DOI:
10.1039/D1RA02517A
(Paper)
RSC Adv., 2021,
11, 27937-27949
Novel sulphonic acid liquid crystal derivatives: experimental, computational and optoelectrical characterizations†
Received
30th March 2021
, Accepted 21st July 2021
First published on 17th August 2021
Abstract
A novel liquid crystal homologous series based on the benzene sulphonic acid moiety, namely (E)-4-((4-((4-(alkoxy)benzoyl)oxy)benzylidene)amino)benzenesulfonic acid (Sn), was synthesized and examined via different experimental and theoretical measurements. The four synthesized members have terminally connected alkoxy chain groups, which vary between 6 and 12 carbons. FT-IR and NMR spectroscopy, as well as elemental analyses, were used to confirm their molecular structures. Mesomorphic and optical investigations of the prepared homologues were also conducted using differential scanning calorimetry (DSC) and polarized optical microscopy (POM). The DSC and POM characterization revealed that all of the synthesized sulphonic acid members are monomorphic, exhibiting a pure smectic A (SmA) mesophase with enantiotropic properties. Moreover, all compounds in the group have high thermal transition temperatures. The terminal electron-withdrawing group –SO3H plays a considerable role in the stabilization of the molecule, which in return resulted in high thermal SmA stability. Furthermore, the experimental data relating to the mesophase behavior were substantiated via computational studies using the DFT approach. In addition, the terminal –SO3H moiety has an essential impact on the thermal and physical parameters of possible geometries. All members of the synthesized Sn series exhibit ohmic behavior with electrical resistance in the GΩ range, as revealed by electrical measurements. The S10 electrode had the highest electrical conductivity: 35.16 pS. It also showed two direct optical band gaps of 3.58 and 3.23 eV with Urbach energies of 1261.1 and 502.4 meV. Upon decreasing the number of carbon atoms to n = 6, the main bandgap for S6 reduced to 3.3 eV. The highest conductivity, good absorption, and two large bandgaps recorded for the chain derivative S10 make it suitable for investigations relating to energy-based applications.
1. Introduction
Nowadays, organic solar cells are highly promising and cost-effective compared to traditional cells. As such, a large number of research studies on the application of organic compounds as photosensitizers for solar cells have been documented.1–4 Moreover, low molar mass molecule solar cells possess great potential.5–9 Innovative properties of organic solar cells, such as light-weight, flexibility, low cost, and solution processability, have attracted considerable attention from researchers and technological engineering. Furthermore, the modern organic solar cell types have proven to be commercially inexpensive with excellent efficiencies.10 For solar energy applications, such as catalytic photo-degradation of dyes, solar hydrogen generation, photo-electrochemical water splitting, and solar cells, bandgap engineering and optical property control are critical parameters of our interest.11–15
Recently, mesomorphic materials have been proven to have broad technological applications as light emitting diodes, displays and photoconductors.16–18 The impact of symmetrical cells filled with liquid crystal (LC) compounds has been previously investigated.19–21 In order to enhance the ion conductivity of engineered materials, they should be incorporated with a liquid crystalline derivative.22 The smectic ordering of liquid crystals, including ion-conductive tails, results in phases with alternating ion conductive/insulating layers.23,24 Moreover, the mesogen cores increase the ion-conductive tails between the insulating parts, thereby influencing in-plane ion transport. Similar behaviors of anisotropic character have been documented within LC polymer mesophases.23,25
The design of new structural shapes to achieve the desired applicable properties in industry is one of our interests.26–30 Thus, the selection of the terminal tail, the terminal and lateral polar substituents, and the mesogenic cores are important criteria in developing novel mesomorphic materials for proper characteristic technological applications. In addition, the molecular geometry enables some considerable modifications in the mesophase properties and plays an important role in the formation, kind, and thermal mesomorphic stability of the reported mesophases.26–30
Liquid crystalline systems with a terminal acid moiety have the ability to form strong supramolecular interactions.31–34 There is a report of LCs formed through the interaction of aromatic carboxylic acids.35 In addition, several types of materials have been formed by the interaction of complementary molecules with their LC behavior being crucially dependent on the shape of the resulting supramolecular systems. Generally, dimerization links connecting two molecular species36,37 have been proven to be optically anisotropic, which agrees with the main properties of liquid crystal molecules.
On the other hand, the inclusion of electron terminal polar functional groups in the LC skeleton may strongly influence their polarizability and/or polarity, as well as their geometric structures. Consequently, this affects the transition phase temperature, kind of mesophase and other physical and geometrical parameters essential for better characteristics of the LC materials.38–41
The Schiff base moiety maintains the rigidity as well as the linearity of the molecular geometry, which enhances the mesomorphic stability. More reports of low molecular mass Schiff base systems and twist bend nematic mesophases have been investigated.42–44 On the other hand, the conjugative interactions between the –COO- group and the phenyl rings play an important role in getting better mesomorphic behaviour. Moreover, the terminal flexible chains or polar compact substituents have essential roles in the phase transition properties.45
The goal of our present work is to synthesize new azomethine derivatives of a terminal sulphanilic acid moiety, with changeable terminal alkoxy chain length, namely (E)-4-((4-((4-(alkoxy)benzoyl)oxy)benzylidene)amino)benzenesulfonic acid, Sn. This study aims to investigate the mesophase properties of the prepared homologues series via experimental and theoretical approaches. Additionally, we intend to evaluate the effect of the different terminal length of the attached alkoxy chain on their mesomorphic properties. To achieve our objectives, electric and optical property, electric resistance, conductance, energy gap and Urbach energy measurements were conducted on the synthesized new azomethine derivatives. Furthermore, a computational approach was employed to corroborate the experimental data.
2. Experimental
2.1. Synthesis
Hydrazones and Schiff bases are important precursors in the synthesis of various valuable organic compounds that are employed in a variety of applications.46–52 The present homologue Sn was prepared according to the following scheme (Scheme 1):
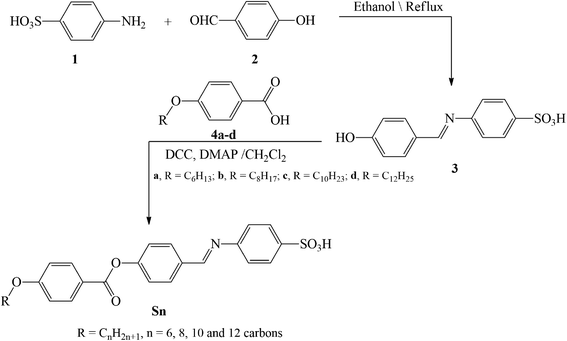 |
| Scheme 1 Synthesis route to the title Sn compounds. | |
2.1.1. Synthesis of (E)-4-((4-hydroxybenzylidene)amino)benzenesulfonic acid (3). Yellow solid, m.p. = 312–314 °C, (Lit m.p. > 300 °C).53
2.1.2. Synthesis of (E)-4-((4-((4-(alkoxy)benzoyl)oxy)benzylidene)amino) benzenesulfonic acid, Sn. The method is provided in the ESI† with all of the physical data of the products Sn. The 1H-NMR and 13C-NMR spectra of derivative S6 are depicted in Fig. 1, as a representative example.
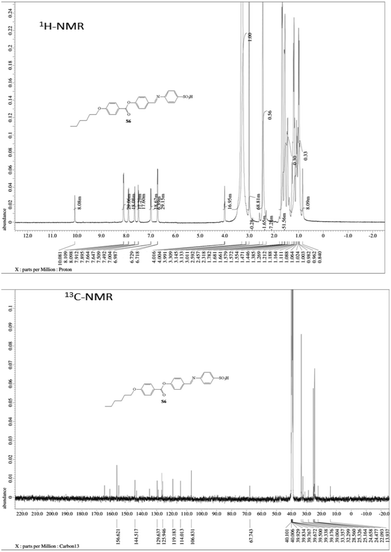 |
| Fig. 1 1H-NMR and 13C-NMR spectra of compound S6. | |
2.2. Computational details
All of the compounds studied were fully optimized without geometrical restriction using the GAUSSIAN 09 program.54 Their nature of convergence was verified via frequency calculation, which in return affirmed all of the frequencies to be real. Both the frontier-molecular orbitals and the molecular-electrostatic potential surfaces were generated from the formatted check (Fchk) file of the optimized structures. All of the measurements were accomplished using the density functional theory (DFT) approach with the B3LYP method,55,56 while using 6-31g(d,p) as the basis set.
3. Results and discussion
3.1. Mesomorphic behavior of the investigated Sn derivatives
Mesomorphic characteristics of the synthesized benzene sulphonic acid derivatives (Sn) have been investigated by DSC and POM. The DSC thermograms of compound S10 during both heating/cooling scans as a representative example are displayed in Fig. 2. It is observed that upon heating, the materials showed two endothermic peaks of the crystal–smectic A and smectic A–isotropic transitions and these were reversed upon cooling. Optical images of the S6 and S12 derivatives under POM are illustrated in Fig. 3. The SmA mesophase showed a focal conic fan texture, which was identified upon heating and cooling scans. The POM measurements confirmed the DSC analyses. This indicates that these derivatives exhibited monomorphic enantiotropic properties. In general, the transition characteristic observation changes according to the molecular geometry of the prepared materials. Significant endothermic as well as exothermic transitions were observed dependent on the attached terminal flexible chain length group and this could be ascribed to the mesomorphic transitions. Details of the transition temperature results and the enthalpy of transitions for the entire investigated Sn series, as derived from DSC measurements, are listed in Table 1. The transition temperatures of all of the evaluated compounds are graphically depicted in Fig. 4 in order to illustrate the impact of terminal alkoxy-chain length (n) on their mesomorphic behavior.
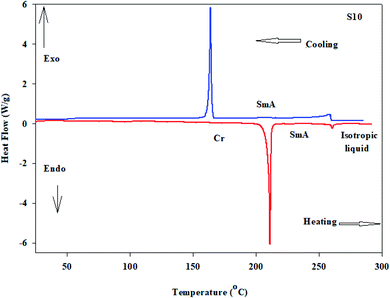 |
| Fig. 2 DSC thermograms of compound S10 at a rate of ±10 °C min−1 recorded from heating and cooling scans. | |
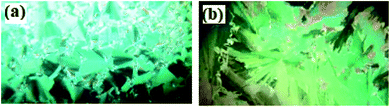 |
| Fig. 3 The focal conic textures of SmA phases observed under POM for the materials (a) S6 at 240.0 °C and (b) S12 at 260.0 °C. | |
Table 1 Mesophase transition temperature (°C), enthalpy of transition ΔH (kJ mol−1), mesomorphic range ΔT (°C), and normalized entropy of transition ΔS/R values for the presented Sn homologuesa
Comp. |
TCr-SmA |
ΔHCr-SmA |
TSmA-I |
ΔHSmA-I |
ΔT |
ΔSSmA-I/R |
Cr-SmA = solid to smectic A mesophase transition. SmA-I = smectic A to isotropic liquid mesophase transition. |
S6 |
215.6 |
37.89 |
254.9 |
2.80 |
39.3 |
0.64 |
S8 |
205.2 |
34.08 |
259.3 |
2.21 |
54.1 |
0.50 |
S10 |
213.2 |
58.01 |
265.0 |
2.03 |
51.8 |
0.45 |
S12 |
220.4 |
47.62 |
284.7 |
3.10 |
64.3 |
0.67 |
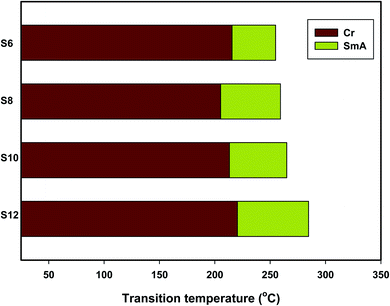 |
| Fig. 4 Effects of the terminal alkoxy chain length (n) on the mesomorphic transitions of the presented Sn homologues. | |
Table 2 and Fig. 4 show that the melting temperatures (Cr-SmA) of the prepared Sn series homologues exhibit an irregular trend, which is less sensitive to the length of the terminal alkoxy chain (n). The melting transition increases as the polarizability of the molecule within the same series increases. However, the observed current trend was not in agreement with this common rule. All synthesized members of the group are mesomorphic in nature with high mesomorphic thermal transitions and a monomorphic enantiotropic mesophase range depending on their flexible terminal length. All of the compounds are purely smectogenic and possess SmA phase with a high thermal stability that increases with the terminal alkoxy chain length.57,58 Compound S6 exhibits the SmA phase with the lowest thermal stability and a temperature range of 254.9–39.3 °C. In the case of the S8 derivative, it possesses an enantiotropic SmA phase with stability and a temperature range close to 259.3–54.1 °C. Also, the S10 homologue exhibits smectogenic stability and a temperature range of nearly 265.0–51.8 °C. However, the derivative bearing the longest terminal chain length (S12) has the highest SmA stability and a broadened temperature range (284.7–64.3 °C). Generally, the molecular geometry, polarizability and dipole moment of the examined materials are highly impacted by the mesomeric-nature of the attached wing groups. Moreover, the mesomorphic character is enhanced by the elevation of the mesogenic core polarity. The mesophase range of the present investigated series increased in the order: S12 > S8 > S10> S6. The mesophase phenomena of calamitic molecules are a direct impact of molecular–molecular interactions that essentially depend on the geometrical shape of the terminal polar groups. The increment of the van der Waals attraction forces between the long terminal alkoxy chains facilitates their lamellar packing and formation of the smectic A phase.
Table 2 Thermal energy, enthalpy, Gibb's free energy, entropy, ionization potential, and electron affinity data for the series at room temperature calculated at the B3LYP/6-31g(d,p) level
Compound |
ZPE, kcal mol−1 |
Thermal, kcal mol−1 |
Enthalpy, kcal mol−1 |
Gibb's free energy, kcal mol−1 |
Entropy, kcal mol K−1 |
I.E., eV |
E.A., eV |
S6 |
305.498 |
326.236 |
326.829 |
259.805 |
224.800 |
5.919 |
2.526 |
S8 |
341.375 |
363.801 |
364.393 |
293.404 |
238.098 |
6.300 |
2.050 |
S10 |
377.129 |
401.288 |
401.881 |
326.490 |
252.860 |
6.294 |
2.050 |
S12 |
412.887 |
438.757 |
439.349 |
359.548 |
267.656 |
6.295 |
2.050 |
The normalized entropy changes (ΔS/R) of mesophase transitions were calculated for the investigated homologues (Sn) and highlighted in Table 1. Irregular trends with small values of ΔS/R associated with the SmA-isotropic transition were observed. This could be attributed to the molecular biaxiality induced by the –COO- linking moiety and the relatively high magnitude of the clearing-temperatures, which in return decreased the SmA-isotropic entropy changes.59–61
3.2. Computational studies
3.2.1. Thermal and geometrical parameters. Computational calculations using DFT were carried out for all of the synthesized compounds (Sn) in order to correlate the estimated quantum chemical parameters and the experimental findings. The theoretical DFT calculations were performed in the gas phase with the DFT/B3LYP method at basis set 6-31G(d,p). Since the synthesized homologues (Sn) are mesomorphic, they should present in a planar conformation. The optimized structure of each member of the present series was affirmed to be stable by the frequency calculation for which no imaginary frequency was predicted for any member (Fig. 5). The zero-point energy and other calculated quantum thermal parameters are highlighted in Tables 2 and 3. They were predicted to increase with the increasing size of the molecule. In the same vein, the Mulliken atomic charges and dipole moment vectors, which are reactivity predictors, are illustrated in Fig. 5. The vector arrow points in the direction of the alkoxy chain for all of the derivatives, thus suggesting the electron donating tendency of the alkoxy terminal owing to its para position. Moreover, the polarity of the derivatives was predicted to increase with the size of the system as indicated by the increasing magnitude of the dipole moment vectors with the alkoxy chain length. On the other hand, it could be seen from Tables 2, 3 and Fig. 5 that all of the derivatives are not completely linear with a slight twisting angle in the core structure. Moreover, the length of the terminal flexible chains has no significant effect on the aromatic ring planarity. It was found that62,63 the planarity of the mesogenic moiety of the mesomorphic compounds is influenced by the electronic nature of the polar attached substituent. Hence, the conjugated π-cloud interactions obtained from the sulphanilic acid moiety play an important role that offers a high thermal stability with good geometrical parameters in the present investigated system.
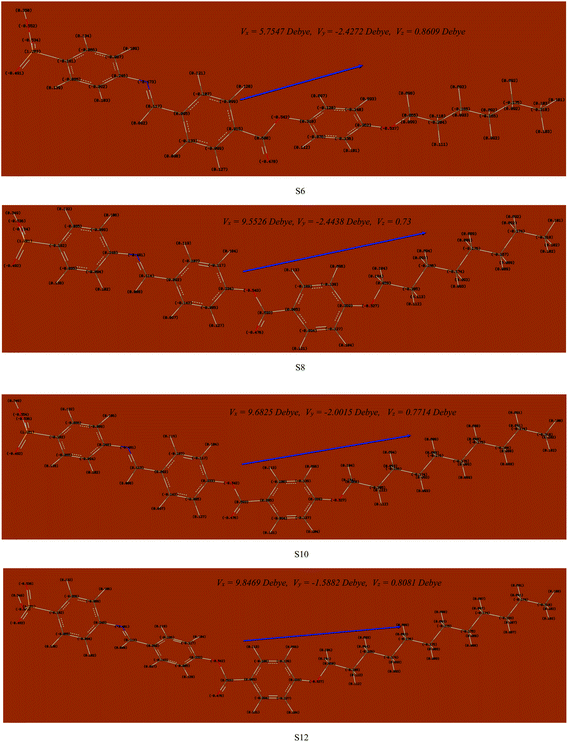 |
| Fig. 5 Atomic charges and dipole moment vectors for the optimized structures of the presented Sn series, predicted at the B3LYP/6-31g(d,p) level. | |
Table 3 HOMO energy, LUMO energy, orbital energy gap, dipole moment, and polarizability data calculated at the B3LYP/6-31g(d,p) level
Compound |
EHOMO, eV |
ELUMO, eV |
ΔE, eV |
Dipole moment, debye |
Polarizability, bohr3 |
S6 |
−5.919 |
−2.526 |
3.393 |
6.305 |
370.67 |
S8 |
−6.300 |
−2.050 |
4.250 |
9.888 |
398.77 |
S10 |
−6.294 |
−2.050 |
4.244 |
9.917 |
421.86 |
S12 |
−6.295 |
−2.050 |
4.245 |
10.007 |
444.79 |
The estimated theoretical data were correlated with the experimental variables of the mesomorphic stability as well as the length of the terminal alkoxy chains (n). It is well known that the mesomorphic stability and kind of mesophase of liquid crystalline materials is mainly dependent on the length of the terminal flexible groups, and this is most often accounted for in terms of molecular geometry.64 Moreover, the polarity of the incorporated substituents, polarizability, rigidity, and shape of the LC molecule are considered important parameters for the enhancement of the type and thermal stability of the mesophases formed. On the other hand, the lower ionization potential (I.E., Table 2) calculated for the shortest terminal length derivative (S6) indicates a more basic nature than others in the series.65 Moreover, a pronounced increment of the dipole moment and polarizability listed in Table 3 are observed with increasing smectic A stability as the length of the alkoxy chain (n) of our investigated compounds (Sn) increases from n = 6 to n = 12 carbons. The rise in dipole moment with increasing number of CH2 in the terminal alkoxy group is in good agreement with the previous report by Davies for the dipole moments of alkyl chlorides in the vapor state.66 The increment of polarizability with terminal length (n) may be attributed to the aspect ratio of the molecules. As the molecular structure aspect ratio increases, the space-filling of the liquid crystalline compound increases, and this results in the enhancement of the polarizability. Furthermore, the dilution of the core–core interactions as the alkoxy chain length increases will influence the polarizability of the whole molecule and increases the intermolecular adhesion forces between molecules, which promotes the degree of molecular ordering and formation of smectic mesophases.
3.2.2. Frontier molecular orbitals (FMOs). For the present investigated compounds (Sn), the frontier molecular orbitals HOMO (highest occupied) and LUMO (lowest unoccupied) analysis depicting the distribution of the HOMO and LUMO in the compounds is presented in Fig. 6 and their resulting energies, as well as energy gaps, are listed in Table 3. The energy gap (ΔE) between the HOMO and LUMO levels is an indicator of the chemical reactivity of the compounds.67 The lower its value, the more reactive the molecule would be. The predicted energy gap included in Table 3 affirms the S6 derivative to be more reactive than the others. It is also softer than others as the energy gap is inversely related to the softness.68 Moreover, in the HOMO of S6, the electron clouds are evenly distributed over the carbon atoms and the π-electron of the terminal phenyl ring attached to the alkoxy chain, but for the others in the series, the distribution extended to the other two phenyl rings. In addition, the similar electron cloud distribution predicted for the HOMO of S8, S10 and S12 could be attributed to their approximately equal HOMO energies. For the LUMO, all of the compounds (Sn) showed similar electron cloud distributions over the carbon atoms and the π-system of the first two phenyl rings attached to the Schiff base linker with the third phenyl ring attached to the alkoxy chain group of S6 being more electron deficient than that of the other counterparts.
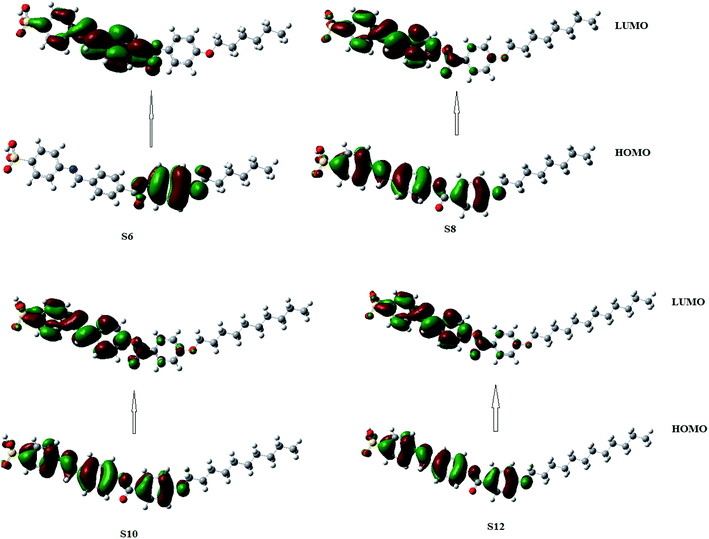 |
| Fig. 6 Estimated FMOs for the synthesized Sn series of homologues. | |
3.2.3. Molecular electrostatic potential (MEP). The molecular geometry of the prepared liquid crystalline material is impacted by the mesomeric-configurations, which are normally affected by the molecular–molecular interactions. One of the best methods for the estimation of the presence of inter or intra-molecular interactions of the evaluated molecules is the MEP. So, the MEP is an indicator that shows the distribution of electron density within a molecule. The MEP for the synthesized homologous series, Sn, was theoretically predicted under the same B3LYP/6-31g(d,p) level of calculation according to MEP (Fig. 7). The MEP analysis predicts low electron density and high electrostatic-potential for the sulphanilic hydrogen atom of all of the investigated compounds in the series (Sn) as it is being shadowed by a blue cloud. On the other hand, the red cloud encircling the oxygen atoms of the sulphanilic acid moiety indicates high electron density. In addition, an appreciable electron density could be noticed for the carbonyl oxygen of the homologous series.
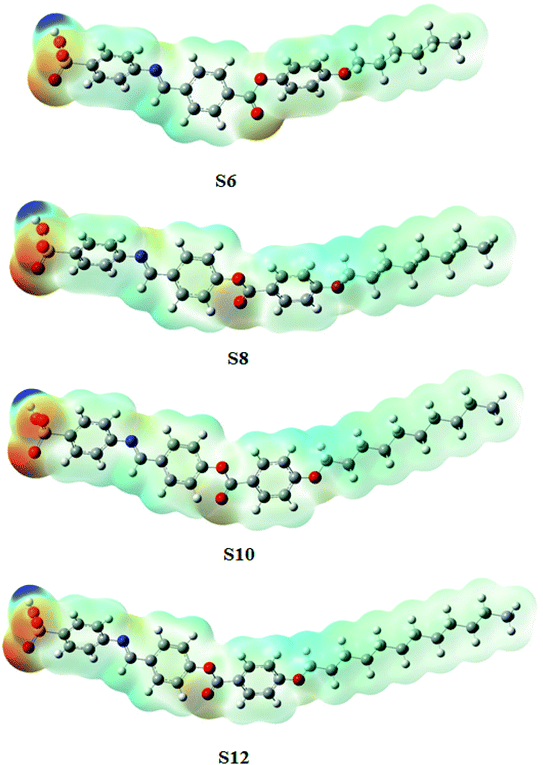 |
| Fig. 7 Molecular electrostatic potential (MEP) plots of the Sn series. | |
3.3. Electric properties
The electrical properties and current–voltage (I–V) characteristics of the investigated Sn series, are measured from −10 V to 10 V with different scan steps, 1 V to 0.005 V, and presented in Fig. 8A and C. The dimensions of the electrode were 22 mm × 22 mm × 0.03 mm. The behaviors are almost linear (ohmic behaviors). As a result, the materials' resistances are nearly constant and independent of the current flowing through them. Recent research has discovered that at low voltage, polymeric and organic systems behave like Schottky diodes. But in the present investigation, the relation between log(I) and V1/2 is non-linear as illustrated in Fig. 8B, which implies that our Sn electrodes do not follow the Schottky diode behavior. Fig. 8A shows how increasing the applied voltage and increasing the carbons to n = 10 increased the current intensity to 0.4 nA at 10 V for the S10 film. As the scan step increased to 1 V, the current intensity is slightly increased, Fig. 8C. As shown in Fig. 8C, the current depends on the voltage and the scan rate. So, the sample seems to have a capacity of some kind. It could be electronic or perhaps ionic. At a specific voltage, the resistive current only depends on voltage and not on the scan rate, while the capacitive current mainly depends on the scan rate. At a given voltage, the capacitance can be calculated by dividing the difference in current by the difference in scan rate, i.e., C = (I1 − I2)/([dV1/dt] − [dV2/dt]) at a specific voltage. The obtained mean value of the capacitance is 148.7 μF for S10 at 5 V. The resistance of the Sn series is decreased by increasing the number of carbons to n = 10. The value of the resistance is decreased from 658.5 GΩ for S6 to 284.4 GΩ for S10. The electrical resistance of the S10 film is decreased from 361.0 GΩ to 273.0 GΩ by increasing the scan step from 0.01 V to 1 V as shown in Fig. 8D. The values of the electrical conductance (σ) were obtained and are shown in Fig. S2† (ESI†). The value of the electrical conductance is increased from 1.52 pS to 35.16 nS by increasing the number of carbons from 6 to 10, Table 4, since the electrical conductance depends mainly on the number and mobility of charge carriers.69–71 By increasing the scan step from 0.01 to 1 V, the film conductance is increased from 27.7 to 36.6 pS. The high resistances and energy band gaps (Section 3.4) imply that the compounds under investigation are dielectrics, with ionic impurities likely causing the materials' conductivity. The compositional heterogeneity of different mobility of these impurities is considered as a potential enhancement of the electrical properties.72,73
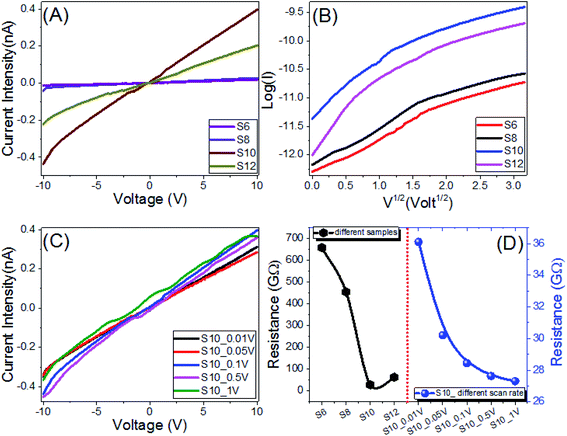 |
| Fig. 8 Electrical characteristics of the Sn series: (A) current–voltage characteristics of the Sn series, (B) log(I) vs. V0.5 plots for the S10 sample at different step scans, (C) current–voltage characteristics of the S10 sample at different step scans, and (D) electrical resistance for the Sn samples and an individual S10 sample at different step scans. | |
Table 4 Values of the electrical conductance (σ), energy gap (Eg), and Urbach energy (EU) for the Sn series
Sample |
σ (pS) |
Eg (eV) |
EU (meV) |
S.D. |
R2 |
S12 |
15.97 |
3.75 |
592.0 |
9.53 |
0.9979 |
S10 |
35.16 |
3.58 |
1261.1 |
34.59 |
0.9947 |
3.23 |
502.4 |
17.29 |
0.9906 |
S8 |
2.20 |
3.40 |
1489.3 |
39.14 |
0.9958 |
S6 |
1.52 |
3.30 |
2136.6 |
67.66 |
0.9930 |
3.4. Optical spectra and energy gap calculations
Fig. 9A and B show how the absorbance spectra of the Sn films are affected by the wavelength of the incident light and the number of carbons of the Sn series. Analysis of the absorbance spectra of S6, S8, and S12, depicted in Fig. 9, show that Sn has a high absorption behavior. For the present Sn series, all of the films display strong absorbance in the UV region and the strongest absorbance was observed for S10 up to 350 nm, Fig. 9A. The absorbance then drops to a plateau up to 880 nm, before dropping again to a minimum absorbance around 1282 nm. Fig. 9C shows a strong absorption band at 298 and 294 nm for S6 and S12, which is blue-shifted by increasing the number of carbons of the prepared Sn series. The S10 sample showed two convoluted absorption bands centered at 293 nm and 344 nm. The absorbance intensity is in the order S10 > S8 > S12. The right edge of the absorption band is blue-shifted by increasing the number of carbons in the Sn series. This red-shift is mainly attributed to the size effects, where large size increases the spin–orbit coupling and controls the exciton positions.74 This strong absorption and wide absorption band in the UV/vis region is a desirable feature for designing energy-efficient solar cells.75 The transmittance spectra of the Sn films, Fig. S3 (ESI†), are affected by the wavelength of the incident light and the number of carbons of the Sn series. All films showed transmission less than 10% up to 360 nm, and then the transmission increased to reach a plateau in the visible light region. Then, the transmission increased exponentially in the near IR region to reach maxima of ∼12.4%, 13.2%, 46.7%, and 57.1% at 1315 nm for the Sn films. After that, the transmission slightly decreased as the wavelength increased.
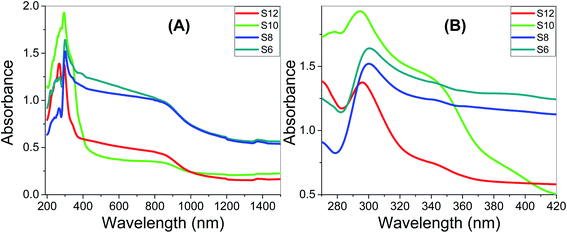 |
| Fig. 9 Optical absorbance spectra of Sn films; (A) wavelength range 200–1500 nm; (B) wavelength range 270–420 nm. | |
According to the optical absorption theorem, the relationship between absorption coefficient, αa, and the photon energy, Eph = hν, h = 6.625 × 10−34 J s−1, for the direct allowed transition is given by:76
|
(αaEph)2 = A(Eph − Eg)
| (1) |
where
Eg is the optical energy gap. The values of direct
Eg for
S6,
S8,
S10, and
S12 are obtained by extending the linear segments of the plot of (
αaEph)
2 vs. Eph to zero as shown in
Fig. 10A. The linear part observed for this figure indicates that the transition is performed directly. Interestingly as reported in
Table 4, there are two direct band gaps for
S10, but one for
S6,
S8, and
S12. The material
S10 may be proceeded to afford a mixture of two geometrical isomers (
E and
Z) and thus, two band gaps of energy were observed during measurements.
77,78 Meanwhile, the other compounds in the series have observed only one isomer with a percent ratio of 100
![[thin space (1/6-em)]](https://www.rsc.org/images/entities/char_2009.gif)
:
![[thin space (1/6-em)]](https://www.rsc.org/images/entities/char_2009.gif)
0 for the
E and
Z isomers. The values of the band gaps for
S10 are 3.23 and 3.58 eV, which are suitable for solar energy applications.
11–15 The energy gap is increased from 3.3 eV to 3.75 eV by increasing the terminal chain length from
S6 to
S12. This increase is ascribed to the influence of the density of localized states. This behavior is consistent with the previously reported studies.
79 The strong absorption and the extension of the bandgap edges are very important for solar energy applications, especially photoelectrochemical hydrogen generation, and solar cells.
80–82
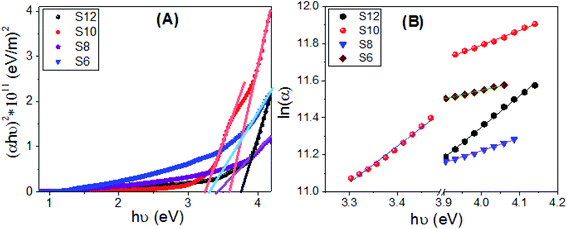 |
| Fig. 10 Calculations of (A) the energy gap and (B) Urbach energy values for S6, S8, S10, and S12 films. | |
Urbach energy (EU) refers to the disorder in the material and represents the width of the exponential absorption edge (Urbach tail of the valence and conduction bands).81 The exponential dependency of EU can be determined according to the following equation:83
|
αa = αao exp(Eph/Eu) → Eu = δEph/δ(ln(αa))
| (2) |
where
αao is the band tail parameter that can be given by:
84where
c is the speed of light,
σo is the electrical conductivity at absolute zero, and Δ
E represents the width of the tail of the localized state in the forbidden gap.
Fig. 10B shows the plot of ln(
α)
vs. hν for the two band gaps of
S6,
S8,
S10, and
S12. The values of
EU were obtained from the slopes of the linear fitting of these curves and reported in
Table 4. The statistical parameters, standard deviation (S.D.) and correlation coefficient (
R2) are also reported in this table. The values are 592.0 ± 9.53 for
S12 and 1261.1 ± 34.59 and 502.4 ± 39.14 eV for
S10, which refers to the extension of the bandgap edges to cover a wide range of the spectral range. For future work and based on the obtained optical properties (absorption,
Eg and
EU), the designed liquid crystalline materials can be further enhanced by incorporating a conductive plasmonic nanomaterial to improve the conductivity and reduce the bandgap.
4. Conclusions
A novel mesomorphic series based on a terminal sulphonic acid moiety, namely (E)-4-((4-((4-(alkoxy)benzoyl)oxy)benzylidene)amino)benzenesulfonic acid (Sn), was synthesized and evaluated for its potential in solar energy applications. Molecular structure elucidation was carried out via elemental analysis, FT-IR spectroscopy, and NMR spectroscopy. Mesomorphic investigations of the prepared homologues were conducted using DSC and POM. An examination of the DSC and POM data revealed that all of the presented synthesized derivatives are monomorphic, possessing a smectogenic (SmA) mesophase with enantiotropic behavior. In addition, all set members have a high thermal transition temperature. The high polarity of the terminal –SO3H group aided in the stabilization of the molecules, which made them achieve high thermal SmA stability. Computational DFT calculations corroborated the recorded experimental values of the mesophase behavior. Moreover, the terminal sulphonic moiety has an essential impact on the thermal and physical parameters of the possible geometries. Energy conversion device measurements affirmed that all of the investigated derivatives exhibit ohmic behavior with electric resistance in the GΩ range, as indicated by electrical property measurements. The highest electrical conductivity, 35.16 pS, was reported for the derivative S10. The energy gap increased from 3.3 eV to 3.75 eV upon increasing the terminal chain length from n = 6 (S6) to n = 12 (S12). Two band gaps were reported for compound S10, 3.23 and 3.58 eV, with band edge tails of 1261.1 ± 34.59 and 502.4 ± 39.14 eV, respectively.
Conflicts of interest
The authors declare no conflicts of interest.
Acknowledgements
The authors acknowledge the Deanship of Scientific Research at Princess Nourah Bint Abdulrahman University through the Fast-track Research Funding Program.
References
- S. Olaleru, J. Kirui, D. Wamwangi, K. Roro and B. Mwakikunga, Perovskite solar cells: the new epoch in photovoltaics, Sol. Energy, 2020, 196, 295–309 CrossRef CAS.
- S. Dong, K. Zhang, B. Xie, J. Xiao, H. L. Yip, H. Yan, F. Huang and Y. Cao, High-performance large-area organic solar cells enabled by sequential bilayer processing via nonhalogenated solvents, Adv. Energy Mater., 2019, 9, 1802832 CrossRef.
- L. M. Gonçalves, V. d. Z. Bermudez, H. A. Ribeiro and A. M. Mendes, Dye-sensitized solar cells: a safe bet for the future, Energy Environ. Sci., 2008, 1(6), 655–667 RSC.
- J. N. Clifford, E. Martinez-Ferrero, A. Viterisi and E. Palomares, Sensitizer molecular structure-device efficiency relationship in dye sensitized solar cells, Chem. Soc. Rev., 2011, 40(3), 1635–1646 RSC.
- S. Badgujar, C. E. Song, S. Oh, W. S. Shin, S.-J. Moon, J.-C. Lee, I. H. Jung and S. K. Lee, J. Mater. Chem. A, 2016, 4, 16335 RSC.
- H. Bin, Y. Yang, Z.-G. Zhang, L. Ye, M. Ghasemi, S. Chen, Y. Zhang, C. Zhang, C. Sun and L. Xue, J. Am. Chem. Soc., 2017, 139, 5085 CrossRef CAS PubMed.
- B. Qiu, L. Xue, Y. Yang, H. Bin, Y. Zhang, C. Zhang, M. Xiao, K. Park, W. Morrison and Z.-G. Zhang, Chem. Mater., 2017, 29, 7543 CrossRef CAS.
- H. Li, Y. Zhao, J. Fang, X. Zhu, B. Xia, K. Lu, Z. Wang, J. Zhang, X. Guo and Z. Wei, Adv. Energy Mater., 2018, 8, 1702377 CrossRef.
- H. Bin, J. Yao, Y. Yang, I. Angunawela, C. Sun, L. Gao, L. Ye, B. Qiu, L. Xue and C. Zhu, Adv. Mater., 2018, 30, 1706361 CrossRef PubMed.
- L. Meng, Y. Zhang, X. Wan, C. Li, X. Zhang, Y. Wang, X. Ke, Z. Xiao, L. Ding and R. Xia, Organic and solution-processed tandem solar cells with 17.3% efficiency, Science, 2018, 361, 1094–1098 CrossRef CAS PubMed.
- A. M. Ahmed, E. M. Abdalla and M. Shaban, Simple and low-cost synthesis of Ba-doped CuO thin films for highly efficient solar generation of hydrogen, J. Phys. Chem. C, 2020, 124(41), 22347–22356 CrossRef CAS.
- M. Shaban and A. M. El Sayed, Influence of the spin deposition parameters and La/Sn double doping on the structural, optical, and photoelectrocatalytic properties of CoCo2O4 photoelectrodes, Sol. Energy Mater. Sol. Cells, 2020, 217, 110705 CrossRef CAS.
- M. Shaban, A. Hamd, R. R. Amin, M. R. Abukhadra, A. F. Khalek, A. Khan and A. Asiri, Preparation and characterization of MCM-48/nickel oxide composite as an efficient and reusable catalyst for the assessment of photocatalytic activity, Environ. Sci. Pollut. Res., 2020, 27, 32670–32682 CrossRef CAS PubMed.
- A. K. Helmy, M. C. Rabia, M. Shaban, A. M. Ashraf, S. A. Ahmed and A. M. Ahmed, Graphite/rolled graphene oxide/carbon nanotube photoelectrode for water splitting of exhaust car solution, Int. J. Energy Res., 2020, 44(9), 7687–7697 CrossRef CAS.
- F. Mohamed, M. Rabia and M. Shaban, Synthesis and characterization of biogenic iron oxides of different nanomorphologies from pomegranate peels for efficient solar hydrogen production, J. Mater. Res. Technol., 2020, 9(3), 4255–4271 CrossRef CAS.
- C. G. Reyes, A. Sharma and J. P. Lagerwall, Non-electronic gas sensors from electrospun mats of liquid crystal core fibres for detecting volatile organic compounds at room temperature, Liq. Cryst., 2016, 43(13–15), 1986–2001 CrossRef CAS.
- S. Setia, S. Sidiq, J. De, I. Pani and S. K. Pal, Applications of liquid crystals in biosensing and organic light-emitting devices: future aspects, Liq. Cryst., 2016, 43(13–15), 2009–2050 CrossRef CAS.
- R. Gupta, V. Manjuladevi, C. Karthik and K. Choudhary, Thin films of discotic liquid crystals and their applications, Liq. Cryst., 2016, 43(13–15), 2079–2091 CrossRef CAS.
- D. Högberg, B. Soberats, M. Yoshio, Y. Mizumura, S. Uchida, L. Kloo, H. Segawa and T. Kato, Self-Assembled Liquid-Crystalline Ion Conductors in Dye-Sensitized Solar Cells: Effects of Molecular Sensitizers on Their Performance, ChemPlusChem, 2017, 82, 834–840 CrossRef PubMed.
- S. Iqbal, Studying discotic liquid crystalline physical gel formation and their applications in solar cells, 2016 Search PubMed.
- M. Bajpai, N. Yadav, S. Kumar, R. Srivastava and R. Dhar, Bulk heterojunction solar cells based on self-assembling disc-shaped liquid crystalline material, Liq. Cryst., 2016, 43, 305–313 CrossRef CAS.
- S. Roddecha and M. Anthamatten, Synthesis and thermotropic behaviour of imidazole-terminated liquid crystals, Liq. Cryst., 2010, 37(4), 389–397 CrossRef CAS.
- M. Yoshio, T. Kato, T. Mukai, M. Yoshizawa and H. Ohno, Mol. Cryst. Liq. Cryst., 2004, 413, 2235–2244 CAS.
- K. Kishimoto, T. Suzawa, T. Yokota, T. Mukai, H. Ohno and T. Kato, J. Am. Chem. Soc., 2005, 127, 15618–15623 CrossRef CAS PubMed.
- K. Hoshino, M. Yoshio, T. Mukai, K. Kishimoto, H. Ohno and T. Kato, J. Polym. Sci., Part A: Polym. Chem., 2003, 41, 3486–3492 CrossRef CAS.
- M. S. Khushaim, H. H. Alalawy, M. M. Naoum and H. A. Ahmed, Experimental and computational simulations of nematogenic liquid crystals based on cinnamic acid in pure and mixed state, Liq. Cryst., 2021, 1–12 CrossRef.
- H. A. Ahmed and M. A. El-Atawy, Synthesis, mesomorphic and geometrical approaches of new non-symmetrical system based on central naphthalene moiety, Liq. Cryst., 2021, 1–13 CrossRef.
- M. A. El-Atawy, O. A. Alhaddad and H. A. Ahmed, Experimental and geometrical structure characterizations of new synthesized laterally fluorinated nematogenic system, Liq. Cryst., 2021, 1–11 CrossRef.
- A. Altowyan, H. Ahmed, S. Gomha and A. Mostafa, Optical and Thermal Investigations of New Schiff Base/Ester Systems in Pure and Mixed States, Polymers, 2021, 13, 1687 CrossRef CAS PubMed.
- S. M. Gomha, H. A. Ahmed, M. Shaban, T. Z. Abolibda, M. S. Khushaim and K. A. Alharbi, Synthesis, Optical Characterizations and Solar Energy Applications of New Schiff Base Materials, Materials, 2021, 14(13), 3718 CrossRef PubMed.
- T. Kato and J. M. J. Fréchet, A new approach to mesophase stabilization through hydrogen bonding molecular interactions in binary mixtures, J. Am. Chem. Soc., 1989, 111, 8533–8534 CrossRef CAS.
- T. Kato and J. M. J. Fréchet, Stabilization of a liquid-crystalline phase through noncovalent interaction with a polymer side chain, Macromolecules, 1989, 22, 3818–3819 CrossRef CAS.
- A. J. Thote and R. B. Gupta, Hydrogen-bonding effects in liquid crystals for applica-tion to LCDs, Ind. Eng. Chem. Res., 2003, 42, 1129–1136 CrossRef CAS.
- M. J. Brienne, J. Gabard, J. M. Lehn and I. Stibor, Macroscopic expression of molecular recognition. Supramolecular liquid crystalline phases induced by association of complementary heterocyclic components, J. Chem. Soc., Chem. Commun., 1989, 24, 1868–1870 RSC.
- G. W. Gray, Molecular Structure and Properties of Liquid Crystals, Academic Press, New York, 1962 Search PubMed.
- H. A. Ahmed and M. M. Naoum, Mesophase behavior of binary and ternary mixtures of benzoic acids bearing terminal substituents of different polarity and chain-lengths, Thermochim. Acta, 2014, 575, 122–128 CrossRef CAS.
- R. I. Nessim and M. I. Nessim, Effect of intermolecular hydrogen-bonding and terminal substituents on the mesophase behavior of binary mixtures of dissimilarly-substituted benzoic acids, Thermochim. Acta, 2010, 511, 27–31 CrossRef CAS.
- P. Jessy, S. Radha and N. Patel, Morphological, optical and dielectric behavior of chiral nematic liquid crystal mixture: study on effect of different amount of chirality, J. Mol. Liq., 2018, 255, 215–223 CrossRef CAS.
- R. Mishra, J. Hazarika, A. Hazarika, B. Gogoi, R. Dubey, D. Bhattacharjee, K. N. Singh and P. R. Alapati, Dielectric properties of a strongly polar nematic liquid crystal compound doped with gold nanoparticles, Liq. Cryst., 2018, 45, 1661–1671 CrossRef CAS.
- L. Al-Mutabagani, L. Alshabanah, H. Ahmed and M. El-Atawy, Synthesis, Optical and DFT Characterizations of Laterally Fluorinated Phenyl Cinnamate Liquid Crystal Non-Symmetric System, Symmetry, 2021, 13, 1145 CrossRef.
- S. A. Al-Zahrani, H. A. Ahmed, M. A. El-Atawy, K. A. Abu Al-Ola and A. Z. Omar, Synthetic, Mesomorphic, and DFT Investigations of New Nematogenic Polar Naphthyl Benzoate Ester Derivatives, Materials, 2021, 14, 2587 CrossRef PubMed.
- A. Nesrullajev and B. Bilgin-Eran, Mesomorphic, morphologic and thermotropic properties of 4-hexyl-N-(4-hexadecyloxysalicylidene) aniline, Mater. Chem. Phys., 2005, 93, 21–25 CrossRef CAS.
- O. Yasa-Sahin, O. Yazici and B. Karaagac, et al., A new liquid crystal of considerable value for the separation of closely related solvents by gas chromatography, Liq. Cryst., 2010, 37, 1111–1118 CrossRef CAS.
- N. Y. Canli, S. Günes and A. Pivrikas, et al., (S)-5-octyloxy-2-[{4-(2-methylbuthoxy)-phenylimino}-methyl]-phenol liquid crystalline compound as additive into polymer solar cells, Sol. Energy Mater. Sol. Cells, 2010, 94, 1089–1099 CrossRef.
- H. Gulbas, D. Coskun and Y. Gursel, et al., Synthesis, characterization and mesomorphic properties of side chain liquid crystalline oligomer having Schiff base type mesogenic group, Adv. Mater. Lett., 2014, 5, 333–338 CrossRef.
- S. M. Gomha, Z. A. Muhammad, H. M. Abdel-Aziz, I. K. Matar and A. A. El-Sayed, Green synthesis, molecular docking and anticancer activity of novel 1,4-dihydropyridine-3,5-dicarbohydrazones under grind-stone chemistry, Green Chem. Lett. Rev., 2020, 13, 6–17 CrossRef CAS.
- S. M. Gomha, A. H. Abdelhady, D. Z. Hassain, A. H. Abdelmonsef, M. El-Naggar, M. M. Elaasser and H. K. Mahmoud, Thiazole-Based Thiosemicarbazones: Synthesis, Cytotoxicity Evaluation and Molecular Docking Study, Drug Des., Dev. Ther., 2021, 15, 659–677 CrossRef PubMed.
- H. R. M. Rashdan, A. H. Abdelmonsef, I. A. Shehadi, S. M. Gomha, A. M. M. Soliman and H. K. Mahmoud, Synthesis, mo-lecular docking screening and anti-proliferative potency evaluation of some new imidazo[2,1-b]thiazole linked thiadi-azole conjugates, Molecules, 2020, 25, 4997 CrossRef CAS PubMed.
- S. M. Gomha and S. M. Riyadh, Synthesis under Microwave Irradiation of [1,2,4]Triazolo[3,4-b][1,3,4]thiadiazoles and Other Diazoles Bearing Indole Moieties and Their Antimicrobial Evaluation, Molecules, 2011, 16, 8244–8256 CrossRef CAS PubMed.
- A. R. Sayed, S. M. Gomha, E. A. Taher, Z. A. Muhammad, H. R. El-Seedi, H. M. Gaber and M. M. Ahmed, One-Pot Synthesis of Novel Thiazoles as Potential Anti-Cancer Agents, Drug Des., Dev. Ther., 2020, 14, 1363–1375 CrossRef CAS PubMed.
- S. Abu-Melha, M. M. Edrees, S. M. Riyadh, M. R. Abdelaziz, A. A. Elfiky and S. M. Gomha, Clean grinding technique: A facile synthesis and in silico antiviral activity of hydrazones, pyrazoles, and pyrazines bearing thiazole moiety against SARS-CoV-2 main protease (Mpro), Molecules, 2020, 25, 4565 CrossRef CAS PubMed.
- S. A. Ouf, S. M. Gomha, M. M. Ewies, A. S. Ouf and I. A. A. Sharawy, Antidermatophytic activity of some newly synthe-sized arylhydrazonothiazoles conjugated with monoclonal antibody, Sci. Rep., 2020, 10, 20863 CrossRef CAS PubMed.
- P. Ruanwas, N. Boonnak and S. Chantrapromma, Five different colours solid-state fluorescence of azastilbenes: a new push-pull p-conjugated system, Bull. Mater. Sci., 2015, 33, 791–795 CrossRef.
- M. J. Frisch, G. W. Trucks, H. B. Schlegel, G. E. Scuseria, M. A. Robb, J. R. Cheeseman, G. Scalmani, V. Barone, B. Mennucci, G. A. Petersson, H. Nakatsuji, M. Caricato, X. Li, H. P. Hratchian, A. F. Izmaylov, J. Bloino, G. Zheng, J. L. Sonnenberg, M. Hada, M. Ehara, K. Toyota, R. Fukuda, J. Hasegawa, M. Ishida, T. Nakajima, Y. Honda, O. Kitao, H. Nakai, T. Vreven, J. A. Montgomery Jr, J. E. Peralta, F. Ogliaro, M. Bearpark, J. J. Heyd, E. Brothers, K. N. Kudin, V. N. Staroverov, R. Kobayashi, J. Normand, K. Raghavachari, A. Rendell, J. C. Burant, S. S. Iyengar, J. Tomasi, M. Cossi, N. Rega, J. M. Millam, M. Klene, J. E. Knox, J. B. Cross, V. Bakken, C. Adamo, J. Jaramillo, R. Gomperts, R. E. Stratmann, O. Yazyev, A. J. Austin, R. Cammi, C. Pomelli, J. W. Ochterski, R. L. Martin, K. Morokuma, V. G. Zakrzewski, G. A. Voth, P. Salvador, J. J. Dannenberg, S. Dapprich, A. D. Daniels, Ö. Farkas, J. B. Foresman, J. V. Ortiz, J. Cioslowski and D. J. Fox, Gaussian 09, Revision A.02, Gaussian, Inc., Wallingford CT, 2009 Search PubMed.
- A. D. Becke, Density-functional exchange-energy approximation with correct asymptotic behavior, Phys. Rev. A: At., Mol., Opt. Phys., 1988, 38, 3098–3100 CrossRef CAS PubMed.
- C. Lee, W. Yang and R. G. Parr, Development of the Colle-Salvetti correlation-energy formula into a functional of the electron density, Phys. Rev. B: Condens. Matter Mater. Phys., 1988, 37, 785–789 CrossRef CAS PubMed.
- C. Imrie and L. Taylor, Liq. Cryst., 1989, 6, 1 CrossRef CAS.
- C. T. Imrie, Liq. Cryst., 2006, 33, 1449 CrossRef CAS.
- T. Donaldson, H. Staesche, Z. Lu, P. Henderson, M. Achard and C. Imrie, Liq. Cryst., 2010, 37, 1097 CrossRef CAS.
- P. A. Henderson, O. Niemeyer and C. T. Imrie, Liq. Cryst., 2001, 28, 463 CrossRef CAS.
- P. A. Henderson and C. T. Imrie, Liq. Cryst., 2011, 38, 1407 CrossRef CAS.
- S. S. Nafee, M. Hagar, H. A. Ahmed, O. A. Alhaddad, R. M. El-Shishtawy and B. M. Raffah, J. Mol. Liq., 2019, 112161 Search PubMed.
- S. S. Nafee, M. Hagar, H. A. Ahmed, R. M. El-Shishtawy and B. M. Raffah, Molecules, 2019, 24, 3032 CrossRef PubMed.
- M. Hagar, H. Ahmed, T. El-Sayed and R. Alnoman, Mesophase behavior and DFT conformational analysis of new symmetrical diester chalcone liquid crystals, J. Mol. Liq., 2019, 285, 96–105 CrossRef CAS.
- S. A. Popoola, A. R. Almohammedi and K. Haruna, Spectroscopic and DFT evaluation of the positional effect of amino group on the properties of aminobenzenesulfonic acid: solvent interaction, Chem. Pap., 2021, 75(6), 2775–2789 CrossRef CAS.
- M. Davies, Some electrical and optical aspects of molecular behavior, The Commonwealth and International Library: Chemistry Division, 1965, p. 507 Search PubMed.
- H. A. Ahmed, M. Hagar, T. H. El-Sayed and R. B. Alnoman, Schiff base/ester liquid crystals with different lateral substituents: mesophase behaviour and DFT calculations, Liq. Cryst., 2019, 46, 1–11 CrossRef CAS.
- H. A. Ahmed, M. Hagar and O. A. Alhaddad, Mesomorphic and geometrical orientation study of the relative position of fluorine atom in some thermotropic liquid crystal systems, Liq. Cryst., 2020, 47(3), 404–413 CrossRef CAS.
- S. Rathi, G. Chauhan, S. K. Gupta, R. Srivastava and A. Singh, J. Electron. Mater., 2017, 46, 1235–1247 CrossRef CAS.
- M. Kumar, S. Kumar, A. Upadhyaya, A. Yadav, S. K. Gupta, A. Singh, Study of charge transport in composite blend of P3HT and PCBM, in AIP Conference Proceedings, AIP Publishing LLC, 2018, vol. 1953 (1), p. 050066 Search PubMed.
- Q. Bian, F. Ma, S. Chen, Q. Wei, X. Su, I. A. Buyanova, W. M. Chen, C. S. Ponseca, M. Linares, K. J. Karki, A. Yartsev and O. Inganäs, Nat. Commun., 2020, 11, 617 CrossRef CAS PubMed.
- K. S. Savage, D. Stefan and S. W. Lehner, Impurities and heterogeneity in pyrite: influences on electrical properties and oxidation products, Appl. Geochem., 2008, 23, 103–120 CrossRef CAS.
- J. Mao, J. Shuai, S. Song, Y. Wu, R. Dally, J. Zhou and Z. Ren, Manipulation of ionized impurity scattering for achieving high thermoelectric performance in n-type Mg3Sb2-based materials, Proc. Natl. Acad. Sci. U. S. A., 2017, 114, 10548–10553 CrossRef CAS PubMed.
- M. Shaban and A. M. El Sayed, Mater. Sci. Semicond. Process., 2016, 41, 323–334 CrossRef CAS.
- S. J. Liu, Z. P. Kan, S. Thomas, F. Cruciani, J. L. Bredas and P. M. Beaujuge, Angew. Chem., Int. Ed., 2016, 55(42), 12996–13000 CrossRef CAS PubMed.
- M. Shaban and A. M. El Sayed, Mater. Sci. Semicond. Process., 2015, 39, 136–147 CrossRef CAS.
- S. S. Nafee, M. Hagar, H. A. Ahmed, R. M. El-Shishtawy and B. M. Raffah, The synthesis of new thermal stable Schiff base/ester liquid crystals: a computational, mesomorphic, and optical study, Molecules, 2019, 24, 3032 CrossRef PubMed.
- N. A. El-Ghamaz, M. A. Diab, A. A. El-Bindary, A. Z. El-Sonbati and H. A. Seyam, Geometrical structure and optical properties of antipyrine Schiff base derivatives, Mater. Sci. Semicond. Process., 2014, 27, 521–531 CrossRef CAS.
- X. Li, J. Guo, L. Yang, M. Chao, L. Zheng, Z. Ma, Y. Hu, Y. Zhao, H. Chen and Y. Liu, Front. Chem., 2019, 7, 362 CrossRef CAS PubMed.
- M. Shaban, M. Rabia, M. G. Eldakrory, R. M. Maree and A. M. Ahmed, Int. J. Energy Res., 2021 DOI:10.1002/er.6326.
- H. S. H. Mohamed, M. Rabia, X.-G. Zhou, X.-S. Qin, G. Khabiri, M. Shaban, H. A. Younus, S. Taha, Z.-Y. Hu, J. Liu, Y. Li and B.-L. Su, J. Mater. Sci. Technol., 2021, 83, 179–187 CrossRef.
- M. Shaban, M. Rabia, M. G. Eldakrory, R. M. Maree and A. M. Ahmed, Efficient photoselectrochemical hydrogen production utilizing of APbI3 (A = Na, Cs, and Li) perovskites nanorods, Int. J. Energy Res., 2021, 45(5), 7436–7446 CrossRef CAS.
- A. M. El Sayed and M. Shaban, Spectrochim. Acta, Part A, 2015, 149, 638–646 CrossRef CAS PubMed.
- S. Sharma, S. Vyas, C. Periasamy and P. Chakrabarti, Superlattices Microstruct., 2014, 75, 378–389 CrossRef CAS.
Footnote |
† Electronic supplementary information (ESI) available. See DOI: 10.1039/d1ra02517a |
|
This journal is © The Royal Society of Chemistry 2021 |
Click here to see how this site uses Cookies. View our privacy policy here.