DOI:
10.1039/D1RA01548F
(Paper)
RSC Adv., 2021,
11, 16490-16499
Solvothermal synthesis of poly(acrylic acid) decorated magnetic molybdenum disulfide nanosheets for highly-efficient adsorption of cationic dyes from aqueous solutions†
Received
26th February 2021
, Accepted 28th April 2021
First published on 5th May 2021
Abstract
Herein we report solvothermal synthesis of poly(acrylic acid) (PAA) decorated magnetic molybdenum disulfide nanosheets (MMoS2/PAA) for highly efficient adsorption of three cationic dyes of basic fuchsin (BF), methylene blue (MB), and crystal violet (CV) from aqueous solutions. The synthesized MMoS2/PAA was characterized by several techniques including transmission electron microscopy (TEM), Fourier transform-infrared spectroscopy (FT-IR), X-ray photoelectron spectroscopy (XPS), thermogravimetric analysis (TGA), vibrating sample magnetometry (VSM), and dynamic light scattering (DLS). Due to the strong electrostatic interaction between cationic dyes and the anionic nanosheet surface, the obtained MMoS2/PAA showed ultrafast adsorption of BF, MB and CV within 2 min with high adsorption capacities of 886.1, 709.0, and 633.6 mg g−1, respectively, much higher than those materials reported recently. PAA molecules bound on the nanosheets played a crucial role in significantly enhancing the dye adsorption. The adsorption kinetics and isotherms of three dyes onto the MMoS2/PAA were well described by the pseudo-second-order kinetic and the Langmuir models. Moreover, the MMoS2/PAA also exhibited high removal efficiencies for various mixed-dye solutions. Besides, such a functional nanomaterial could be effectively recovered from dye solutions under an external magnetic field and reused for dye adsorption without compromising on its performance indicating it can serve as an excellent adsorbent for effective removal of a variety of cationic organic pollutants from industrial effluents.
1. Introduction
Organic dyes are widely used in printing, textiles, paint, pigment and many other significant industries. Dye effluents discharged from numerous dye-utilizing industries have posed a serious threat to human beings and marine environments.1,2 The presence of dyes in water reduces the light penetration and lowers oxygen gas solubility, thus decreasing the photosynthetic efficiency of aquatic plants. Moreover, most dyes are highly toxic and can have teratogenic, carcinogenic and mutagenic effects on aquatic life and humans even at a very low concentration.3,4 Therefore, effective removal of dye contaminants prior to discharge into the water environment is of great importance. Currently various biological, chemical and/or physical technologies such as biodegradation, chemical oxidation, ion exchange, membrane filtration and adsorption have been used for eliminating dye pollutants from environmental wastewater.1–6 Among them, adsorption is regarded as the most effective technique to remove dye contaminants from water since its inexpensiveness, high efficiency, wide adaptability and convenient operation.3–7 The key of this method is developing high-performance adsorbents.
Two-dimensional (2D) laminar materials have recently attracted considerable attentions as promising adsorbents for pollutant treatment due to their unique properties of large specific surface area, high chemical stability, and low production cost.8,9 Molybdenum disulfide (MoS2) nanosheets and their composites, as newly emerging 2D layered materials have been intensively studied for eliminating varieties of organic and inorganic pollutants from water.10–14 However, recovering those nanomaterials from aqueous solutions is time-consuming and expensive owing to involvement of complex filtration or/and centrifugation operations, thus hampering their practical applications. Loading certain amount of superparamagnetic Fe3O4 nanoparticles (NPs) on the surface endows them convenient separability from contaminated water under an external magnetic field (EMF) seems feasible to solve this problem. However, introducing Fe3O4 NPs inevitably reduces active adsorption sites on their surface, thus lowering its equilibrium adsorption capacity for pollutants.15,16 Through surface decoration using polymers with rich active groups will dramatically enhance their uptakes for organic dyes.
In this work, poly(acrylic acid) (PAA) with plentiful of anionic carboxylic groups (–COO−) that have high affinity to cationic dyes, was utilized to decorate Fe3O4 NPs-loaded MoS2 nanosheets for synthesizing MMoS2/PAA nanocomposite. The synthesized MMoS2/PAA showed high adsorption capacities for three typical cationic dyes of basic fuchsin (BF), methylene blue (MB), and crystal violet (CV). As shown in Fig. 1a, MoS2 nanosheets with relative large specific surface area were first synthesized by versatile hydrothermal method.17 Then superparamagnetic Fe3O4 NPs and poly(acrylic acid) (PAA) functional polymers were simultaneously introduced on the MoS2 surface by simple solvothermal reaction, thus causing the formation of MMoS2/PAA. The resulted MMoS2/PAA was characterized by several techniques including transmission electron microscope (TEM), Fourier transform-infrared spectroscopy (FT-IR), X-ray photoelectron spectroscopy (XPS), thermogravimetric analysis (TGA), vibrating sample magnetometry (VSM), and dynamic light scattering (DLS) instrument. Thanks to strong electrostatic interaction between cationic dyes and the anionic –COO− groups in PAA molecules, the MMoS2/PAA showed ultrafast adsorption of BF, MB and CV within 2 min with high adsorption capacities of 886.1, 709.0, and 633.6 mg g−1, respectively, comparable and even higher than those materials reported previously (Table 1).4,10,11,15,18–23 PAA molecules on the MoS2 played a crucial role in significantly enhancing the adsorption of cationic dyes. The adsorption behaviors were fitted well with the pseudo-second-order kinetic and the Langmuir models. The batch adsorption experiments with the effects of parameters like solution pH, contact time, initial dye concentrations and temperature on the adsorption were optimized for dye adsorption. Moreover, the MMoS2/PAA also demonstrated high removal efficiency for various mixed dye solutions and excellent regenerability. Such a functional nanocomposite with ultrafast adsorption rate and high adsorption capacities for cationic dyes is promising to become an excellent adsorbent for removing various cationic organic pollutants from industrial effluents.
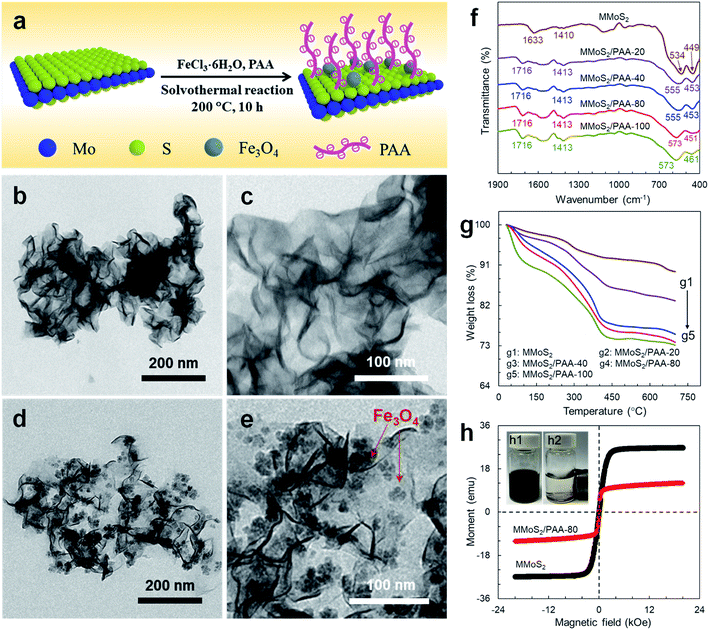 |
| Fig. 1 (a) Schematic illustration of the synthesis of MMoS2/PAA; typical TEM images of (b and c) MoS2 and (d and e) MMoS2/PAA-80; FT-IR spectra (f) and TGA curves (g) of MMoS2, MMoS2/PAA-20, MMoS2/PAA-40, MMoS2/PAA-80, and MMoS2/PAA-100; (h) magnetic hysteresis curves of MoS2 and MMoS2/PAA-80. The inset showed the digital photographs of MMoS2/PAA-80 aqueous dispersion before (h1) and after (h2) magnetic separation. | |
Table 1 Comparison of dye adsorption capacity by various adsorbents
Adsorbent |
Dye |
qe (mg g−1) |
Ref. |
Fe3O4/chitosan/graphene |
MB |
81.6 |
4 |
MoS2/graphene on cellulose paper |
MB |
485.4 |
10 |
Ultrathin MoS2 nanosheets |
MB |
146.4 |
11 |
Fe3O4 decorated MoS2 nanosheets |
Congo |
71.0 |
15 |
NH2-modified titanate nanotubes |
Remazol blue R |
435.6 |
18 |
PSSMA-modified Fe3O4 NPs |
MB |
52.2 |
19 |
PAA-functionalized magnetic GO |
MB |
291.0 |
20 |
M3D–PAA–CCN |
MB |
332.0 |
21 |
Fe3O4/activated carbon |
Rhodamine B |
182.5 |
22 |
Methyl orange |
150.4 |
22 |
Chitosan/heulandite/Fe3O4 |
MB |
45.1 |
23 |
Methyl orange |
149.2 |
23 |
MMoS2/PAA |
BF |
886.1 |
This work |
MB |
709.0 |
CV |
633.6 |
2. Experimental section
2.1 Chemical materials
Sodium molybdate dihydrate (Na2Mo4·2H2O, ≥99.0%) and thioacetamide (C2H5NS, ≥99.0%) were purchased from Aladdin Chemicals (Shanghai, China). Poly(acrylic acid) (PAA, MW = 1800, ≥99.0%) was obtained from Sigma-Aldrich Chemicals (Shanghai, China). Iron(III) chloride hexahydrate (FeCl3·6H2O, ≥99%), sodium acetate anhydrous (CH3COONa, ≥99.0%), basic fuchsin (BF, ≥99.0%), methylene blue (MB, ≥99.0%), and crystal violet (CV, ≥99.0%) were bought from Kelong Chemicals (Chengdu, China). All other chemicals were of analytical grade (≥99.5%), purchased from Chengdu Kelong Chemicals and used as received. Deionized water (18.2 MΩ, 25 °C) from a Milli-Q plus purification system (Millipore) was used throughout the experiments.
2.2 Synthesis of MoS2 nanosheets
MoS2 nanosheets were synthesized by a versatile hydrothermal reaction.17 Briefly, 0.3 g of Na2Mo4·2H2O and 0.6 g of C2H5NS were dissolved in 40 mL water by sonication for 30 min. Then the obtained solution was transferred into a 100 mL Teflon-lined stainless-steel autoclave and reacted at 220 °C for 24 h. After natural cooling, the black precipitate was separated by centrifugation at 8500 rpm for 15 min and then washed with water several times, and finally dispersed in 20 mL water for subsequent use.
2.3 Synthesis of MMoS2/PAA
PAA-bound magnetic MoS2 nanosheets (MMoS2/PAA) were synthesized according to the ref. 17 with some modification. In brief, 15 mL of MoS2 aqueous dispersion (∼100 mg MoS2) was mixed with 30 mL water/ethanol (v/v = 1
:
2) for 1 h by ultrasonication. Then 0.5 g of FeCl3·6H2O, 0.75 g of CH3COONa and some amount of PAA (0, 20, 40, 80, and 100 mg) were added under stirring for 30 min. After that, the mixture was transferred into 100 mL Telfon-lined stainless-steel autoclave and heated at 200 °C for 10 h. After natural cooling, the black precipitates were collected with a magnet and washed with water several times, and then dispersed in 20 mL water (denoted as MMoS2, MMoS2/PAA-20, MMoS2/PAA-40, MMoS2/PAA-80, and MMoS2/PAA-100, respectively) for the subsequent adsorption experiments. To conduct the contrast trials, PAA-modified magnetic NPs sample (Fe3O4/PAA-100) was synthesized with the same method using 100 mg PAA while without addition of MoS2 during the solvothermal reaction.
2.4 Characterization
Transmission Electron Microscope (TEM) images were obtained with a JEM-2010 microscope (JEOL, Japan) at an accelerating voltage of 120 kV. The samples were prepared by drop-casting a dilute solution of particles onto carbon-coated copper grids, followed by air drying. FT-IR spectra were recorded on an IR 200 spectrometer (Thermo Nicolet, USA) using KBr pellets. X-Ray photoelectron spectroscopy (XPS) was performed on a Thermo Fisher ESCALAB Xi+. Thermogravimetric analysis (TGA) was performed on a Mettler TGA/SDTA851e° instrument (Switzerland) at a heating rate of 5 °C min−1 from 30 to 700 °C under N2 atmosphere. Magnetic property was measured by vibrating sample magnetometry (VSM) on a model 2000 physical property measurement system (Quantum Design) at room temperature. Zeta potentials of samples were determined on a dynamic light scattering (DLS) instrument (Zetasizer Nano-ZS, Malvern Instruments, UK).
2.5 Batch adsorption experiments
Typically, 10 mg of MoS2 nanocomposites was added in 30 mL aqueous solutions contained BF, MB, and CV with desired concentrations and pH values in glass vials. The initial pH values of solutions were regulated by adding negligible volume of 0.1 M NaOH and/or HCl solution. Then the solid–liquid mixtures were shaken in a thermostatic shaker (80 rpm) at a desired temperature for certain time. To confirm that PAA bound on the MMoS2 played a significant role in adsorption of three cationic dyes, the MMoS2/PAA serials samples synthesized with different dosages of PAA during the solvothermal process (MMoS2, MMoS2/PAA-20, MMoS2/PAA-40, MMoS2/PAA-80, and MMoS2/PAA-100) were used for dye adsorption. To study the effect of initial dye concentration on the adsorption and adsorption isotherms, the concentrations were varied from 50 to 600 mg L−1 for MB and CV, and from 50 to 700 mg L−1 for BF. To probe the influence of contact time on the adsorption and adsorption dynamics, the contact time between adsorbents and dyes changed from 0 to 20 min. The dye-saturated materials were collected from solutions with a magnet, and the dye concentrations before and after adsorption were measured on a UV-vis spectrophotometer (TU-1950, Persee, Beijing, China) at the absorbance of 540, 664 and 590 nm for BF MB, and CV, respectively. The used adsorbents were regenerated by washing thrice with NaOH/ethanol solution (0.1 M) and reused for dye adsorption. The adsorption capacity, qe (mg g−1) and removal efficiency (%) of dyes onto adsorbents were calculated from the following equations: |
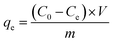 | (1) |
|
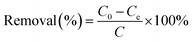 | (2) |
where C0 and Ce (mg L−1) are the initial and equilibrium concentrations of dyes in solutions, respectively. V (L) is the volume of dye solutions, and m (g) is the mass of adsorbents. All the adsorption experiments were performed at least thrice.
3. Results and discussion
3.1 Materials characterizations
The morphologies and microstructures of materials were observed by TEM. As observed in Fig. 1b and c, MoS2 has a 2D wrinkled and layered microstructure. After solvothermal reaction, multiple black Fe3O4 NPs with size range of 20–50 nm were loaded on the MoS2 surface (Fig. 1d and e), endowing convenient separability of MoS2 nanosheets from contaminated aqueous solutions under an EMF. Loh and coworkers reported one kind of edge-oriented MoS2 nanosheet-like films that exhibited weak magnetism (∼1–2 emu g−1) and 2.5% magnetoresistance effects with a Curie temperature of 685 K.24 The metal oxides or sulfides film with a high density of edge spins could give rise to interesting magnetic phenomena even though the bulk material is nonmagnetic. The MoS2 thin films prepared by thermal evaporation of the single-source precursor tetrakis(diethyl-aminodithiocarbomate)molybdate(IV) owned the rhombohedral or hexagonal edges. In this work the MoS2 nanosheets were synthesized by hydrothermal reaction, and demonstrated significantly different 2D wrinkled and layered microstructure, which is consistent with those previous reports.17,25 The successful binding of PAA on the magnetic MoS2 (MMoS2) surface was confirmed by FT-IR spectrum. Fig. 1f shows the FT-IR spectra of MMoS2 before and after binding of PAA. MMoS2 is composed of MoS2 and Fe3O4. As observed in Fig. 1f, the strong band at around 1633 cm−1 is attributed to the bending vibration of adsorbed water molecules on the MoS2 surface,26 the adsorption band at 1410 cm−1 is related to the C–N groups from the C2H5NS used in the synthesis,27 and the characteristic peak at 449 cm−1 is attributable to the Mo–S bond from the MoS2.26 Besides, the peak at 534 cm−1 is due to the Fe–O stretching vibration from Fe3O4 NPs,19,28 indicating that Fe3O4 NPs have been introduced on the MoS2 to cause the formation of MMoS2. After binding of PAA on the MMoS2, the typical peak of C
O stretching vibration of PAA at 1716 cm−1 is evidently observed in all the spectra of MMoS2/PAA samples.20 Besides, the peak of Fe–O is observed to shift from 534 to 555 cm−1 for both MMoS2/PAA-20 and MMoS2/PAA-40 with low binding amount of PAA, and shift to 573 cm−1 for MMoS2/PAA-80 and MMoS2/PAA-100 with higher binding amount due to the coordination interaction between iron cations and multiple –COO− groups.29 These results indicate that PAA functional molecules and Fe3O4 NPs have successfully introduced on the MoS2 surface, causing the formation of MMoS2/PAA.
The binding amount of PAA on the MMoS2 surface was estimated by TGA technique. Fig. 1g shows the TGA curves of MMoS2, MMoS2/PAA-20, MMoS2/PAA-40, MMoS2/PAA-80, and MMoS2/PAA-100. As observed, all the samples exhibited a two-step loss weight process as the temperature increased from 30 to 700 °C. The first weight loss at temperature below ∼120 °C was caused by the evaporation of physically-adsorbed water on the materials. The second weight loss at temperature range of 120–430 °C was ascribed to the decomposition of PAA bound on the MoS2 surface,20 and the binding amount increased with an increase in PAA dosage during the solvothermal reaction, in agreement with the FT-IR results. The binding amounts were calculated to be respectively 72.9, 156.4, 176.5, and 183.3 mg g−1 for MMoS2/PAA-20, MMoS2/PAA-40, MMoS2/PAA-80, and MMoS2/PAA-100 based on the TGA results. Compared with MMoS2/PAA-80, no evident increase of binding amount was observed for MMoS2/PAA-100. Therefore, MMoS2/PAA-80 was used for the subsequent characterization.
To further characterize the chemical compositions of the MoS2 before and after modification, the resulted MoS2 and MMoS2/PAA-80 samples were examined by XPS. The high-resolution XPS spectra of Mo 3d and S 2p in MoS2 and MMoS2/PAA-80 were shown in Fig. S1.† The distinct doublet peaks of Mo 3d spectra at 228.6 (Mo 3d5/2) and 231.7 eV (Mo 3d3/2) were attributed to the Mo4+ oxidation state of MoS2 nanosheets11,30 (Fig. S1a†). The relatively weak peak located at 235.95 eV were assigned to Mo 3d peaks with a higher oxidation state (Mo6+), which may originate from the partial oxidation of Mo atoms at the edges or defects on the crystal plane of the MoS2 nanosheets. The peak located at 225.85 eV was arising from S 2s in MoS2, and the two peaks at 162.5 and 161.45 eV were attributed to S 2p3/2 and S 2p1/2 (ref. 11 and 30) (Fig. S1c†). After PAA decoration, the corresponding peaks slightly shift towards the higher binding energy (Fig. S1b and d†). Excellent magnetic property of nanocomposites endows them convenient separability from polluted aqueous solutions under an EMF.
The magnetism of MMoS2 before and after PAA modification were studied by VSM. As observed in Fig. 1h, the MMoS2 sample had high saturation magnetization (Ms) value of 26.8 emu g−1. After introduction of non-magnetic PAA on its surface, the Ms value lowered to 9.2 emu g−1. But such a Ms value was high enough to enable it excellent separability in a few minutes from water under an EMF (Fig. 1h, the right inset). As the EMF was removed, being slightly shaken, the particles could be well redispersed in water, indicating excellent superparamagnetism of the nanocomposite, highly beneficial to its practical application such as dye adsorption.
3.2 Adsorption performance
To confirm that PAA bound on the MMoS2 played a crucial role in improving the adsorption of cationic dyes, the MMoS2/PAA serials nanocomposites with various binding amounts of PAA (0, 72.9, 156.4, 176.5, and 183.3 mg g−1 corresponding to the MMoS2, MMoS2/PAA-20, MMoS2/PAA-40, MMoS2/PAA-80, and MMoS2/PAA-100) were utilized to remove three typical dyes of BF, MB and CV from aqueous solutions. Furthermore, in order to prove that MoS2 nanosheets played an important role in enhancing the dye adsorption since which provided large specific surface area for PAA binding and loading of magnetic Fe3O4 NPs, we carried out an adsorption experiment by using the PAA-bound Fe3O4 NPs without MoS2 components (Fe3O4/PAA-100) in the nanocomposite. As observed in Fig. 2a, the Fe3O4/PAA-100 showed very low adsorption capacities for three cationic dyes. The maximum adsorption capacities of BF, MB, and CV were only 31.0, 26.9, and 19.8 mg g−1, respectively (Fig. 2a). However, when Fe3O4 NPs were loaded on the MoS2 surface to produce MMoS2, which showed higher adsorption capacities for BF, MB, and CV (156.78, 73.15, and 55.33 mg g−1, respectively) than Fe3O4/PAA-100 and the PSSMA-modified Fe3O4 NPs (52.22 mg g−1 for MB) developed in our previous work,19 indicating that the presence of MoS2 with larger specific surface area in the nanocomposite played a crucial role in improving the dye adsorption. Electrostatic interaction between cationic dyes and few numbers of residual anionic groups (COO−) on the material contributed small adsorption,20,28 which were generated from incomplete reduction of –COOH groups on the surface during the solvothermal process.20 In comparison to MMoS2, the PAA-bound MMoS2/PAA serials exhibited much higher dye uptakes since a large number of active sites (COO−) in PAA available for capturing cationic dye molecules.20,28 Due to presence of numerous PAA functional molecules on the MMoS2, the MMoS2/PAA-80 behaved long-term water dispersibility (Fig. S2a†). By comparison, owing to absence of PAA on the surface, MMoS2 particles was easily precipitated out of water within 5 min (Fig. S2b†). Moreover, the uptake of three dyes onto the MMoS2/PAA increased with increasing PAA dosage during the solvothermal process, and kept nearly invariable at dosage over 80 mg. A larger dosage gave rise to a higher binding amount, thus providing more active sites (COO− groups) for dye adsorption via electrostatic interaction, finally leading to higher dye uptakes. Maximum uptakes of BF, MB, and CV onto MMoS2/PAA-80 were respectively 588.0, 574.6, and 535.6 mg g−1 at an initial concentration of 200 mg L−1, much higher than those on the MMoS2, indicating that PAA polymers on the MMoS2 could significantly improve the uptakes of cationic dyes.
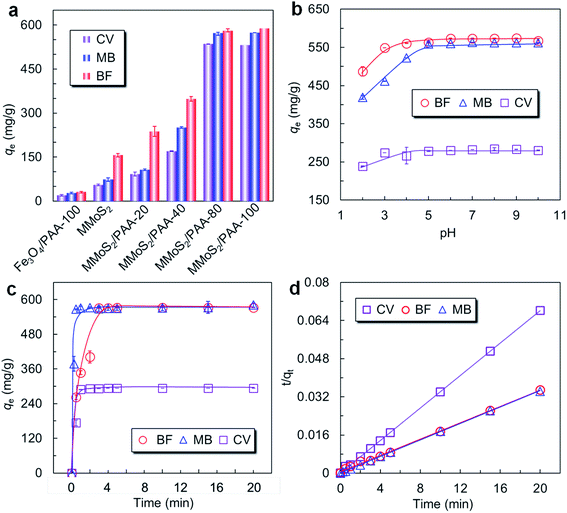 |
| Fig. 2 (a) Equilibrium adsorption capacities of BF, MB, and CV onto Fe3O4/PAA-100 and the MMoS2/PAA serials adsorbents; effects of initial solution pH values (b) and contact time (c) on the adsorption of BF, MB, and CV onto MMoS2/PAA-80; (d) fitting curves of pseudo-second-order kinetic model. Condition: m/V = 10 mg/30 mL, T = 25 °C. CBF = CMB = CCV = 200 mg L−1 in (a), and CCV = 100 mg L−1, CBF = CMB = 200 mg L−1 in (b–d). | |
3.2.1 Effect of pH. Solution pH significantly affects the surface charges of an adsorbent and the degree of ionization of active sites.10,22 Therefore, effect of solution pH on the adsorption of BF, MB and CV onto MMoS2/PAA-80 was studied in this work. As shown in Fig. 2b, the adsorption capacities of MMoS2/PAA-80 for three dyes were pH-dependent. With increasing solution pH values from 2.0 to 5.0, the uptakes of three dyes increased gradually, and then reached equilibrium at solution pH values above 5.0. Changing solution pH over the investigated range of 2.0–10.0 dramatically affected the surface charges of the synthesized MMoS2/PAA-80 (Fig. S3†), thus the electrostatic interaction between the cationic BF, MB and CV molecules and the negatively charged adsorbent surface, finally their dye uptakes. As the solution pH was lower than 5.0 (nearby the pKa of PAA, 4.75), protonation of –COOH groups in PAA molecules weakened the electrostatic interaction between dye molecules and PAA, thus lowering the dye uptakes. While at higher pH media (≥5.0), deprotonation of –COOH increased the surface charges of the MMoS2/PAA-80 (Fig. S3†), thus enhancing the electrostatic interaction between dye molecules and PAA, as a result, promoting dye adsorption.20,31 Moreover, MMoS2/PAA-80 showed higher uptakes for BF and MB than that for CV due to different initial concentrations of dyes. The initial concentrations of BF, MB and CV were 200, 200 and 100 mg L−1, respectively. A higher initial concentration facilitated a stronger driving force to overcome the mass transfer resistance of dye molecules between the aqueous phase and the solid phase, thus causing more collisions between dyes and active sites.32 Since the dye adsorptions were little unchanged at higher pH, the solution pH was fixed at 7.0 in following adsorption experiments.
3.2.2 Effect of contact time and adsorption kinetics. The time-dependent dye adsorption onto MMoS2/PAA-80 was studied at initial concentrations of BF, MB and CV of 200, 200 and 100 mg L−1, respectively. As observed in Fig. 2c, the adsorption capacities of three dyes onto MMoS2/PAA-80 sharply increased within the first 2 min, and then reached equilibrium. At the first adsorption stage, all the vacant active sites on the MMoS2/PAA-80 were conveniently available for binding dye molecules. As the adsorption proceeded the available number of active sites reduced, and repulsion occurred between the adsorbent and dye molecules. Finally, the equilibrium reached as the adsorption/desorption rates were identical, and increased in adsorption capacities were no longer observed.10 MMoS2/PAA-80 showed high adsorption capacities for BF, MB and CV (572.1, 581.6, and 293.2 mg g−1, respectively) due to high binding amounts of PAA on the surface, thus providing plentiful of active sites. This indicates that the synthesized MMoS2/PAA in this work can serve as an excellent adsorbent for effective removal of cationic dyes from contaminated water. To well understand the adsorption process, two classical kinetic models of pseudo-first-order (eqn (3)) and pseudo-second-order (eqn (4)) kinetic models were used to analyze the adsorption data.Pseudo-first-order model:33
|
ln(qe − qt) = ln qe − k1t
| (3) |
Pseudo-second-order model:34
|
 | (4) |
where
qe and
qt (mg g
−1) refer to the adsorption capacities of dyes at equilibrium state and at time
t (min), respectively;
k1 (min
−1) and
k2 (g (mg
−1 min
−1)) represent the rate constants of the pseudo-first-order and pseudo-second-order kinetic models, respectively. The values of
qe and
k1 for pseudo-first-order model could be determined experimentally by plotting ln(
qe −
qt)
versus t and extracting information from the least squares analysis of slope and intercept and substituting into
eqn (3), while the values of
qe and
k2 for pseudo-second-order model could be calculated from the slope and intercept of the linear relationship between (
t/
qt) and
t (
eqn (4)).
The corresponding kinetic parameters and correlation coefficients (R2) were calculated by the linear regression of the two kinetic models and listed in Table 2. Higher R2 values (>0.998) were obtained for the pseudo-second-order model, and all of the calculated adsorption capacities (qe,cal) for three dyes were closer to the experimental values (qe,exp), indicating that the kinetics of the dyes adsorption onto MMoS2/PAA-80 fitted better the pseudo-second-order model (Fig. 2d and S4†), and the interaction between dye molecules and the MMoS2/PAA-80 was mainly controlled by chemisorption.
Table 2 Kinetic parameters of the adsorption of BF, MB, and CV onto MMoS2/PAA-80
Dye |
C0 (mg L−1) |
qe,exp (mg g−1) |
Pseudo-first-order |
Pseudo-second-order |
qe,cal (mg g−1) |
R2 |
k1 (min−1) |
qe,cal (mg g−1) |
R2 |
k2 (g (mg−1 min−1)) |
BF |
200 |
572.1 |
112.5 |
0.6840 |
0.6422 |
588.2 |
0.9980 |
0.0048 |
MB |
200 |
581.6 |
24.5 |
0.1718 |
0.0826 |
588.2 |
0.9999 |
0.0029 |
CV |
100 |
293.2 |
5.1 |
0.3508 |
0.3129 |
294.1 |
0.9998 |
0.0578 |
3.2.3 Effect of initial dye concentrations and adsorption isotherms. To further study the behaviors of equilibrium adsorption, effect of initial dye concentrations on the adsorption of BF, MB and CV onto MMoS2/PAA-80 were also investigated in this work. As shown in Fig. 3a, the adsorption capacities of three dyes gradually increased with increasing the dye concentrations at first, and then reached adsorption saturation at higher concentrations. For a given adsorbent, the total number of active sites (–COO−) available for binding dye molecules was fixed. At low dye concentrations (below 400 mg L−1), the available active sites on the adsorbent were excessive and could fully interact with relatively insufficient dye molecules in solution via strong electrostatic interaction. While at higher concentrations (≥400 mg L−1), dyes became surplus, and those excessive dye molecules were never captured by –COO− groups, thus equilibrium adsorptions were achieved.35 Moreover, MMoS2/PAA-80 showed different adsorption capacities for three dyes over the studied concentration range due to the different molecular structures of dyes. The higher uptake of BF was maybe due to the hydrogen-bond interaction between the primary amino group (–NH2) in BF molecules and the –COOH groups in PAA, facilitating additional dye adsorption. As for MB and CV, a relative larger molecular structure of CV generated higher steric hindrance than MB (Fig. S5†), accounted for a lower dye adsorption.
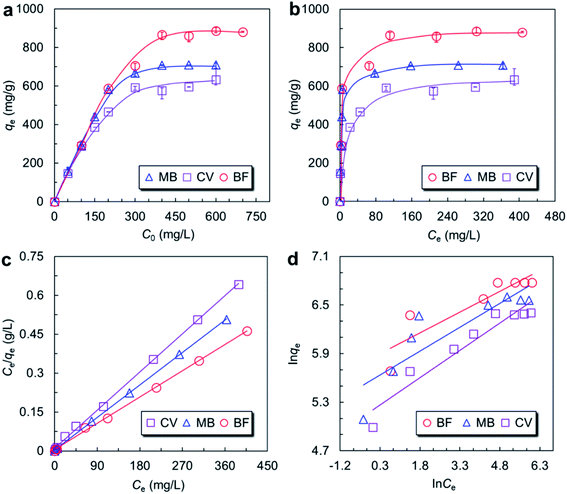 |
| Fig. 3 (a) Effects of initial dye concentrations on the adsorption of BF, MB, and CV onto MMoS2/PAA-80; The non-linear regression (b), linear Langmuir (c) and Freundlich (d) isotherms. Condition: pH = 7.0, m/V = 10 mg/30 mL, T = 25 °C, and contact time = 5 min. | |
To further ascertain the adsorption mechanism, adsorption isotherms of BF, MB and CV onto MMoS2/PAA-80 were investigated in this work. The adsorption data were fitted by the well-known Langmuir and Freundlich isotherm models.36,37
Langmuir isotherm model assumes monolayer adsorption of dye molecules over homogenous adsorbent surface, and all of the active sites being equivalent, which was expressed as follows:36
|
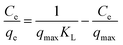 | (5) |
here,
Ce (mg L
−1) is the equilibrium concentration in dye solutions,
qe and
qmax (mg g
−1) are the equilibrium and maximum adsorption capacities of dyes, respectively,
KL (L mg
−1) represents the Langmuir constant related to the energy of adsorption. The values of
qmax and
KL could be calculated from the slopes and intercepts of the linear plots of
Ce/
qe versus Ce (
eqn (5)).
One of the essential features of Langmuir isotherm could be described by a separation factor, RL, which was defined as follows:4
|
 | (6) |
From the theory of Langmuir isotherm, the value RL indicates the shape of Langmuir isotherm to be either unfavorable (RL > 1), linear (RL = 1), favorable (1 > RL > 0) or irreversible (RL = 0). In this study all the RL values lay in between 0 and 1, indicating that the adsorption of three dyes onto MMoS2/PAA-80 were favorable processes.
Freundlich isotherm model takes the assumption that adsorption occurs on heterogeneous surface without saturation of active sites. The equation was expressed as follows:37
|
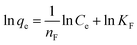 | (7) |
where
qe is the equilibrium adsorption capacities of dyes,
Ce (mg L
−1) is the equilibrium concentration in dye solutions,
KF ((mg
1−1/n L
1/n)/g) and 1/
nF represent an empirical parameters related to the intensity of the adsorption interaction.
KF and 1/
nF could be achieved from the slopes and intercepts of the linear plots of ln
qe versus ln
Ce (
eqn (7)).
The Langmuir and Freundlich isotherms obtained by curve fitting were shown in Fig. 3c and d. The model parameters were listed in Table 3. The R2 values for all the dyes obtained from the Langmuir model higher (≥0.998) indicated that the Langmuir isotherm model matched better with the experimental data (Fig. 3c). The qmax,cal values of BF, MB, and CV were 909.1, 714.3, and 625.0 mg g−1, respectively, in accordance with the qmax,exp values well. Therefore, the adsorption of three dyes was monolayer adsorption and the surface of adsorbents was homogeneous.4,32 Besides, the maximum adsorption capacities of dyes were higher than those materials reported previously (Table 1),4,10,11,15,18–23 indicating that the synthesized MMoS2/PAA in this work could serve as an excellent adsorbent for effective removal of cationic dye wastewater.
Table 3 Isotherm parameters of the adsorption of MB, CV and BF onto MMoS2/PAA-80
Dye |
qexp,cal (mg g−1) |
Langmuir |
Freundlich |
qmax,cal (mg g−1) |
R2 |
KL (L mg−1) |
RL |
nF |
KF ((mg1−1/n L1/n)/g) |
R2 |
MB |
709.0 |
714.3 |
0.9998 |
0.3256 |
0.0151 |
4.792 |
246.8 |
0.7374 |
CV |
633.6 |
625.0 |
0.9989 |
0.1684 |
0.0561 |
4.789 |
196.0 |
0.8845 |
BF |
886.1 |
909.1 |
0.9992 |
0.2444 |
0.0201 |
5.824 |
346.9 |
0.8058 |
3.2.4 Effect of temperature and thermodynamics of adsorption. Temperature is another significant factor to be considered during the adsorption of dyes since it affects the adsorption rate which in turn affects the equilibrium adsorption capacity. Fig. S6† showed the effect of temperature on the adsorption of BF, MB and CV onto MMoS2/PAA-80 at initial concentrations of 200, 200 and 100 mg L−1, respectively. The adsorption capacities decreased with increased temperatures. The thermodynamic parameters reflecting internal energy changes for the adsorption process, including free energy change (ΔG), enthalpy change (ΔH), and entropy change (ΔS), were calculated by the following equations:22 |
ΔG = −RT ln KL
| (8) |
|
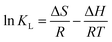 | (9) |
where R is the universal gas constant (8.314 J (mol−1 K−1)), T is the absolute temperature (K), and KL is the Langmuir constant. The values of ΔH and ΔS were obtained from the slope and intercept of the van't Hoff plot of ln
KL versus 1/T.The ΔG, ΔH, and ΔS values of three dyes at various temperatures were listed in Table 4. The positive values of ΔS indicated the affinity of MMoS2/PAA-80 for three dyes and increasing randomness at the solid–liquid interface in the adsorption reaction. The negative values of ΔH showed that the adsorption processes were exothermic. The negative values of ΔG increased with an increase in temperature, indicating that the adsorption was spontaneous and more favorable at lower temperature.32
Table 4 Thermodynamic parameters for MB, CV and BF adsorption by MMoS2/PAA-80
T (K) |
ΔG (kJ mol−1) |
ΔH (kJ mol−1) |
ΔS (J (mol−1˙K)) |
MB |
CV |
BF |
MB |
CV |
BF |
MB |
CV |
BF |
298 |
−11.96 |
−11.44 |
−12.30 |
−124.82 |
−118.25 |
−148.70 |
0.38 |
0.36 |
0.46 |
308 |
−6.51 |
−6.14 |
−5.88 |
318 |
−4.98 |
−4.51 |
−3.90 |
3.2.5 Adsorption of mixed dyes. Industrial effluents usually coexists with several dyes. Therefore, the adsorption performances of the synthesized MMoS2/PAA-80 for mixed dyes were also studied in this work. As observed in Fig. 4a, the MMoS2/PAA-80 showed high removal efficiencies for various concentration combinations of mixed-dye solutions. With increasing the concentrations of mixed dyes, the removal efficiencies slightly reduced due to that the vacant active sites on the MMoS2/PAA-80 were saturated by the dye molecules in solutions as the concentration higher than certain value. However, high removal efficiency (>85%) even for the highest combined concentrations (CMB = CCV = CBF = 120 mg L−1) indicated a superior adsorption performance of the MMoS2/PAA-80 for mixed dyes. Moreover, MMoS2/PAA-80 demonstrated slightly higher removal efficiency of MB than of BF and CV for all the mixed-dye solutions. This was probably owing to that the adsorption of MB in mixed solutions restricted the adsorption of BF and CV by steric effects or preferential occupation of active sites on the MMoS2/PAA-80, resulted from relative larger molecular weights of BF and CV and their distinct structure features (Fig. S5†).
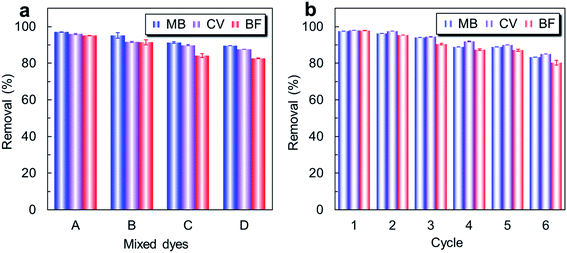 |
| Fig. 4 (a) Removal efficiencies of MB, CV and BF in mixed-dye solutions with various concentration combinations by MMoS2/PAA-80. The four concentration combinations of the mixed dye solutions as: sample A: CMB = CCV = CBF = 60 mg L−1, sample B: CMB = CCV = CBF = 80 mg L−1, sample C: CMB = CCV = CBF = 100 mg L−1, sample D: CMB = CCV = CBF = 120 mg L−1. Conditions: pH = 7.0, m/V = 10 mg/30 mL, T = 25 °C and contact time = 5 min. (b) Reusability of MMoS2/PAA-80 for dye removal. Conditions: CMB = CBF = 200 mg L−1, CCV = 100 mg L−1, pH = 7.0, m/V = 10 mg/30 mL, T = 25 °C and contact time = 5 min. | |
3.2.6 Regeneration performance. The regeneration ability of an adsorbent play an important role in its practical application. The loaded Fe3O4 NPs on the MoS2 nanosheets endowed it excellent separability from polluted water under an EMF (Fig. 1h), therefore, the dye-saturated nanocomposite could be readily recovered and regenerated using NaOH ethanol solutions (0.1 M). The regenerability was evaluated by the removal efficiency of MMoS2/PAA-80 for MB, CV and BF in six successive cycles as presented in Fig. 4b. The removal efficiencies of three dyes slightly reduced with increasing the cycles due to the loss of small amount of adsorbent during the washing process. However, over 80% removal efficiency were still achieved even it was suffered from six successive cycles of adsorption/desorption process, indicating that the MMoS2/PAA owns excellent regenerability and is promising to serve as an excellent adsorbent for treating cationic dye wastewater.
4. Conclusions
In summary, polyacrylic acid (PAA) decorated magnetic MoS2 nanosheets (MoS2/PAA) with high adsorption capacities for three cationic dyes of basic fuchsin (BF), methylene blue (MB), and crystal violet (CV) have been successfully synthesized by a simple solvothermal method. PAA with rich anionic groups (–COOH) played a crucial role in significantly enhancing the dye adsorption. Ultrafast adsorption rate and high adsorption capacities of BF, MB and CV onto MMoS2/PAA are mainly driven by strong electrostatic interaction between cationic dyes and the anionic MMoS2/PAA surface. The adsorption capacities of three dyes dramatically increased with increasing the binding amount of PAA. The adsorption behaviors of dyes onto MMoS2/PAA were well fitted with the pseudo-second-order kinetic and the Langmuir models. The batch adsorption experiments with the effects of parameters like solution pH, contact time, initial dye concentrations and temperature on the adsorption were optimized for dye adsorption. Moreover, the MMoS2/PAA also showed high removal efficiencies for various mixed-dye solutions and excellent regenerability. Such a functional nanocomposite with ultrafast adsorption rate and high adsorption capacities for cationic dyes can serve as an excellent adsorbent for effective removal of variety of cationic contaminants from industrial effluents.
Conflicts of interest
There are no conflicts to declare.
Acknowledgements
This work was supported by the National Natural Science Foundation of China (21676219), the Sichuan Science and Technology Program (2019YJ0254), and the Fundamental Research Funds for the Central Universities, Southwest Minzu University (2020PTJS20010).
Notes and references
- K. M. Lee, C. W. Lai, K. S. Ngai and J. C. Juan, Water Res., 2016, 88, 428 CrossRef CAS PubMed.
- V. Katheresan, J. Kansedo and S. Y. Lau, J. Environ. Chem. Eng., 2018, 6, 4676 CrossRef CAS.
- E. N. E. Qada, S. J. Allen and G. M. Walker, Chem. Eng. J., 2008, 135, 174 CrossRef.
- L. Fan, C. Luo, X. Li, F. Lu, H. Qiu and M. Sun, J. Hazard. Mater., 2012, 215–216, 272 CrossRef CAS PubMed.
- K. B. Tan, M. Vakili, B. A. Hord, P. E. Poh, A. Z. Abdullah and B. Salamatinia, Sep. Purif. Technol., 2015, 150, 229 CrossRef CAS.
- S. Natarajan, H. C. Bajaj and R. J. Tayade, J. Environ. Sci., 2018, 65, 204 Search PubMed.
- M. Rafatullah, O. Sulaiman, R. Hashim and A. Ahmad, J. Hazard. Mater., 2010, 177, 70 CrossRef CAS PubMed.
- K. Lu, G. X. Zhao and X. K. Wang, Chin. Sci. Bull., 2012, 57, 1223 CrossRef.
- I. Ali, A. A. Basheer, X. Y. Mbianda, A. Burakov, E. Galunin, I. Burakova, E. Mkrtchyan, A. Tkachev and V. Grachev, Environ. Int., 2019, 127, 160 CrossRef CAS PubMed.
- A. Gopalakrishnan, S. P. Singh and S. Badhulika, New J. Chem., 2020, 44, 5489 RSC.
- X. Q. Qiao, F. C. Hu, F. Y. Tian, D. F. Hou and D. S. Li, RSC Adv., 2016, 6, 11631 RSC.
- Z. Y. Wang and B. X. Mi, Environ. Sci. Technol., 2017, 51, 8229 CrossRef CAS PubMed.
- C. Liu, F. F. Jia, Q. M. Wang, B. Q. Yang and S. X. Song, Appl. Mater. Today, 2017, 9, 220 CrossRef.
- S. Yang, M. X. Hua, L. Shen, X. L. Han, M. Y. Xu, L. J. Kuang and D. B. Hua, J. Hazard. Mater., 2018, 354, 191 CrossRef CAS PubMed.
- H. J. Song, S. S. You, X. H. Jia and J. Yang, Ceram. Int., 2015, 41, 13896 CrossRef CAS.
- A. S. K. Kumar, S. J. Jiang and J. K. Warchoł, ACS Omega, 2017, 2, 6187 CrossRef PubMed.
- Y. Chen, B. H. Song, X. S. Tang, L. Lu and J. M. Xue, Small, 2014, 10, 1536 CrossRef CAS PubMed.
- T. M. F. Marques, D. A. Sales, L. S. Silva, R. D. S. Bezerra, M. S. Silva, J. A. Osajima, O. P. Ferreira, A. Ghosh, E. C. S. Filho, B. C. Viana and J. M. E. Matos, Appl. Surf. Sci., 2020, 512, 145659 CrossRef CAS.
- Y. B. Song, S. N. Lv, C. J. Cheng, G. L. Ni, X. W. Xie, W. Huang and Z. G. Zhao, Appl. Surf. Sci., 2015, 324, 854 CrossRef CAS.
- J. W. Zhang, M. S. Azam, C. Shi, J. Huang, B. Yan, Q. X. Liu and H. B. Zeng, RSC Adv., 2015, 5, 32272 RSC.
- R. Samadder, N. Akter, A. C. Roy, M. M. Uddin, M. J. Hossen and M. S. Azam, RSC Adv., 2020, 10, 11945 RSC.
- X. D. Liu, J. F. Tian, Y. Y. Li, N. F. Sun, S. Mi, Y. Xie and Z. Y. Chen, J. Hazard. Mater., 2019, 373, 397 CrossRef CAS PubMed.
- D. W. Cho, B. H. Jeon, C. M. Chon, F. W. Schwartz, Y. Jeong and H. Song, J. Ind. Eng. Chem., 2015, 28, 60 CrossRef CAS.
- J. Zhang, J. M. Soon, K. P. Loh, J. Yin, J. Ding, M. B. Sullivian and P. Wu, Nano Lett., 2007, 7, 2370 CrossRef CAS PubMed.
- D. Su, S. Dou and G. Wang, Adv. Energy Mater., 2014, 5, 1401205 CrossRef.
- A. T. Massey, R. Gusain, S. Kumari and O. P. Khatri, Ind. Eng. Chem. Res., 2016, 55, 7124 CrossRef CAS.
- N. J. Fu, L. T. Li, K. J. Liu, C. K. Kim, J. Li, T. Zhu, J. H. Li and B. K. Tang, Talanta, 2019, 197, 567 CrossRef CAS PubMed.
- Y. Y. Xu, M. Zhou, H. J. Geng, J. J. Hao, Q. Q. Ou, S. D. Qi, H. L. Chen and X. G. Chen, Appl. Surf. Sci., 2012, 258, 3897 CrossRef CAS.
- W. J. Xu, M. S. Wang, Z. W. Li, X. J. Wang, Y. Q. Wang, M. Y. Xing and Y. D. Yin, Nano Lett., 2017, 17, 2713 CrossRef CAS PubMed.
- Q. W. Wang, S. Y. Dong and D. Zhang, J. Mater. Sci., 2018, 53, 1135 CrossRef CAS.
- Q. Y. Lv, Y. Shen, Y. Qiu, M. Wu and L. M. Wang, J. Appl. Polym. Sci., 2020, 137, e49322 CrossRef.
- L. H. Ai, C. Y. Zhang and Z. L. Chen, J. Hazard. Mater., 2011, 192, 1515 CrossRef CAS PubMed.
- S. Largegren, K. Sven. Vetenskapsakad. Handl., 1898, 24, 1 Search PubMed.
- Y. S. Ho and G. M. Mckay, Proc. Biochem., 1999, 34, 451 CrossRef CAS.
- H. Yan, X. Tao, Z. Yang, K. Li, H. Yang, A. M. Li and R. S. Cheng, J. Hazard. Mater., 2014, 268, 191 CrossRef CAS PubMed.
- I. Langmuir, J. Am. Chem. Soc., 1916, 38, 2221 CrossRef CAS.
- H. M. F. Freundlich, J. Phys. Chem., 1906, 57, 385 CAS.
Footnote |
† Electronic supplementary information (ESI) available: High-resolution XPS spectra of Mo 3d and S 2p in MoS2 and MMoS2/PAA-80 samples; digital photographs of MMoS2/PAA-80 and MMoS2 aqueous dispersions after keeping different times; zeta potentials of MMoS2 and MMoS2/PAA-80; fitting of pseudo-first-order kinetic model of BF, MB, and CV adsorption onto MMoS2/PAA-80; molecular structures of methylene blue (MB), basic fuchsin (BF), and crystal violet (CV); effect of temperature on the adsorption of BF, MB, and CV onto MMoS2/PAA-80. See DOI: 10.1039/d1ra01548f |
|
This journal is © The Royal Society of Chemistry 2021 |
Click here to see how this site uses Cookies. View our privacy policy here.