DOI:
10.1039/D1RA01382C
(Paper)
RSC Adv., 2021,
11, 15921-15926
Preparation and dye adsorption properties of an oxygen-rich porous organic polymer†
Received
20th February 2021
, Accepted 23rd April 2021
First published on 29th April 2021
Abstract
Porous organic polymers (POPs), allowing fine synthetic control over their chemical structures, have shown great promise for addressing environmental issues. The high specific surface area and abundant porous structures of POPs can provide large storage space to adsorb dye molecules. Meanwhile, the introduction of polar groups, such as oxygen-containing functional groups in POPs, can not only improve the hydrophilicity, but also provide a strong interaction with dye molecules, thereby improving their adsorption performance. In this paper, an oxygen-rich porous polymer, POP-O, containing polar carbonyl and hydroxyl groups, was prepared by Sonogashira–Hagihara cross-coupling polycondensation. The characteristic results show that POP-O exhibits a hierarchical pore structure with a high specific surface area of 619 m2 g−1. The combination of abundant polar functional groups and high porosity endows POP-O with decent dye adsorption performance, and its theoretical maximum adsorption capacity for Rhodamine B (Rh B) is calculated to be 1012 mg g−1.
Introduction
Organic dyes in wastewater from paper printing, textile dyeing, and plastic and food industries are one of the main sources of water pollution,1,2 and they are highly hazardous to the aquatic environment and potentially damaging to human health. Adsorption is the most direct and effective way to remove contaminants from water.3,4 Many porous materials with high specific surface area and rich porous structures such as activated carbons,5,6 zeolites7,8 and porous organic polymers (POPs),9–11 have been used as adsorbents to remove organic dyes from wastewater. Among them, POPs are attracting more and more attention because of their unique advantages, such as structural diversity, adjustable porosity and ease of functionalization.
In order to achieve high adsorption capacity and selectivity for specific organic substances, tailor-designed functional moieties are often introduced into the porous polymer structures.12–14 Generally, there are two methods to introduce special functional groups into the porous polymers and the first one is the post-functionalization method.15 For example, Fang et al. synthesized polyarylether-based covalent organic frameworks (COFs) with carboxyl or amino groups being functionalized through post-modification process, achieving the removal of antibiotics from water over a wide pH range.16 Song et al. prepared cationic conjugated microporous polymers (CMPs) by post-polymerization functionalization and found that the introduction of cations was beneficial to increasing the adsorption rate and amount of anionic dyes such as Congo red and methyl orange.17 However, the degree of post-functionalization reaction is usually low and it may cause the damage of the polymer structure during the post-modification process. The other functionalization way is the bottom-up method,18 which involves the direct polymerization of the functional group containing monomers. This method could accurately control the amounts and locations of functional groups without damaging the structure of POPs. Some special functional groups such as hydroxyl (–OH),19 amino (–NH2),20 carboxyl (–COOH)21 and sulfonic acid (–SO3H) groups22 have been introduced into POPs through the bottom-up method and the functionalized POPs have been extensively used in the field of dye adsorption.
Among these functional groups, oxygen-containing functional groups could bring about some special properties, when they are incorporated into POPs.23,24 Firstly, the presence of oxygen-containing groups can increase hydrophilicity of the POPs and thus expand the contact area between POPs and the dye molecules in water. Also, the oxygen-rich units could make the functionalized POPs more electronegative, affording additional electrostatic interactions with cationic dye molecules.25 Cooper et al. found that the introduction of hydrophilic hydroxyl groups can improve the dye adsorption capacity of CMPs.19 Liu et al. prepared a novel triterpene and crown ether-based porous copolymer POP-TCE-15, and found that there was electrostatic interaction between the lone pair of electrons on the O atom of crown-ether-15 and the positively charged cationic dye methylene blue.26 Although recent studies have shown that introducing oxygen-containing groups could display significant effects on the adsorption performance of the POPs, the synergistic effect between different oxygen-containing functional groups still needs to be studied. Based on the above considerations, a novel oxygen-rich POP, containing polar carbonyl and hydroxyl groups, was synthesized via Sonogashira–Hagihara cross-coupling polycondensation. The effects of pore structure and polar groups on the adsorption performance were discussed. Density functional theoretical (DFT) calculation revealed that the carbonyl and hydroxyl groups of POP-O ensured high binding energy toward Rh B molecules.
Experimental
Materials
2,5-Dichloro-3,6-dihydroxy-p-benzoquinone was purchased from TCI, trimethylsilyl acetylene, 1,4-dibromobenzene, 1,3,5-tribromobenzene, tetrakis(triphenylphosphine)palladium (Pd(PPh3)4), copper(I) iodide (CuI), trimethylamine (TEA), and N,N-dimethylformamide (DMF) were all purchased from Adamas. 1,3,5-Triethynylbenzene was synthesized by previously reported literature methods.27 The NMR spectrum of 1,3,5-triethynylbenzene is shown in Fig. S1.†
Synthesis of polymers
Experimentally, 1,3,5-triethynylbenzene (150 mg, 1 mmol), 2,5-dichloro-3,6-dihydroxy-p-benzoquinone (209 mg, 1 mmol), Pd(PPh3)4 (58 mg, 0.05 mmol) and CuI (19 mg, 0.1 mmol), were dissolved in the mixture of DMF (20.0 mL) and TEA (20.0 mL). The reaction mixture was heated to 80 °C and stirred for 72 h under argon atmosphere. Then deionized water was added into the resultant solution after it was cooled down to room temperature. The polymer collected by filtration was washed thoroughly with water, methanol, dichloromethane and acetone. Further purification of the polymer was achieved by Soxhlet extraction with methanol, chloroform and tetrahydrofuran. The product was dried in vacuum for 24 h at 80 °C and POP-O was obtained in 72% yield as a brown solid.
For comparison, a structural analogue polymer without oxygen-containing polar groups, POP-B, was synthesized (Scheme S1†) according to the literature.19
Characterization
Fourier transformed infrared (FT-IR) spectra were recorded in transmission mode on a Bruker VERTEX 70 FT-IR spectrometer. 13C CP-MAS (cross-polarization magic-angle-spinning) solid-state NMR was recorded on BRUKER AVANCE III HD 400 MHz NMR spectrometer. Elemental analysis was measured by Elementer Vario EL elemental analyzer. The thermal stability of POP-O was evaluated by thermogravimetric analysis (TGA) under N2 atmosphere with a heating rate of 5 °C min−1 and over the temperature range of 30–800 °C. X-ray diffraction (XRD) was carried out on Philips X' Pert Pro. Scanning electron microscopy (SEM) images of POP-O were obtained by the Nova Nano SEM 450 scanning electro-microscope. Transmission electron microscopy (TEM) images of the POP-O were performed on the Tecnai G2 F30 (FEI Holland) transmission electron microscope. Surface area and pore size distributions (PSDs) (77 K) were measured by using Belsorp-Max Automatic Micropore Adsorption Analyzer. Before analysis, the sample was degassed in a vacuum at 120 °C for 12 h. The surface area was calculated based on nitrogen adsorption isotherms by Brunauer–Emmett–Teller (BET). Ultraviolet-visible spectra were measured on a UV-vis-NIR spectrophotometer (UV-3600) at room temperature, over the wavelength range of 400–800 nm. The particle size distribution and zeta potential were measured by the Nano-ZS Malvern instrument.
Dye adsorption measurement
Experimental operation: at a temperature of 298 K, 10 mg of the polymer was weighed into multiple glass bottles containing 20 mL of Rh B solution at various concentrations, and the mixture was agitated at 180 rpm for different time intervals. After adsorption, the polymer is filtered and the absorbance of the remaining solution is measured by UV-vis spectra to obtain the resultant dye concentration (characteristic absorbance peak of Rh B is 554 nm).
The calculation formula of the adsorption capacity of the polymer to Rh B is:
Among them,
C0 is the initial concentration of Rh B aqueous solution (mg L
−1);
Ct is the concentration of Rh B in the solution in
t hour (mg L
−1);
V is the volume of Rh B aqueous solution (L);
m is the mass of polymer (g).
The Langmuir and Freundlich models were used to fit the adsorption isotherm. Rh B aqueous solutions with initial concentrations of 214, 276, 328, 481, 498, 546 and 663 mg L−1 were selected for testing, and the sampling time was 18 h.
The Langmuir equation is:
The Freundlich equation is:
Among them,
qm is the maximum adsorption capacity of Rh B by polymer (mg g
−1);
Ce is the equilibrium concentration (mg L
−1);
KL is the Langmuir constant;
KF is the Freundlich constant; and 1/
n is the adsorption index.
A pseudo-first-order28 kinetic model and pseudo-second-order kinetic model29 were used to fit the kinetic process of polymer adsorption of Rh B.
The formula of the pseudo-first-order kinetic model is:
The formula of the pseudo-first-order kinetic model is:
Among them,
qe is the equilibrium adsorption capacity of Rh B by polymer (mg g
−1);
qt is the adsorption capacity of Rh B by polymer in
t hour (mg g
−1);
k1 is the pseudo-first-order fitting constant;
k2 is pseudo-second-order fitting constant;
t is time (h).
Results and discussion
Synthesis and characterization
The POP-O was synthesized by Sonogashira–Hagihara cross-coupling polycondensation according to the procedure shown in Scheme 1. The monomer 1,3,5-triethynylbenzene with three functionalities is used as the strut to construct the network structure and the comonomer 2,5-dichloro-3,6-dihydroxy-p-benzoquinone containing polar carbonyl and hydroxyl groups, is chosen as the oxygen-rich moiety. Due to the crosslinked skeleton, the obtained POP-O was insoluble in common organic solvents such as dichloromethane, tetrahydrofuran, methanol, acetone, N,N-dimethylformamide. The chemical structure of POP-O is confirmed by Fourier transform infrared (FT-IR) spectroscopy and solid-state 13C CP-MAS NMR. In the FT-IR spectra (Fig. S2†), the C–Cl absorption of POP-O at 1263 cm−1 almost disappear after the polycondensation, indicating high polymerization efficiency. FT-IR spectra also show characteristic absorbance bands at 1660 cm−1 and 3290 cm−1 corresponding to the C
O and O–H stretching vibrations, respectively, indicating the presence of carbonyl and hydroxyl groups. Moreover, solid state 13C CP-MAS NMR spectra of POP-O (Fig. S3†) shows that aromatic carbon displays in broad peaks from 110 to 150 ppm and two characteristic broad peaks of ethynyl groups could be found at 80 to 90 ppm.30 Meanwhile, the elemental analysis of the polymer shows that the mass fractions of carbon atoms and hydrogen atoms in POP-O are 68.86% and 2.1% respectively, which are basically consistent with the theoretical content, further confirming the chemical structure of the POP-O.
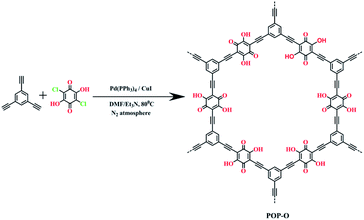 |
| Scheme 1 Synthetic route of the polymer POP-O. | |
Thermogravimetric analysis curve (TGA) of POP-O is shown in Fig. S4.† The first weight loss (10.9%) stage of POP-O was around 210 °C, which could be assigned to the weight loss of the adsorbed solvent. The following weight loss of the polymer could be further attributed to the decomposition of the framework. Because there is a certain amount of hydroxyl groups in the polymer structure, compared with other POPs, the thermal stability of the POP-O is slightly inferior.31–33 The powder X-ray diffraction pattern of POP-O shows a broad peak in the range of 5–35° without obvious sharp peaks (Fig. S5†), indicating the amorphous structure of POP-O. The morphology of polymer is investigated by scanning electron microscopy (SEM) and transmission electron microscopy (TEM). It could be observed that the POP-O manifests as irregular particle accumulation (Fig. 1a–c). As can be seen from the TEM images (Fig. 1d–f), POP-O exhibits as flake shape. These particles assembled from flakes can help improve the contact area between the polymer and the organic dye.34 In order to understand the hydrophilic property of the material, the contact angle between POP-O and water is tested (Fig. S6†). POP-O shows a contact angle with water of 60°, while POP-B exhibits a contact angle with water of 109°, indicating that the oxygen-containing functional groups could improve the hydrophilic property of the polymer. Decent hydrophilicity makes POP-O easier to form a uniform dispersion in aqueous solution, thereby helping to improve the adsorption performance.
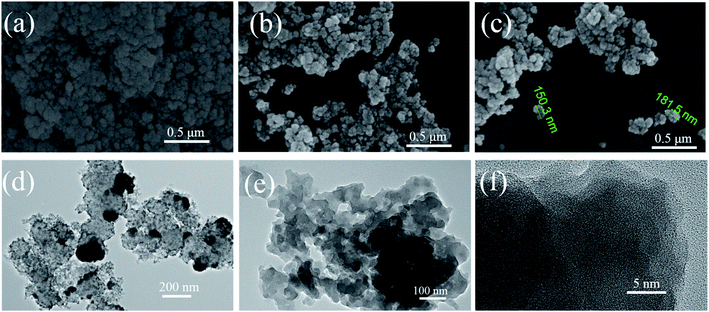 |
| Fig. 1 (a–c) Scanning electron microscopy (SEM) images of POP-O; (d–f) transmission electron microscopy (TEM) images of POP-O. | |
Porosity of POP-O
The porosity of POP-O is measured by nitrogen adsorption and desorption experiments at 77 K. As shown in Fig. 2a, POP-O shows a combination of Type I and Type IV sorption isotherms with a hysteresis loop. The specific surface area of the POP-O is calculated to be 619 m2 g−1 by the BET model. The pore size distribution of POP-O is shown in Fig. 2b, which is obtained from the nonlocalized density functional theory (NLDFT). POP-O mainly has micropores around 0.9 nm and mesopores around 2.2 nm, and there are also some mesopores at 5–40 nm. The results indicate that there is a hierarchical pore structure in the polymer. This hierarchical pore structure combines the advantages of micropores and mesopores, which could improve the mass transfer rate of the material and facilitate the entry of dye molecules into POP-O, thereby increasing its application potential in the field of adsorption.
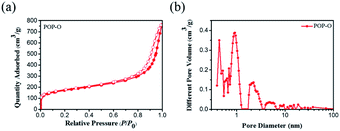 |
| Fig. 2 Nitrogen adsorption–desorption isotherm (a) and pore size distribution (b) of the polymer POP-O. | |
Particle size and zeta potential
The adsorption performance of the polymer is not only related to its porosity, but also has an important relationship with the particle size in the solution.35,36 10 mg of the polymer was sonicated in 20 mL of aqueous solution for 5 minutes, and then its particle size was measured through light scattering method. As shown in Fig. S7,† the particles of POP-O have two size distributions of 1000–1700 nm and 100–180 nm, which is consistent with the observation result of the SEM images. The large-sized particles are formed from the aggregation of small-sized nanoparticles. Meanwhile, the particle size distribution of POP-B only shows one peak centered at 1414 nm. Smaller particle size of POP-O is beneficial for the adsorption of dye molecules. To further characterize the stability of the colloidal dispersions, zeta potential of the obtained polymers were measured. It is found that POP-O shows a zeta potential value of −27.4 mV, while the zeta potential value of POP-B is −12.7 mV, indicating higher stability of the POP-O dispersion.
Dye adsorption properties of POP-O
With large amounts of oxygen-rich groups and hierarchical pore structure, POP-O could potentially possess good adsorption capabilities for organic dye pollutants from water. We used Rhodamine B (Rh B) as the adsorbate to study the adsorption properties of POP-O for water-soluble pollutants. Fast kinetic adsorption capacity is one of the key factors for adsorbent, which directly determines the actual adsorption efficiency of the adsorbent. Adsorption of Rh B solution at 25 °C with an initial concentration of 481 mg L−1 is shown in Fig. 3a. The adsorption amounts of Rh B by POP-O increases rapidly within the first hour, and then gradually reaches equilibrium (Table S1†). The POP-O displayed an absorption of 590 mg g−1 at equilibrium, and the adsorption capacity of the comparative analogue polymer POP-B is 390 mg g−1 in equilibrium. The experimental data of the Rh B adsorption onto POP-O is fitted to the Langmuir and Freundlich adsorption isotherm models respectively (Fig. S8a, b and Tables S2, S3†). It can be seen that the adsorption of Rh B by POP-O is more in line with the Langmuir isotherm adsorption equation (R2 = 0.99367) than Freundlich isotherm adsorption equation (R2 = 0.98591), indicating that the Rh B adsorption onto POP-O is probably in a monomolecular-layer manner.7 The maximum adsorption capacity of POP-O toward Rh B can thus be calculated through Langmuir equation to be 1012 mg g−1, which is better than the adsorption capacity of many organic porous materials that have been reported so far, as shown in Table 1. The excellent adsorption capacity of POP-O for Rh B is mainly attributed to the following reasons. Firstly, due to the presence of hydroxyl and carbonyl groups, the POP-O has good hydrophilicity, which make POP-O easier to form a uniform dispersion in Rh B aqueous solution. Secondly, the oxygen-rich units make POP-O electronegative, which allow additionally strong electrostatic interactions with cationic dye molecules (Rh B). The last but not least, the large specific surface area of POP-O provides a large storage space for the adsorption of dye molecules. The schematic adsorption process is shown in Fig. S9†. When POP-O is added to the aqueous solution of Rh B, the electronegative POP-O interacts with Rh B electrostatically. Meanwhile, the hierarchical pore structure is also beneficial to the adsorption of Rh B.
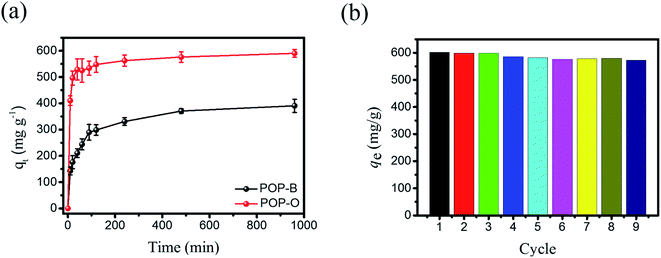 |
| Fig. 3 (a) The adsorption capacity of Rh B by polymers over time; (b) recyclability of POP-O for the Rh B adsorption. | |
Table 1 Comparison of the maximum equilibrium adsorption capacity of different porous polymers towards Rh B at room temperature
Adsorbents |
qmax (mg g−1) of Rh B |
Ref. |
HJ-1 |
73.5 |
37 |
CoOF |
72.15 |
38 |
SA-MMNPs |
216 |
39 |
PDVB-VI |
260.42 |
40 |
C-NSANaphHCP@Br |
142 |
41 |
POP-TCE-15 |
421.9 |
26 |
CuP-DMNDA-COF/Fe |
424 |
10 |
h-COP-P |
460 |
42 |
DPT-HPP |
256.40 |
43 |
CTF-CTTD |
684.9 |
44 |
Noria-POP-1 |
855 |
45 |
HCPs-5% |
1400 |
46 |
AzoPPOP |
1357.58 |
47 |
POP-O |
1012 |
This work |
Pseudo-first-order and pseudo-second-order kinetic models are employed to fit the adsorption kinetic data (Fig. S10a, b and Table S4†), and the corresponding fitting parameters in Table S4† show that the pseudo-second-order kinetic model can better describe the kinetic data due to the higher correlation coefficient (R2 = 0.99983). The results indicate that dye adsorption process appears to be controlled by the chemisorption process involving covalent forces through sharing or exchange of electrons between surface functional groups of POP-O and the positive charged of Rh B.
Stability and recycling are very important for POPs to be used as an adsorbent for industrial applications. The recyclability of POP-O for adsorption of Rh B was evaluated for nine cycles under the same condition. Before each run, the used POP-O was washed several times in ethanol until the colour of the filtrate became clear. The adsorption capacity of the POP-O in the nine adsorption cycles is shown in Fig. 3b. No loss of Rh B adsorption capacity is observed, indicating that POP-O has good adsorption stability and recyclability. Meanwhile, the SEM images, FT-IR spectra and PXRD patterns of POP-O were tested after the cycles, and found that there were no significant changes (Fig. S11–S13†).
To clarify the possible mechanism of the adsorption process, the binding energies (BEs, BE = Etotal − Esegment − ERh B)48 between the Rh B molecule and polymer segments are calculated by density functional theory (DFT) using Materials Studio software with the GGA-PBE basis set as shown in Fig. 4. The other three scaffolds only containing carbonyl or hydroxyl or neither were selected as model systems for comparison. After the optimization of all the moieties and their complexes, a difference in binding energies (BEs) is found where the POP-O segment shows the maximum BE toward Rh B with a value of −3.779 eV (Fig. 4). The results of the theoretical analysis show that the synergistic effect of different oxygen-containing functional groups in POP-O has a crucial influence on the Rh B adsorption ability. According to the experimental results and DFT calculations, it is suggested that the structural features of abundant oxygen-containing groups, high surface area, and hierarchical pore structures of POP-O work together to achieve excellent adsorption performance of Rh B dyes from aqueous solutions.
 |
| Fig. 4 Binding energies of Rh B molecule with the segments of polymers network (C, gray; N, blue; H, white; O, red; Cl, green) (a) the POP-O segment; (b) POP-O analogue with only carbonyl groups; (c) POP-O analogue with only hydroxyl groups; (d) POP-O analogue without carbonyl and hydroxyl groups. | |
Conclusions
In summary, an oxygen-rich POP(POP-O) was prepared through Sonogashira–Hagihara cross-coupling polycondensation using monomers containing carbonyl and hydroxyl groups. The introduction of polar groups in POP-O not only improves the hydrophilicity, which makes POP-O easier to form a uniform dispersion in Rh B aqueous solution, but also can make POP-O more electronegative, which allows additionally strong electrostatic interactions with Rh B. Meanwhile, the high specific surface area of POP-O and the presence of hierarchical pore structure can provide a large storage space to adsorb dye molecules. POP-O exhibits excellent dye adsorption performance, and theoretical maximum adsorption capacity for Rh B can reach 1012 mg g−1. This result indicates that through delicate structure design, functional POPs can be used as effective adsorbents for water treatment.
Conflicts of interest
There are no conflicts to declare.
Acknowledgements
This work is financially supported by National Natural Science Foundation of China (51973128) and Science and Technology Department of Sichuan Province (2019YJ0128).
References
- A. Alsbaiee, B. J. Smith, L. Xiao, Y. Ling, D. E. Helbling and W. R. Dichtel, Nature, 2016, 529, 190–194 CrossRef CAS PubMed.
- M. A. Shannon, P. W. Bohn, M. Elimelech, J. G. Georgiadis, B. J. Mariñas and A. M. Mayes, Nature, 2008, 452, 301–310 CrossRef CAS PubMed.
- J. Zhang, Y. Wang, D. Liang, Z. Xiao, Y. Xie and J. Li, Ind. Eng. Chem. Res., 2020, 59, 14531–14536 CrossRef CAS.
- X. Xie, X. Huang, W. Lin, Y. Chen, X. Lang, Y. Wang, L. Gao, H. Zhu and J. Chen, ACS Omega, 2020, 5, 13595–13600 CrossRef CAS PubMed.
- C. Patawat, K. Silakate, S. ChuanUdom, N. Supanchaiyamat, A. J. Hunt and Y. Ngernyen, RSC Adv., 2020, 10, 21082–21091 RSC.
- S. Moosavi, C. W. Lai, S. Gan, G. Zamiri, O. Akbarzadeh Pivehzhani and M. R. Johan, ACS Omega, 2020, 5, 20684–20697 CrossRef CAS PubMed.
- Y. Li, X. Yan, X. Hu, R. Feng and M. Zhou, Chem. Eng. J., 2019, 375, 122003 CrossRef CAS.
- W. Schwieger, A. G. Machoke, T. Weissenberger, A. Inayat, T. Selvam, M. Klumpp and A. Inayat, Chem. Soc. Rev., 2016, 45, 3353–3376 RSC.
- Y. Yuan, H. Huang, L. Chen and Y. Chen, Macromolecules, 2017, 50, 4993–5003 CrossRef CAS.
- Y. Hou, X. Zhang, C. Wang, D. Qi, Y. Gu, Z. Wang and J. Jiang, New J. Chem., 2017, 41, 6145–6151 RSC.
- D. Shetty, I. Jahovic, J. Raya, F. Ravaux, M. Jouiad, J. C. Olsen and A. Trabolsi, J. Mater. Chem. A, 2017, 5, 62–66 RSC.
- J. Wang, G. Wang, W. Wang, Z. Zhang, Z. Liu and Z. Hao, J. Mater. Chem. A, 2014, 2, 14028–14037 RSC.
- F. T. Costa, K. V. Jardim, A. F. Palomec-Garfias, P. R. Caceres-Velez, J. A. Chaker, A. Medeiros, S. E. Moya and M. H. Sousa, ACS Omega, 2019, 4, 5640–5649 CrossRef CAS PubMed.
- R. M. N. Kalla, M. R. Kim and I. Kim, Ind. Eng. Chem. Res., 2018, 57, 11583–11591 CrossRef CAS.
- W. Zhao, Y. Jiao, R. Gao, L. Wu, S. Cheng, Q. Zhuang, A. Xie and W. Dong, Chem. Eng. J., 2020, 391, 123591 CrossRef CAS.
- X. Guan, H. Li, Y. Ma, M. Xue, Q. Fang, Y. Yan, V. Valtchev and S. Qiu, Nat. Chem., 2019, 11, 587–594 CrossRef CAS PubMed.
- Y. Liu, Y. Cui, C. Zhang, J. Du, S. Wang, Y. Bai, Z. Liang and X. Song, Chem.–Eur. J., 2018, 24, 7480–7488 CrossRef CAS PubMed.
- N. Huang, L. Zhai, H. Xu and D. Jiang, J. Am. Chem. Soc., 2017, 139, 2428–2434 CrossRef CAS PubMed.
- R. Dawson, A. Laybourn, R. Clowes, Y. Z. Khimyak, D. J. Adams and A. I. Cooper, Macromolecules, 2009, 42, 8809–8816 CrossRef CAS.
- P. Su, X. Zhang, Z. Xu, G. Zhang, C. Shen and Q. Meng, New J. Chem., 2019, 43, 17267–17274 RSC.
- Y. Li, C. X. Yang, H. L. Qian, X. Zhao and X. P. Yan, ACS Appl. Nano Mater., 2019, 2, 7290–7298 CrossRef CAS.
- G. Xiong, B. B. Wang, L. X. You, B. Y. Ren, Y. K. He, F. Ding, I. Dragutan, V. Dragutan and Y. G. Sun, J. Mater. Chem. A, 2019, 7, 393–404 RSC.
- Y. Gan, G. Chen, Y. Sang, F. Zhou, R. Man and J. Huang, Chem. Eng. J., 2019, 368, 29–36 CrossRef CAS.
- K. Y. Yan, J. Y. Chen, X. Y. Li, Q. Wang and G. C. Kuang, Macromol. Chem. Phys., 2020, 221, 1900553 CrossRef CAS.
- R. Shen and H. Liu, RSC Adv., 2016, 6, 37731–37739 RSC.
- T. Xu, Y. He, Y. Qin, C. Zhao, C. Peng, J. Hu and H. Liu, RSC Adv., 2018, 8, 4963–4968 RSC.
- J. He, N. Wang, Z. Cui, H. Du, L. Fu, C. Huang, Z. Yang, X. Shen, Y. Yi, Z. Tu and Y. Li, Nat. Commun., 2017, 8, 1172 CrossRef PubMed.
- J. Fu, Z. Chen, M. Wang, S. Liu, J. Zhang, J. Zhang, R. Han and Q. Xu, Chem. Eng. J., 2015, 259, 53–61 CrossRef CAS.
- T. Wang, K. Kailasam, P. Xiao, G. Chen, L. Chen, L. Wang, J. Li and J. Zhu, Microporous Mesoporous Mater., 2014, 187, 63–70 CrossRef CAS.
- X.-H. Zhang, X. P. Wang, J. Xiao, S. Y. Wang, D. K. Huang, X. Ding, Y.-G. Xiang and H. Chen, J. Catal., 2017, 350, 64–71 CrossRef CAS.
- H. Zhou, B. Zhao, C. Fu, Z. Wu, C. Wang, Y. Ding, B.-H. Han and A. Hu, Macromolecules, 2019, 52, 3935–3941 CrossRef CAS.
- Y. Wang, H. Liu, Q. Pan, C. Wu, W. Hao, J. Xu, R. Chen, J. Liu, Z. Li and Y. Zhao, J. Am. Chem. Soc., 2020, 142, 5958–5963 CrossRef CAS PubMed.
- T. Xu, Y. Li, Z. Zhao, G. Xing and L. Chen, Macromolecules, 2019, 52, 9786–9791 CrossRef CAS.
- Q. Huang, L. Guo, N. Wang, X. Zhu, S. Jin and B. Tan, ACS Appl. Mater. Interfaces, 2019, 11, 15861–15868 CrossRef CAS PubMed.
- Y. Liu, C. y. Liu, L. b. Chen and Z. y. Zhang, J. Colloid Interface Sci., 2003, 257, 188–194 CrossRef CAS PubMed.
- S. Jang, J. Park, S. Shin, C. Yoon, B. K. Choi, M.-s. Gong and S.-W. Joo, Langmuir, 2004, 20, 1922–1927 CrossRef CAS.
- J. H. Huang, K. L. Huang, S. Q. Liu, A. T. Wang and C. Yan, Colloids Surf., A, 2008, 330, 55–61 CrossRef CAS.
- M. Barylak, K. Cendrowski and E. Mijowska, Ind. Eng. Chem. Res., 2018, 57, 4867–4879 CrossRef CAS.
- Q. Li, Z. Zhan, S. Jin and B. Tan, Chem. Eng. J., 2017, 326, 109–116 CrossRef CAS.
- Y. Han, W. Li, J. Zhang, H. Meng, Y. Xu and X. Zhang, RSC Adv., 2015, 5, 104915–104922 RSC.
- X. Shen, S. Ma, H. Xia, Z. Shi, Y. Mu and X. Liu, J. Mater. Chem. A, 2018, 6, 20653–20658 RSC.
- H. J. Zhang, J. H. Wang, Y. H. Zhang and T. L. Hu, J. Polym. Sci., Part A: Polym. Chem., 2017, 55, 1329–1337 CrossRef CAS.
- M. G. Mohamed, A. F. M. El-Mahdy, T. S. Meng, M. M. Samy and S. W. Kuo, Polymers, 2020, 12, 2426 CrossRef CAS PubMed.
- Q. Jiang, H. Huang, Y. Tang, Y. Zhang and C. Zhong, Ind. Eng. Chem. Res., 2018, 57, 15114–15121 CrossRef CAS.
- D. Jiang, R. Deng, G. Li, G. Zheng and H. Guo, RSC Adv., 2020, 10, 6185–6191 RSC.
- S. Wang, L. Shao, Y. Sang and J. Huang, J. Chem. Eng. Data, 2019, 64, 1662–1670 CrossRef CAS.
- Y. Chen, Y. Fang, J. Yu, W. Gao, H. Zhao and X. Zhang, J. Hazard. Mater., 2021, 406, 124769 CrossRef CAS PubMed.
- T. Xu, S. An, C. Peng, J. Hu and H. Liu, Ind. Eng. Chem. Res., 2020, 59, 8315–8322 CrossRef CAS.
Footnote |
† Electronic supplementary information (ESI) available. See DOI: 10.1039/d1ra01382c |
|
This journal is © The Royal Society of Chemistry 2021 |
Click here to see how this site uses Cookies. View our privacy policy here.