and executed with an ultrasonic treatment to obtain a suspension. Then, different volumes of Zn2+ ions (0.1 M) were added to the above suspension, and the emission spectra were determined (Fig. 3a). With incremental addition of Zn2+, the emission intensities gradually increased. The association constant was calculated to be 1.812 × 104 mol−1 based on the fitted linear equation I/I0 = 1.289 + 1.812 × 104 [M], where I0 and I are fluorescence intensities before and after analyte incorporation, respectively, and [M] represents the concentration of the analyte (Fig. 3b). The limit of detection (LOD) for Zn2+ was 4.172 × 10−4 M. To the best of our knowledge, the reported sensors for Zn2+ are mostly based on organic molecules or composite materials,54–56 and CP-based fluorescent sensors for detecting Zn2+ ions are very rare.
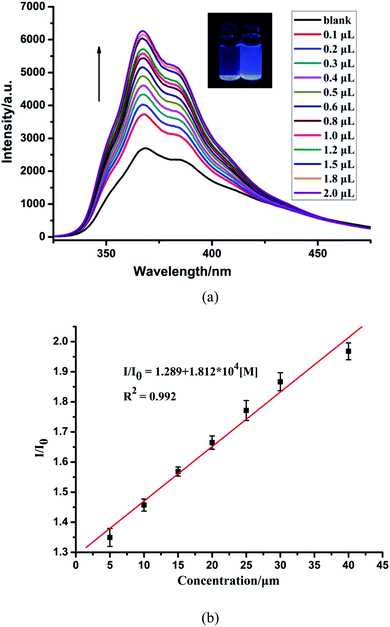 |
| Fig. 3 (a) Fluorescence spectra of CP-1 dispersed in an aqueous suspension upon the incremental addition of Zn2+ ions. (b) The linear correlation for the plot of I/I0 vs. the concentration of Zn2+. | |
The sensing sensitivities of CP-1 for Fe3+ ions were also checked with the same method as in case of Zn2+. The increasing concentration of Fe3+ resulted in a gradual decrease in the fluorescent intensity of CP-1 (Fig. 4a). The quenching efficiency was estimated through the equation: I0/I = 1.159 + 4.959 × 104 [M] (Fig. 4b). The quenching coefficient was calculated as 4.959 × 104 mol−1 for Fe3+. The LOD was 1.524 × 10−4 M for Fe3+.
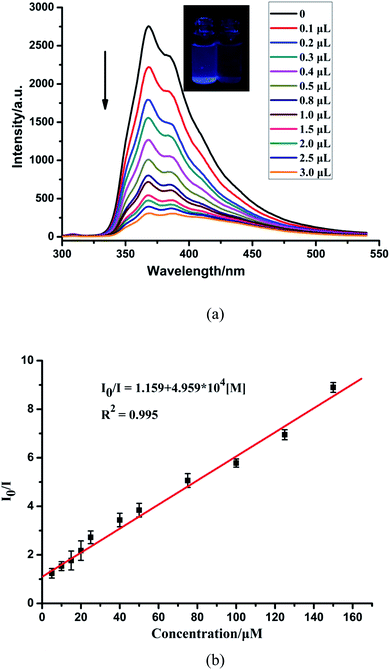 |
| Fig. 4 (a) Fluorescence spectra of CP-1 dispersed in an aqueous suspension upon the incremental addition of Fe3+ ions. (b) The linear correlation for the plot of I0/I vs. the concentration of Fe3+. | |
Sensing of anions
Simultaneously, to check the effects of anions on the luminescence intensity of CP-1, numerous anions (F−, Cl−, Br−, I−, NO3−, CH3COO−, SCN−, ClO4−, H2PO4−, SO32−, SO42−, and Cr2O72−) were selected. 1 mg of CP-1 was dispersed in aqueous solutions containing above anions (2 mL, 10−3 M). Obviously, Cr2O72− ions quenched the emission of CP-1, while other anions almost led to a little change in the fluorescence intensity of CP-1, indicating the potential detection of CP-1 towards Cr2O72− (Fig. 5a). Then, the sensing selectivity towards Cr2O72− was explored through competition experiments. Upon adding Cr2O72− into the mixture of CP-1 and each of other anions, the emission for each case was quenched greatly, showing the high selectivity of CP-1 towards Cr2O72− even in the presence of other interfering anions (Fig. 5b).
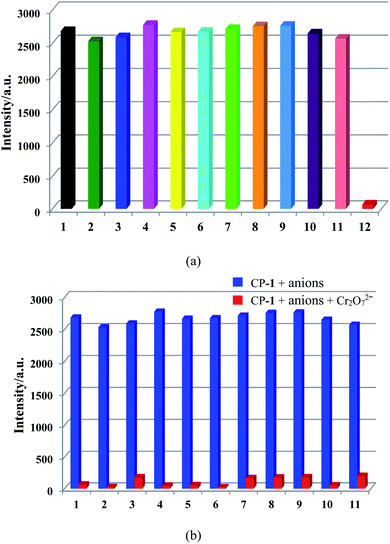 |
| Fig. 5 Luminescence intensity histograms of CP-1 (1 mg) dispersed into various anion aqueous solutions (2 mL, 10−3 M) excited at 278 nm (a) and subsequent addition of Cr2O72− (b). (1) Blank, (2) F−, (3) Cl−, (4) Br−, (5) I−, (6) CH3COO−, (7) SO42−, (8) H2PO4−, (9) ClO4−, (10) SO32−, (11) SCN−, (12) Cr2O72− (b). | |
To further investigate the sensing capability of CP-1 towards Cr2O72−, fluorescence titrations were conducted through the addition of different volumes of the Cr2O72− (0.1 M) aqueous solution to the suspension of CP-1. The emission intensity of CP-1 was gradually quenched with incrementally adding Cr2O72− (Fig. 6a). The quenching equation could be written as I0/I = 0.879 + 1.775 × 104 [M] (Fig. 6b). The quenching coefficient was calculated as 1.793 × 104 M−1. The LOD was calculated to be 4.21 × 10−4 M.
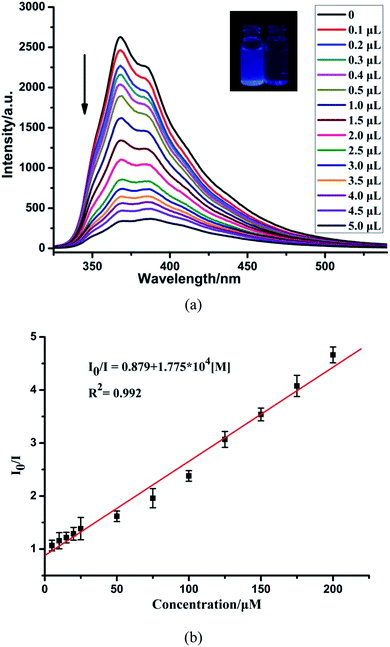 |
| Fig. 6 (a) Fluorescence spectra of CP-1 dispersed in an aqueous suspension upon the incremental addition of Cr2O72− ions. (b) The linear correlation for the plot of I0/I vs. the concentration of Cr2O72−. | |
The mechanism of luminescence sensing
To elucidate the reasons for the enhancement caused by Zn2+, and the quenching caused by Fe3+ and Cr2O72−, the sensing mechanisms were also investigated. First, the samples were immersed in the aqueous solutions with numerous analytes for 16 h. Then, the PXRD patterns were recorded to examine the stability of the structure. As confirmed by the PXRD patterns (Fig. S5†), the whole skeleton of CP-1 remained unchanged after immersed in the Zn2+ aqueous solution. In the structure of CP-1, there were uncoordinated Lewis basic N and S atoms from ligand H2btic. Therefore, the hypothesis was that the uncoordinated N and S atoms possibly coordinated to Zn2+ ions, which increased the delocalization of CP-1 and improved the energy-transfer efficiency from ligand to metal ions, and finally enhanced the whole fluorescence intensity. To further prove the hypothesis, the XPS of N 1s and S 2p were carried out on CP-1 and Zn2+@CP-1 (Fig. S6†). The N 1s peak at 398.65 eV in CP-1 was shifted to 398.75 eV, while the S 2p peak at 163.45 eV in CP-1 shifted to 163.3 eV after Zn2+ incorporation, indicating the formation of weak bonds between S/N and Zn2+. The formation of weak bonds caused the efficient energy transfer between ligand and metal ions, which further led to the fluorescence enhancement.
After immersed in Fe3+ and Cr2O72− aqueous solution, the PXRD patterns showed that the skeleton of CP-1 also did not change, indicating that the fluorescence quenching was unrelated to the structure of CP-1 (Fig. S7 and S8†). Then, the energy transfer mechanism was checked through the measurement of UV-vis spectra of Fe3+ and Cr2O72−, and the emission spectrum of CP-1, which depended upon the degree of overlap of the two kinds of spectra. As shown in Fig. S9 and S10,† the spectrum of CP-1 partly overlapped with absorption spectra of Fe3+ and Cr2O72−, while other metal ions or anions displayed no spectral overlap. Therefore, the energy transfer mechanism could account for the luminescence quenching effects induced by Fe3+ and Cr2O72− ions.
Conclusions
In summary, we synthesized a Cd(II) coordination polymer with a chain structure (CP-1) based on the mixed-ligand method under solvothermal conditions. The excellent characteristics of high chemical stability, intense fluorescence, and the uncoordinated Lewis basic N and S atoms in the structure of CP-1 made it a rare multi-responsive fluorescence sensor to detect Zn2+, Fe3+ and Cr2O72− with fluorescence enhancement or quenching. This study also demonstrates that the introduction of functional groups or atoms in the ligands can endow the CPs with expected properties.
Conflicts of interest
There are no conflicts to declare.
Acknowledgements
The work was supported by the Natural Science Foundation of Hebei Province (No. B2018201226), the Science and Technology Project of Hebei Education Department (No. ZD2018027), the Youth Top Talent Project of Hebei Province and Key Laboratory of Public Health Safety of Hebei Province.
Notes and references
- Z. S. Zang, Y. M. Xu and A. T. Y. Lau, Toxicol. Res., 2016, 5, 987–1002 CrossRef CAS PubMed.
- Y. Mikata, Y. Nodomi, A. Kizu and H. Konno, Dalton Trans., 2014, 43, 1684–1690 RSC.
- Z. Xu, J. Yoon and D. R. Spring, Chem. Soc. Rev., 2010, 39, 1996–2006 RSC.
- X. F. Liu and E. C. Theil, Acc. Chem. Res., 2005, 38, 167–175 CrossRef CAS PubMed.
- L. M. Hyman and K. J. Franz, Coord. Chem. Rev., 2012, 256, 2333–2356 CrossRef CAS PubMed.
- M. L. Zastrow, R. J. Radford, W. Chyan, C. T. Anderson, D. Zhang, A. Loas, T. Tzounopoulos and S. J. Lippard, ACS Sens., 2016, 1, 32–39 CrossRef CAS PubMed.
- X. C. Shen, S. Ma, H. Xia, Z. Shi, Y. Mu and X. M. Liu, J. Mater. Chem. A, 2018, 6, 20653–20658 RSC.
- J. M. Liu, Y. Ye, X. D. Sun, B. Liu, G. H. Li, Z. Q. Liang and Y. L. Liu, J. Mater. Chem. A, 2019, 7, 16833–16841 RSC.
- X. Wang, Y. Han, X. X. Han, X. Y. Hou, J. J. Wang and F. Fu, New J. Chem., 2018, 42, 19844–19852 RSC.
- D. Yildiz, I. Kula and N. Şahin, Eurasian J. Anal. Chem., 2013, 8, 112–122 Search PubMed.
- A. Ohashi, H. Ito, C. Kanai, H. Imura and K. Ohashi, Talanta, 2005, 65, 525–530 CrossRef CAS PubMed.
- Z. M. Sun and P. Liang, Microchim. Acta, 2008, 162, 121–125 CrossRef CAS.
- P. Singh, A. K. Singh and A. K. Jain, Electrochim. Acta, 2011, 56, 5386–5395 CrossRef CAS.
- S. Caprara, L. M. Laglera and D. Monticelli, Anal. Chem., 2015, 87, 6357–6363 CrossRef CAS PubMed.
- D. J. Lin, J. Wu, M. Wang, F. Yan and H. X. Ju, Anal. Chem., 2012, 84, 3662–3668 CrossRef CAS PubMed.
- L. Moens, P. Verrept, R. Dams, U. Greb, G. Jung and B. Laser, J. Anal. At. Spectrom., 1994, 9, 1075–1078 RSC.
- D. V. Biller and K. W. Bruland, Mar. Chem., 2012, 130–131, 12–20 CrossRef CAS.
- F. Séby, S. Charles, M. Gagean, H. Garraud and O. Donard, J. Anal. At. Spectrom., 2003, 18, 1386–1390 RSC.
- S. R. Batten, N. R. Champness, X. M. Chen, J. Garcia-Martinez, S. Kitagawa, L. Öhrström, M. O'Keeffe, M. P. Suh and J. Reedijk, Pure Appl. Chem., 2013, 85, 1715–1724 CAS.
- X. Y. Liu, L. Sun, H. L. Zhou, P. P. Cen, X. Y. Jin, G. Xie, S. P. Chen and Q. L. Hu, Inorg. Chem., 2015, 54, 8884–8886 CrossRef CAS PubMed.
- J. W. Zhang, M. C. Hu, S. N. Li, Y. C. Jiang, P. Qu and Q. G. Zhai, Chem. Commun., 2018, 54, 2012–2015 RSC.
- D. S. Li, Y. Wu, J. Zhao, J. Zhang and J. Lu, Coord. Chem. Rev., 2014, 261, 1–27 CrossRef CAS.
- A. Pankajakshan, M. Sinha, A. A. Ojha, S. Manda and W. S. Nanoscale, ACS Omega, 2018, 3, 7832–7839 CrossRef CAS PubMed.
- X. Y. Liu, F. F. Li, X. H. Ma, P. P. Cen, S. C. Luo, Q. Shi, S. R. Ma, Y. W. Wu, C. C. Zhang, G. Xie and S. P. Chen, Dalton Trans., 2017, 46, 1207–1217 RSC.
- X. H. Ma, Y. R. Liu, W. M. Song, Z. Wang, X. Y. Liu, G. Xie, S. P. Chen and S. L. Gao, Dalton Trans., 2018, 47, 12092–12104 RSC.
- R. W. Huang, Y. S. Wei, X. Y. Dong, X. H. Wu, C. X. Du, S. Q. Zang and T. C. W. Mak, Nat. Chem., 2017, 9, 689–697 CrossRef CAS PubMed.
- F. L. Li, Q. Shao, X. Q. Huang and J. P. Lang, Angew. Chem., Int. Ed., 2018, 57, 1888–1892 CrossRef CAS PubMed.
- C. Y. Sun, C. Qin, C. G. Wang, Z. M. Su, S. Wang, X. L. Wang, G. S. Yang, K. Z. Shao, Y. Q. Lan and E. B. Wang, Adv. Mater., 2011, 23, 5629–5632 CrossRef CAS PubMed.
- N. D. Rudd, H. Wang, E. M. Fuentes-Fernandez, S. J. Teat, F. Chen, G. Hall, Y. J. Chabal and J. Li, ACS Appl. Mater. Interfaces, 2016, 8, 30294–30303 CrossRef CAS PubMed.
- F. L. Hu, Z. Y. Di, M. Y. Wu, M. C. Hong and J. Li, Cryst. Growth Des., 2019, 19, 6381–6387 CrossRef CAS.
- X. Lian and B. Yan, Dalton Trans., 2016, 45, 18668–18675 RSC.
- L. H. Liu, X. T. Qiu, Y. J. Wang, Q. Shi, Y. Q. Sun and Y. P. Chen, Dalton Trans., 2017, 46, 12106–12113 RSC.
- C. Wang, L. Tian, W. Zhu, S. Wang, P. Wang, Y. Liang, W. Zhang, H. Zhao and G. Li, ACS Appl. Mater. Interfaces, 2017, 9, 20076–20085 CrossRef CAS PubMed.
- B. X. Dong, Y. M. Pan, W. L. Liu and Y. L. Teng, Cryst. Growth Des., 2018, 18, 431–440 CrossRef CAS.
- H. J. Zhang, R. Q. Fan, W. Chen, J. Z. Fan, Y. W. Dong, Y. Song, X. Du, P. Wang and Y. L. Yang, Cryst. Growth Des., 2016, 16, 5429–5440 CrossRef CAS.
- C. Q. Zhang, L. B. Sun, Y. Yan, J. Y. Li, X. W. Song, Y. L. Liu and Z. Q. Liang, Dalton Trans., 2015, 44, 230–236 RSC.
- N. Xu, Q. H. Zhang, B. S. Hou, Q. Cheng and G. A. Zhang, Inorg. Chem., 2018, 57, 13330–13340 CrossRef CAS PubMed.
- J. Zhao, Y. N. Wang, W. W. Dong, Y. P. Wu, D. S. Li and Q. C. Zhang, Inorg. Chem., 2016, 55, 3265–3271 CrossRef CAS PubMed.
- C. H. Chen, X. S. Wang, L. Li, Y. B. Huang and R. Cao, Dalton Trans., 2018, 47, 3452–3458 RSC.
- X. S. Zeng, H. L. Xu, Y. C. Xu, X. Q. Li, Z. Y. Nie, S. Z. Gao and D. R. Xiao, Inorg. Chem. Front., 2018, 5, 1622–1632 RSC.
- N. Xu, Q. H. Zhang and G. A. Zhang, Dalton Trans., 2019, 48, 2683–2691 RSC.
- S. S. Zhao, J. Yang, Y. Y. Liu and J. F. Ma, Inorg. Chem., 2016, 55, 2261–2273 CrossRef CAS PubMed.
- B. Gole, A. K. Bar and P. S. Mukherjee, Chem.–Eur. J., 2014, 20, 13321–13336 CrossRef CAS PubMed.
- A. Khatun, D. K. Panda, N. Sayresmith, M. G. Walter and S. Saha, Inorg. Chem., 2019, 58, 12707–12715 CrossRef CAS PubMed.
- Y. J. Mu, Y. G. Ran, B. B. Zhang, J. L. Du, C. Y. Jiang and J. Du, Cryst. Growth Des., 2020, 20, 6030–6043 CrossRef CAS.
- A. E. Lozano, J. G. de la Campa, J. de Abajo and J. Preston, Polymer, 1994, 35, 872–877 CrossRef CAS.
- G. M. Sheldrick, Acta Crystallogr., Sect. A: Found. Crystallogr., 2008, 64, 112–122 CrossRef CAS PubMed.
- S. Y. Liu, M. M. Guo, H. D. Guo, Y. Y. Sun, X. M. Guo, S. W. Sun and E. V. Alexandrov, RSC Adv., 2018, 8, 4039–4048 RSC.
- D. K. Singha, P. Majee, S. K. Monda and P. Mahata, ChemistrySelect, 2017, 2, 5760–5768 CrossRef CAS.
- F. Li, M. L. Sun, X. Zhang and Y. G. Yao, Cryst. Growth Des., 2019, 19, 4404–4416 CrossRef CAS.
- L. Wen, Z. Lu, J. Lin, Z. Tian, H. Zhu and Q. Meng, Cryst. Growth Des., 2007, 7, 93–99 CrossRef CAS.
- H. Y. Bai, J. F. Ma, J. Yang, Y. Y. Liu, H. Wu and J. C. Ma, Cryst. Growth Des., 2010, 10, 995–1016 CrossRef CAS.
- J. G. Lin, S. Q. Zang, Z. F. Tian, Y. Z. Li, Y. Y. Xu, H. Z. Zhu and Q. J. Meng, CrystEngComm, 2007, 9, 915–921 RSC.
- J. Jiang, H. E. Jiang, X. L. Tang, L. Z. Yang, W. Dou, W. S. Liu, R. Fang and W. Liu, Dalton Trans., 2011, 40, 6367–6370 RSC.
- Z. X. Li, L. F. Zhang, L. N. Wang, Y. K. Guo, L. H. Cai, M. M. Yu and L. H. Wei, Chem. Commun., 2011, 47, 5798–5800 RSC.
- Y. Y. Li, X. Hu, X. D. Zhang, H. Y. Cao and Y. M. Huang, Anal. Chim. Acta, 2018, 1024, 145–152 CrossRef CAS PubMed.
Footnote |
† Electronic supplementary information (ESI) available: Crystallographic parameters for CP-1, powder XRD, TGA, photoluminescences and related spectra. CCDC reference number 2045504 for CP-1. For ESI and crystallographic data in CIF or other electronic format see DOI: 10.1039/d0ra10203b |
|
This journal is © The Royal Society of Chemistry 2021 |
Click here to see how this site uses Cookies. View our privacy policy here.