DOI:
10.1039/D1RA02582A
(Paper)
RSC Adv., 2021,
11, 19747-19754
A highly selective “turn-on” water-soluble fluorescent sensor for gallium ion detection†
Received
1st April 2021
, Accepted 18th May 2021
First published on 1st June 2021
Abstract
In this work, a novel sensor, (E)-N′-(3-(tert-butyl)-2-hydroxybenzylidene)thiophene-2-carbohydrazide (1), based on salicylaldehyde and thiophene hydrazide moieties was designed and synthesized. The single-crystal structure of 1 was achieved and studied for understanding its functional properties. The interaction and recognition abilities of 1 with different metal ions were investigated. Sensor 1 showed excellent “turn-on” fluorescence with highly selective and specific recognition ability in the presence of gallium ions (Ga3+) in an aqueous solution. The sensing behavior of 1 with Ga3+ was also studied by photophysical experiments, ESI-MS analysis, and 1H NMR titration. The limit of detection (LOD) and limit of quantification (LOQ) of 1 for the detection of Ga3+ in an aqueous solution were calculated as 58 nM, and 192 nM, respectively. DFT calculations were carried out to optimize the configuration of 1 and 1–Ga3+ complexes and rationalize the photophysical experimental data. Highly selective test strips based on sensor 1 were developed for Ga3+ detection. Sensor 1 was also used to detect Ga3+ in actual water samples, and a considerable recovery rate was obtained.
Introduction
Gallium is a silvery-white rare metal that does not exist in its simple form in nature.1,2 It exists in trace amounts in bauxite and zinc earth in the form of Ga3+ salt.3 Gallium is employed in the manufacture of many electronic products such as LEDs and solar cells because of its excellent chemical and physical properties.4–10 Ga3+ salt is also used as an anticancer drug because of its excellent biological affinity for tumor tissues.11–13 Owing to its excellent physical, chemical, and biological applications, the use of Ga3+ is gradually increasing, resulting in the gradual accumulation of Ga3+ in the environment. Although Ga3+ plays a critical role in human life activities, disease problems caused by Ga3+ ion exposure cannot be ignored. Long-term exposure to Ga3+ can cause numerous side effects to the human body, such as gastrointestinal diseases, anemia, and coma, and may even lead to death.14,15 However, there are few reports on Ga3+ sensors and even fewer reports on fluorescent sensors. To the best of our knowledge, reports on Ga3+ fluorescent sensors are rare.16–28 Among the reported sensors, few work in aqueous solutions. In addition, most reported Ga3+ sensors recognize not only Ga3+, but also other interfering ions29–33 (such as Al3+ and In3+) simultaneously, which will interfere with the judgment of detection results. A sensor that can specifically recognize a certain ion will avoid misjudgment in actual use. Fluorescent sensors have the characteristics of high sensitivity, good selectivity, convenient operation, and intuitive observation.34–44 Therefore, it is necessary to develop a fluorescent sensor that can recognize Ga3+ specifically and selectively in an aqueous solution.
Acylhydrazone Schiff bases are widely studied because of their simple synthesis, excellent easy-to-adjust photoelectric properties, multiple coordination sites, and easy combination with metal ions.45 In recent decades, a large number of Schiff base-based fluorescent sensors have been reported.45–48 However, most sensors reported are related to identifying common metal ions and heavy metal ions such as aluminum and mercury.49,50 There are limited reports on the sensors of some trace elements (such as Ga3+). Therefore, it is also significant to study trace element sensors. In the past few years, our group has reported some research in fluorescence detection fields.51–54 Based on previous research, a novel fluorescent sensor based on acylhydrazone Schiff base was designed and synthesized in this work. It exhibits high selectivity and specificity for fluorescence “turn-on” recognition of Ga3+ in an aqueous solution. To study the working mechanism and the internal molecular interaction, we grew a single crystal of sensor 1 and carried out photophysical experiments, 1H NMR titration, and ESI-MS mass spectrometry analysis. DFT calculations were also carried out to study the energy levels of the molecular orbitals and the change in the HOMO–LUMO band gaps before and after the coordination process. Moreover, highly selective test strips based on sensor 1 were developed for Ga3+ detection. Sensor 1 was also used to detect Ga3+ in actual water samples. We expect this work will contribute to the development and application in Ga3+ detection fields.
Experimental
Chemicals and solvents
All chemicals and solvents (analytical and spectral) were used as standard and purchased from Sigma-Aldrich.
Measurements and instruments
1H NMR and 13C NMR spectra were recorded using a Bruker AVANCE III HD 400 MHz nuclear magnetic resonance spectrometer and a Bruker AVANCE III HD 700 MHz nuclear magnetic resonance spectrometer. Absorption spectra were recorded using a PERSEE TU-1950 double-beam UV-vis spectrophotometer at room temperature. Fluorescence emission spectra were recorded using a HITACHI F-7000 fluorescence spectrophotometer. Quantum yield was collected using a QuantaurusQY Absolute PL quantum yield spectrometer, C11347-11|Hamamatsu Photonics. ESI-MS spectra were recorded using a Q-TOF 6520 LC-MS AGILENT instrument. IR spectra were recorded using a Thermo IS5 Fourier transform infrared spectrometer. Elemental analysis was performed using an EA3000 elemental analyzer at the Analysis and Testing Center of Beijing Institute of Technology.
Preparation and characterization of 1
(E)-N′-(3-(tert-Butyl)-2-hydroxybenzylidene)thiophene-2-carbohydrazide (1). A typical synthesis of sensor 1 was performed by refluxing a mixture of 3-(tert-butyl)-2-hydroxybenzaldehyde (1.0 mmol) and thiophene-2-carbohydrazide (1.01 mmol) in 10 mL methanol for 24 h. After the reaction was complete, the obtained mixture was cooled to room temperature. The product was filtered off and then washed with cold methanol and cold water. The resulting solid product was obtained in 98% yield as a white powder. 1H NMR (400 MHz, DMSO-d6) δ 12.32 (s, 1H), 12.26 (s, 1H), 8.55 (s, 1H), 7.93 (s, 1H), 7.91 (d, J = 0.9 Hz, 1H), 7.31 (s, 1H), 7.29 (s, 1H), 7.28–7.22 (m, 1H), 6.89 (t, J = 7.7 Hz, 1H), 1.40 (s, 9H). 13C NMR (176 MHz, DMSO-d6) δ 157.43, 156.83, 150.58, 137.17, 136.36, 132.30, 129.52, 129.38, 128.54, 128.21, 118.77, 117.64, 34.46, 29.22. HRMS calcd. C16H18N2O2S 302.1089, calcd. C16H19N2O2S+ 303.1162, found M + H+ 303.1163. Elemental analysis: calcd for C16H18N2O2S: C, 63.55; H, 6.00; N, 9.26; O, 10.58; S, 10.60. Found C, 63.49; H, 5.95; N, 9.04; S, 10.46.
UV-vis titration experiments
A solution of sensor 1 (3 mM, dissolved in DMSO) was prepared, and the sensor 1 solution (10 μL, 3 mM in DMSO) was diluted to 2.990 mL bis–tris buffer solution (pH = 7.4) to achieve a final concentration of 10 μM (0.3% DMSO/H2O). Then, 1–5 μL Ga(NO3)3 (3 mM) aqueous solution was added to 3 mL above 1 solution. After mixing for 5 minutes, UV-vis absorption spectrum experiments were performed at room temperature.
Fluorescence titration experiments
A solution of sensor 1 (3 mM, dissolved in DMSO) was prepared, and the sensor 1 solution (1 μL, 3 mM in DMSO) was diluted to 2.999 mL bis–tris buffer solution (pH = 7.4) to achieve a final concentration of 1 μM (0.03% DMSO/H2O). Then, 1–5 μL of 0.3 mM Ga(NO3)3 aqueous solution was added to 3 mL above 1 solution. After mixing for 5 minutes, fluorescence spectrum experiments were performed at room temperature.
Job's plot measures
First, 0.1 μM sensor 1 bis–tris solution (dissolved in 0.03% DMSO/H2O) and Ga(NO3)3 bis–tris solution were prepared respectively. A series of solutions containing 1 and Ga(NO3)3 were prepared to keep the volume of each solution at 3 mL. The volume ratio of 1 and Ga3+ are 10
:
0, 9
:
1, 8
:
2, 7
:
3, 6
:
4, 5
:
5, 4
:
6, 3
:
7, 2
:
8, 1
:
9, and 0
:
10. After mixing for 5 minutes, the fluorescence data were collected at room temperature. Job's plot curve was obtained by plotting the volume ratio of Ga3+ ion to 1 + Ga3+ ion and the fluorescence intensity on the X and Y axes, respectively.
Competition experiments
A solution of sensor 1 (3 mM, dissolved in DMSO) was prepared, and 1 μL sensor 1 solution (3 mM) was diluted to 2.999 mL bis–tris buffer solution to achieve a final concentration of 1 μM. Solutions of various metal ions (4.5 μL, 20 mM, 30 eq.) including Na+, K+, Ag+, Mg2+, Hg2+, Pb2+, Ca2+, Cd2+, Co2+, Cu2+, Mn2+, Ni2+, Zn2+, Fe2+, Fe3+, Al3+, Cr3+, and Ga3+ were added to each 1 solution (3 mL, 1 μM in bis–tris solutions). Ga(NO3)3 aqueous solutions (4.5 mL, 20 mM, 30 eq.) were then added to the above solutions. After mixing for 5 min, fluorescence spectra were recorded at room temperature.
1H NMR titration
NMR samples of sensor 1 (3.02 mg, 0.01 mmol) were dissolved in DMSO-d6 (500 μL), and different amounts of Ga(NO3)3 (0–0.5 eq.) were added to each sample. After the mixtures were mixed for 30 minutes, 1H NMR spectroscopy was performed at room temperature.
Theoretical calculation
The structures of 1 and 1–Ga3+ were fully optimized by DFT calculations using the Gaussian 09 program.55 The B3LYP functional adopted the 6-31G basis set for H, C, N, O, and S atoms and Lanl2DZ basis set for Ga atoms. The input files were generated using GaussView 6.0 (scaling radii of 75%, isovalue of 0.05). GaussSum 3.0 (ref. 56) was adopted to calculate the contribution of molecular orbitals (MO) in electronic transitions and the theoretical absorption spectrum.
Fluorescent test strips
In order to facilitate rapid detection and analysis, fluorescent test strips for Ga3+ detection were developed. A series of rectangle test strips were prepared by adding 10 μL of 10 μM sensor 1 solution and then dried to make Ga3+ test strips. Following this, 10 μL 10 μM different metal ions (Na+, K+, Ag+, Mg2+, Hg2+, Pb2+, Ca2+, Cd2+, Co2+, Cu2+, Mn2+, Ni2+, Zn2+, Fe2+, Fe3+, Al3+, Cr3+, and Ga3+) were added to each test strip.
Crystallographic data collection and refinement
A suitable single crystal with a size of 0.3 × 0.2 × 0.1 mm for sensor 1 (CCDC number: 2064800) was selected for single-crystal X-ray diffraction analysis. Data collection was conducted using a Bruker-AXS CCD area detector with graphite monochromatic MoKα (λ = 0.71073 Å) radiation in the ω scan mode at 296(2) K. Unit-cell parameters were determined by automatic centering of the reflections. The diffraction data were corrected for Lorentz and polarization effects and absorption (empirically from ψ scan data). Using Olex2-1.3,57 the structure was solved using the SHELXT58 structure solution program by Intrinsic Phasing and refined using the SHELXL59 refinement package by least squares minimization. The relevant crystallographic data for 1 are presented in Table 1, and the selected bond lengths and angles are given in Tables S1 and S2, ESI.†
Table 1 Crystallographic details for sensor 1
Identification code |
1 |
Empirical formula |
C16H18N2O2S |
Formula weight |
302.38 |
Temperature/K |
296.15 |
Crystal system, space group |
Monoclinic, P21/c |
Unit cell dimensions |
a = 19.277(4) Å, α = 90° |
b = 9.640(2) Å, β = 81.711(7)° |
c = 8.8871(18) Å, γ = 90° |
Volume/Å3 |
1634.2(6) |
Z, ρcalc g cm−3 |
4, 1.229 |
μ/mm−1, F(000) |
0.204, 640.0 |
Crystal size/mm3 |
0.3 × 0.2 × 0.1 |
Radiation |
Mo Kα (λ = 0.71073) |
2θ range for data collection/° |
4.27 to 46.184 |
Index ranges |
−21 ≤ h ≤ 21, −10 ≤ k ≤ 10, −9 ≤ l ≤ 9 |
Reflections collected |
12 926 |
Independent reflections |
2295 [Rint = 0.0377, Rsigma = 0.0283] |
Data/restraints/parameters |
2295/0/194 |
Goodness-of-fit on F2 |
1.038 |
Final R indexes [I ≥ 2σ(I)] |
R1 = 0.0567, wR2 = 0.1576 |
Final R indexes [all data] |
R1 = 0.0824, wR2 = 0.1782 |
Largest diff. peak/hole/e Å−3 |
0.39/−0.41 |
Results and discussion
Synthesis and structural characterization of 1
As shown in Scheme 1, 1 was synthesized by direct condensation of 3-(tert-butyl)-2-hydroxybenzaldehyde and thiophene-2-carbohydrazide with a 98.6% yield in a methanol solution and characterized by 1H NMR, 13C NMR, and ESI-MS spectra (see ESI, Fig. S1–S3†). Sensor 1 has good solubility in common organic solvents such as methanol, ethanol, DMF, and DMSO, and slight solubility in water. The single crystal of 1 was achieved by slowly evaporating the solvent (MeOH
:
MeCN = 4
:
1, v/v) at room temperature. The single-crystal data (Fig. 1, Table 1) of 1 were collected using an X-ray single-crystal diffractometer and solved using the Olex2-1.3 program.55 As shown in Fig. 1a, there is an intra-molecular H-bonding (O2–H2⋯N2, 1.87 Å, 2.601 Å, 148.1°) between the hydroxyl proton and the adjacent imine nitrogen, which can reduce the molecular flexibility. However, the rotation of the N–N single bond makes the thiophene ring and the salicylaldehyde part form a dihedral angle with 24.52° (Fig. 1e). Clearly, the molecular rotation of 1 in the solution will be more significant than that in the crystallized state, which contributes to the difference in the solution and solid-state fluorescence emissions (see ESI, Fig. S4†). The molecules of 1 were connected by a strong intermolecular hydrogen bond N1–H1/O1 (1.992 Å, 2.815 Å, 159.9°) into a one-dimensional chain. Then, these 1D chains are stacked by van der Waals interactions along 2D and 3D directions in the crystal lattice (Fig. 1b–d).
 |
| Scheme 1 Synthesis route of 1. | |
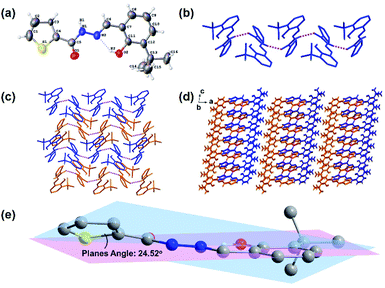 |
| Fig. 1 Views of sensor 1: (a) ORTEP representation showing the crystal structure of 1 with 30% thermal ellipsoid probability; (b) the 1D belt linked by H-bondings (N1–H1⋯O1, 1.992 Å, 2.815 Å, 159.9°); (c) the 2D and (d) 3D structures formed by van der Waals interactions viewed down from the b axis (hydrogen atoms without forming H-bonds are omitted for clarity); and (e) dihedral angle of the molecule of 1. | |
UV-vis absorption studies
The UV-vis absorption spectrum of sensors is an essential photophysical property for the investigation on sensing property. The absorption spectrum of 1 and 1–Ga3+ in a bis–tris buffer solution (about 0.3% DMSO/H2O) was recorded at room temperature. As shown in ESI, Fig. S5,† the UV-vis spectrum of 1 shows two prominent absorption bands at 306 nm and 338 nm, corresponding to π–π* and n–π* transitions, respectively. 1–Ga3+ shows two notable absorption bands at 315 and 405 nm. The maximum absorption peaks of sensor 1 and 1–Ga3+ are 338 and 404 nm, respectively. The binding ability of 1 and Ga3+ ion was initially studied by UV-vis titration analysis. As shown in Fig. 2a, upon the addition of Ga3+ (0–0.5 eq.), the absorption peaks near 306 and 338 nm of 1 gradually decreased. 1–Ga3+ has a new absorption band near 404 nm, which are attributed to the π–π* transition of the thiophene unit and the charge transfer transition in the Ga3+ complex. As a result, the maximum absorption peak shifted from 338 nm to 404 nm. Two clear isosbestic points (266 and 354 nm) indicates the formation of complex 1–Ga3+ between 1 and Ga3+. As shown in Fig. 2b, S6 and S7,† the calculated spectrum shows the calculated maximum absorption peaks of 1 and 1–Ga3+ to be 348 and 406 nm (a similar red-shift occurs from 1 to 1–Ga3+). The calculated results are consistent with the experimental results, validating the experimental results.
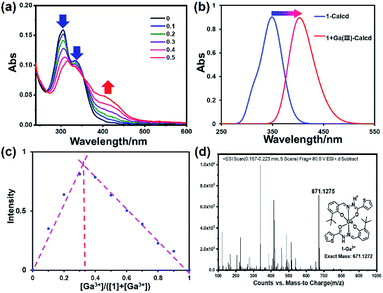 |
| Fig. 2 (a) Absorption spectra of 1 (10 μM) upon the addition of Ga3+ (0–0.5 eq.) in 0.3% DMSO/bis–tris buffer solution. (b) Theoretical calculated UV-vis absorption spectrum of 1 and 1–Ga3+. (c) Job's plot for determining the stoichiometry of 1 and Ga3+ in 0.03% DMSO/bis–tris buffer solution. (d) ESI-MS spectrum of 1–Ga3+. | |
In order to determine the stoichiometry of 1 towards Ga3+, we carried out Job's plot in a bis–tris buffer solution (Fig. 2c). According to Job's plot, when the abscissa value is 0.33, the fluorescence intensity is the strongest, which is equivalent to the sensor-to-ion ratio of 2
:
1. The ESI mass spectrum was regarded as the direct evidence to determine the stoichiometry between metal ions and sensors (Fig. 2d). Furthermore, the ESI mass spectrum of 1–Ga3+ in CH3OH was achieved. The ESI-MS mass spectrum peak at m/z 671.1275, corresponding to [2·1 + Ga3+–2H+]+ (calcd = 671.1272), can be observed when an excess amount of Ga3+ was added to 1, suggesting a 2
:
1 ligand–Ga3+ binding stoichiometry (Fig. 2d). This result is consistent with the result of Job's plot.
In order to further study the linear relationship between 1 and Ga3+, the absorption intensity graph of 1 versus Ga3+ concentrations was achieved (see ESI, Fig. S8†). The association constants (Ka) of 1–Ga3+ complex were calculated to be 8.5 × 103 through Benesi–Hildebrand expression (see ESI, Fig. S9†). In practical applications, LOD and LOQ are important parameters to measure the detection ability of a sensor. The calibration curve was plotted as 1 versus Ga3+ concentrations (0–5 μM), which was found linear [R2 = 0.9951, SD = 0.0002, n = 11, and m = 0.0104]. The LOD and LOQ of sensor 1 towards Ga3+ were calculated according to the eqn (1) and (2),28 and were found as 58 nM, and 192 nM, respectively. In eqn (1) and (2), m refers to the slope of the calibration curve, and SD is the standard deviation of the absorption intensity of different Ga3+ concentrations. The comparative analysis of 1 with previously reported Ga3+ sensors is shown in Table S3, ESI.† It can be seen from the comparison results that sensor 1 has the following advantages: easy synthesis, determined structure, water solubility, lower LOD, and specific recognition of Ga3+.
|
 | (1) |
|
 | (2) |
1H NMR titrations
In order to clarify the binding mode and coordination position of Ga3+ to 1, and better explain the detection mechanism, 1H NMR titration was performed by adding different amounts of Ga3+ (0–0.5 eq.) to sensor 1. The chemical shifts of H atoms of sensor 1 were assigned in the 1H NMR spectrum, as shown in Fig. 3. The N–H (H4) chemical shift is at 12.32 ppm, and O–H (H9) chemical shift is at 12.26 ppm. After the addition of Ga3+ to sensor 1, the 1H NMR spectrum significantly changed (Fig. 3). The chemical shift signals of H4 and H9 gradually weakened. After the addition amount of Ga3+ reached 0.5 eq., chemical shift signals disappear entirely. These results can also verify that the binding stoichiometry of 1 to Ga3+ was 2
:
1. The disappearance of the strong N–H (H4) and O–H (H9) peaks of phenol-OH suggests that H4 participating enol tautomeric carbonyl and H9 involving interaction between the phenolic hydroxyl group and Ga3+. All changes in the 1H NMR spectrum suggest that two molecular of sensor 1 are coordinated with one Ga3+ through two O atoms of the carbonyl group, two azomethine-N atoms, and two phenolic-OH O atoms.
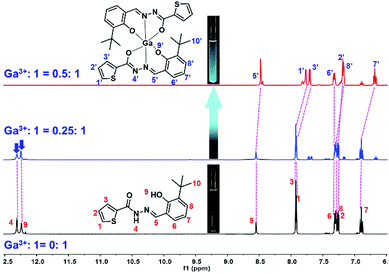 |
| Fig. 3 1H NMR titration of 1 upon the addition of Ga3+ (0–0.5 eq.) in DMSO-d6 (inset: photograph of 1 and 1–Ga3+ in the NMR tube under the irradiation of a 365 nm UV lamp). | |
Fluorescence studies
The fluorescence spectrum of 1 in the presence of various metal ions (Na+, K+, Ag+, Mg2+, Hg2+, Pb2+, Ca2+, Cd2+, Co2+, Cu2+, Mn2+, Ni2+, Zn2+, Fe2+, Fe3+, Al3+, Cr3+, and Ga3+) was studied (Fig. 4). The fluorescence spectra of all these ions were recorded under the same condition. As shown in Fig. 4a and d, apparent spectral changes were observed only in the presence of Ga3+. In contrast, treatment with other metal ions resulted in no apparent phenomenon, evidencing that 1 is a highly selective and specific sensor for Ga3+ over other metal ions. In order to study the anti-interference ability during the detection process of sensor 1, we conducted competitive ion experiments (Fig. 4e). The operation of the competitive ion experiments is to add other metal ions to the 1–Ga3+ solution, and then test the fluorescence intensity of the mixed ion solution. We can observe that the fluorescence intensity of complex 1–Ga3+ was not significantly affected by most of the coexistent metal ions. Therefore, sensor 1 can be used as an effective fluorescent sensor for Ga3+ in the presence of most competing metal ions. In order to observe and compare the effect of fluorescence changes more intuitively, we conducted experiments on sensor 1 with different metal ions in a series of sample bottles (Fig. 4c). The results indicate that the fluorescence in most sample bottles has almost no change, except for those containing Ga3+ under the irradiation of an UV lamp. Compared with 1, the fluorescence intensity of its Ga3+ complex changed dramatically with a strong blue-green fluorescence emission (see ESI, Fig. S10†). However, no obvious fluorescence colour and intensity changed when other metal ions were added to 1 (Fig. 4c). Moreover, because Ga3+ and Al3+ have similar chemical properties, the task of distinguishing them is a well-known challenge.60 Therefore, we also carried out UV titration experiments on Al3+ with sensor 1 (see ESI, Fig. S11†). The result indicates that with the addition of Al3+, even if the amount reaches 30 equivalents, the wavelength of the absorption spectrum does not change significantly. There is also no equivalent absorption point in the titration curve, which is completely different from the titration result of sensor 1 by Ga3+. Therefore, sensor 1 has high selectivity and specificity for recognizing Ga3+.
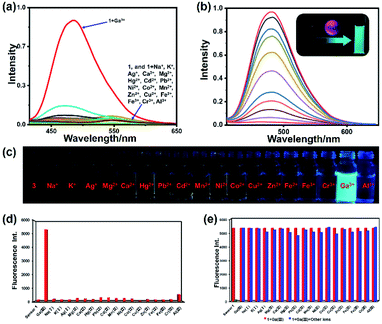 |
| Fig. 4 (a) Fluorescence spectra of 1 (1 μM) with metal ions (30 μM) (Na+, K+, Ag+, Mg2+, Ca2+, Hg2+, Pb2+, Cd2+, Mn2+, Ni2+, Co2+, Cu2+, Zn2+, Fe2+, Fe3+, Cr3+, Al3+ and Ga3+). (b) Fluorescence spectra of 1 (1 μM) upon addition of Ga3+ in 0.03% DMSO/tris–bis buffer solution (inset: photo of 1 and its Ga3+ complex solution under 365 nm UV lamp irradiation). (c) Fluorescence photo of 1 (10 μM) and 1 with metal ions (10 μM) under the irradiation of a 365 nm UV lamp. (d) Fluorescence intensity of 1 (1 μM) with different metal ions. (e) Competitive experiments of 1–Ga3+ toward other metal ions. | |
In order to conduct a more in-depth study of sensor 1 and Ga3+, we performed fluorescence titration experiments on the addition of Ga3+ towards sensor 1 (Fig. 4b). As shown in Fig. 4b, free sensor 1 showed very weak fluorescence emission in the bis–tris buffer solution, which may be caused by the ESIPT effect. Upon the addition of Ga3+ ions (0–0.5 eq.), the fluorescence intensity of 1–Ga3+ increases linearly with a significant enhancement at 480 nm. The quantitative experiment of fluorescence titration can also prove that the probe-to-ion binding ratio is 2
:
1. The fluorescence quantum yields of 1 and 1–Ga3+ (1 μM) are 0.012 and 0.28, respectively. Quantum yield was improved more than 20 times after the binding process.
The time-dependent fluorescence response spectrum shows that it takes 30 seconds to reach the saturated steady-state fluorescence intensity (see ESI, Fig. S12†). The pH effect was also verified because it is an important indicator of sensor applications (see ESI, Fig. S13†). The fluorescence intensity of free sensor 1 remains almost unchanged in the range of pH = 3–12. However, in the presence of Ga3+, the fluorescence ratio showed a strong change in the pH range of 4–8, strongly indicating that sensor 1 is suitable for monitoring Ga3+ under physiological conditions.
Theoretical calculation
The possible binding mode of sensor 1 and Ga3+ was verified by Job's plot, ESI-MS, and 1H NMR titration, that is, sensor 1 and Ga3+ were connected through a 2
:
1 coordination ratio. The possible connection mode is that one gallium atom is coordinated with two imino N atoms, two phenolic hydroxyl O atoms, and two carbonyl O atoms of two 1 ligands. The DFT calculation was performed to optimize the possible molecular structure of 1–Ga3+ based on B3LYP/6-31G(d) (Fig. 5). The TD-DFT calculation was operated to study the spectral change behavior from 1 to 1–Ga3+. As shown in the calculated results, the highest occupied molecular orbital (HOMO) of 1 is mainly located within the benzene ring, acylhydrazine, and Schiff base framework. The lowest unoccupied molecular orbital (LUMO) of 1 is distributed in the π-conjugated skeleton of the intact molecule. The HOMO of 1–Ga3+ is also located within the benzene ring, acylhydrazine, and Schiff base framework. The LUMO of 1–Ga3+ is also distributed in the whole-molecule π-conjugated skeleton. The HOMO and LUMO energy levels of 1 are −5.69 eV and −1.78 eV. In addition, the HOMO and LUMO energy levels of 1–Ga3+ are −2.26 eV and 1.10 eV (Fig. 5, Tables S4–S6†). Therefore, we can obtain the HOMO–LUMO energy gap (Egap) values of 1 and 1–Ga3+ at 3.91 and 3.36 eV through the formula Egap = ELUMO − EHOMO. The energy gap value of 1–Ga3+ is lower than that of 1. The Egap decreased significantly from 1 to 1–Ga3+, indicating that Egap become narrower during the combination process, which means that it takes less energy to excite 1–Ga3+ than 1. Thus, a red-shift in the absorption spectrum occurred from 1 to 1–Ga3+. The Egap gradually becomes narrower from 1 to 1–Ga3+, which means the maximum absorption wavelength gradually becomes more extensive and coincides with the experimental results.
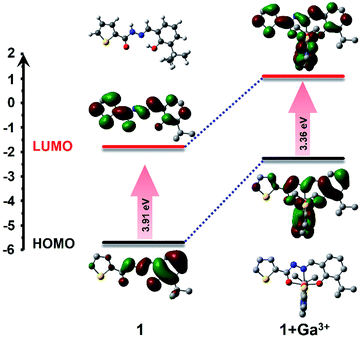 |
| Fig. 5 Optimized molecular structure and HOMO and LUMO orbital levels and energy gap of 1 and 1 + Ga3+, calculated with DFT at the B3LYP/6-31G(d) level using Gaussian 09. | |
Possible sensing mechanism of 1
From the single-crystal structure of 1 (Fig. 1a) and the optimized structure diagram of 1 and 1–Ga3+ (see ESI, Fig. S14 and S15†), we can observe that the flexibility of 1 was restricted through the crystallization and coordination to Ga3+. Due to the existence of intramolecular hydrogen bonds, there is the ESIPT effect in the molecule, which can make the molecular fluorescence very weak. The solid-state fluorescence spectrum was conducted using bulky crystallized samples (see ESI, Fig. S4 and S16†), which is stronger than the solution fluorescence emission, as we expect. The molecular charge distribution was also changed after the coordination with Ga3+, which is favorable to fluorescence emission in the energy level. Therefore, the possible sensing mechanism of 1 was proposed, according to which the interaction of 1 and Ga3+ brings about intra-molecular hydrogen bond (O–H⋯N) cleavage and enol interconversion of the carbonyl group, which inhibits the ESIPT and PET effects (Fig. 6). As a result, 1–Ga3+ showed fluorescence enhancement up to 50 folds.
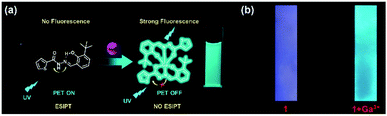 |
| Fig. 6 (a) Proposed sensing mechanism of sensor 1 to Ga3+. (b) Photograph of test paper strips of 1 and 1 + Ga3+ under the irradiation of a 365 nm UV lamp. | |
Application of Ga3+ test strips and determination in water samples
In order to explore the practical application of sensor 1 and make it easily and conveniently applied in our daily lives, we developed test strips based on sensor 1. A series of 3 mM sensor 1 solutions were dropped onto the filter paper strips and dried to obtain test strips. Then, metal ion solutions were added dropwise onto the strips. After a few seconds, under a 365 nm UV lamp, we can observe that the test strip with Ga3+ emitted strong blue-green fluorescence (Fig. 6b). However, the fluorescence of test strips without Ga3+ ions was almost unchanged. This result indicates that simple and easy-prepared test strips can detect Ga3+. As shown in Table 2, we conducted the practical application of sensor 1 in drinking water and tap water. The experimental results of both water samples showed appropriate recovery rates. We also calculated the relative standard deviation (RSD) of the experiment. These results indicate that 1 can be used as a useful sensor for determining the Ga3+ content in actual water samples.
Table 2 Detection of Ga3+ in water samplesa
Sample |
Ga3+ added (μM) |
Ga3+ found (μM) |
Recovery (%) |
RSD% (n = 3) |
Conditions: the concentration of sensor 1 is 1 μM in bis–tris buffer solution. 3 mM Ga3+ was added artificially to the drinking water sample to reach 1.0 μM concentration. 3 mM Ga3+ was added artificially to the tap water sample to reach 1.0 μM concentration. |
Drinking water |
0 |
0 |
— |
— |
1.0b |
0.986 |
98.6 |
0.33 |
Tap water |
0 |
0 |
— |
— |
1.0c |
0.972 |
97.2 |
0.41 |
Conclusions
In summary, we have synthesized and characterized a novel sensor, 1, based on the thiophene acylhydrazone Schiff base. Sensor 1 has outstanding highly selective and specific “turn-on” fluorescence recognition ability for Ga3+ detection in aqueous solutions. The interaction of sensor 1 with Ga3+ has been investigated based on ESI-MS spectrometry, 1H NMR titration, fluorescence emission, and theoretical calculations. The possible sensing mechanism was proposed with the ESIPT effect. The LOD of 1 towards Ga3+ was found to reach a 10−8 M level in an aqueous solution. Moreover, highly selective test strips based on sensor 1 have been developed for Ga3+ detection. Sensor 1 was also used to detect Ga3+ concentration in actual water samples, and a considerable recovery rate was obtained. Based on the results, we believe that this work will contribute to the development and application in gallium detection fields.
Conflicts of interest
There are no conflicts to declare.
Acknowledgements
Thanks for the support of the National Natural Science Foundation of China (No. 21471017).
Notes and references
- L. R. Bernstein, Pharmacol. Rev., 1998, 50, 665 CAS.
- C. Lim, M. An, H. Seo, J. H. Huh, A. Pandith, A. Helal and H. S. Kim, Sens. Actuators, B, 2017, 241, 789 CrossRef CAS.
- D. Yun, J. M. Jung and C. Kim, Inorg. Chim. Acta, 2018, 479, 154 CrossRef CAS.
- H. Dong, H. Zhu, Q. Meng, X. Gong and W. Hu, Chem. Soc. Rev., 2012, 41, 1754 RSC.
- J. Yoon, S. Jo, I. S. Chun, I. Jung, H. S. Kim, M. Meitl, E. Menard, X. Li, J. J. Coleman, U. Paik and J. A. Rogers, Nature, 2010, 465, 329 CrossRef CAS PubMed.
- H. M. Kim, Y. H. Cho, H. Lee, S. I. Kim, S. R. Ryu, D. Y. Kim, T. W. Kang and K. S. Chung, Nano Lett., 2004, 4, 1059 CrossRef CAS.
- R. Trotta, P. Atkinson, J. D. Plumhof, E. Zallo, R. O. Rezaev, S. Kumar, S. Baunack, J. R. Schroter, A. Rastelli and O. G. Schmidt, Adv. Mater., 2012, 24, 2668 CrossRef CAS PubMed.
- J. M. Kikkawa and D. D. Awschalom, Nature, 1999, 397, 139–141 CrossRef CAS.
- J. Goldberger, R. He, Y. Zhang, S. Lee, H. Yan, H. J. Choi and P. Yang, Nature, 2003, 422, 599 CrossRef CAS PubMed.
- A. Kumar, J. Y. Lee and H. S. Kim, Sens. Actuators, B, 2017, 239, 85 CrossRef CAS.
- F. Wang, T. Gao, Q. Zhang, Z. Y. Hu, B. Jin, L. Li, X. Zhou, H. Li, G. V. Tendeloo and T. Zhai, Adv. Mater., 2018, e1806306 Search PubMed.
- S. Crunkhorn, Nat. Rev. Drug Discovery, 2018, 17, 786 CrossRef CAS PubMed.
- M. M. Berggren, L. A. Burns, R. T. Abraham and G. Powis, Cancer Res., 1993, 53, 1862 CAS.
- D. Kara, A. Fisher, M. Foulkes and S. Hill, Spectrochim. Acta, Part A, 2010, 75, 361 CrossRef PubMed.
- H. J. Jang, J. H. Kang, D. Yun and C. Kim, Photochem. Photobiol. Sci., 2018, 17, 1247 CrossRef CAS PubMed.
- V. K. Gupta, A. J. Hamdan and M. K. Pal, Anal. Chim. Acta, 2010, 673, 139 CrossRef CAS PubMed.
- X. He, C. Wu, Y. Qian, Y. Li, F. Ding, Z. Zhou and J. Shen, Talanta, 2019, 205, 120118 CrossRef CAS PubMed.
- X. He, C. Wu, Y. Qian, Y. Li, L. Zhang, F. Ding and J. Shen, Analyst, 2019, 144, 3807 RSC.
- X. He, W. Xiong, C. Xu, J. Fan, Y. Qian, J. Wen and J. Shen, Dyes Pigm., 2020, 174, 108059 CrossRef CAS.
- A. Kumar and P. S. Chae, Anal. Chim. Acta, 2017, 958, 38 CrossRef CAS PubMed.
- F. Cai, F. Xia, Y. Guo, W. Zhu, B. Fu, X. Liang, S. Wang, Z. Cai and H. Xu, New J. Chem., 2019, 43, 18012 RSC.
- V. Kumar, P. Kumar, S. Kumar, D. Singhal and R. Gupta, Inorg. Chem., 2019, 58, 10364 CrossRef CAS PubMed.
- L. Liu, A. Wang, G. Wang, J. Li and Y. Zhou, Sens. Actuators, B, 2015, 215, 388 CrossRef CAS.
- H. Tavallali, P. Vahdati and E. Shaabanpur, Sens. Actuators, B, 2011, 159, 154 CrossRef CAS.
- Z. Wang, Y. Zhang, J. Yin, M. Li, H. Luo, Y. Yang, X. Xu, Q. Yong and S. Wang, Sens. Actuators, B, 2020, 320, 128249 CrossRef CAS.
- X. Zhang, Y. Jiang and N. Xiao, Chem. Commun., 2018, 54, 12812 RSC.
- T. A. Sheikh, M. N. Arshad, A. M. Asiri and M. M. Rahman, New J. Chem., 2018, 42, 13589 RSC.
- M. M. Hussain, A. M. Asiri, M. N. Arshad and M. M. Rahman, New J. Chem., 2018, 42, 1169 RSC.
- H. J. Jang, J. H. Kang, D. Yun and C. Kim, Photochem. Photobiol. Sci., 2018, 17, 1247 CrossRef CAS PubMed.
- Y. W. Wang, S. B. Liu, W. J. Ling and Y. Peng, Chem. Commun., 2016, 52, 827 RSC.
- J. Kimura, H. Yamada, H. Ogura, T. Yajima and T. Fukushima, Anal. Chim. Acta, 2009, 635, 207 CrossRef CAS PubMed.
- J. Y. Noh, S. Kim, I. H. Hwang, G. Y. Lee, J. S. Kang, H. Kim, J. Min, S. Park, C. Kim and J. Kim, Dyes Pigm., 2013, 99, 1016 CrossRef CAS.
- X. He, W. Xiong, C. Xu, J. Fan, Y. Qian, J. Wen and J. Shen, Dyes Pigm., 2020, 174, 108059 CrossRef CAS.
- L. Guo, M. Tian, Z. Zhang, Q. Lu, Z. Liu, G. Niu and X. Yu, J. Am. Chem. Soc., 2021, 143, 3169 CrossRef CAS PubMed.
- J. Kong, C. He, X. Zhang, Q. Meng and C. Duan, Dyes Pigm., 2014, 101, 254 CrossRef CAS.
- S. Sharma, G. Dubey, B. S. Sran, P. V. Bharatam and G. Hundal, ACS Omega, 2019, 4, 18520 CrossRef CAS PubMed.
- F. Shi, S. Cui, H. Liu and S. Pu, Dyes Pigm., 2020, 173, 107914 CrossRef CAS.
- Y. Tang, Y. Ma, J. Yin and W. Lin, Chem. Soc. Rev., 2019, 48, 4036 RSC.
- H. Dong, M. Luo, S. Wang and X. Ma, Dyes Pigm., 2017, 139, 118 CrossRef CAS.
- M. Jiang, X. Gu, J. W. Y. Lam, Y. Zhang, R. T. K. Kwok, K. S. Wong and B. Z. Tang, Chem. Sci., 2017, 8, 5440 RSC.
- Z. Li, M. Ren, L. Wang, L. Dai and W. Lin, Sens. Actuators, B, 2020, 307, 127643 CrossRef.
- W. Fu, C. Yan, Z. Guo, J. Zhang, H. Zhang, H. Tian and W. H. Zhu, J. Am. Chem. Soc., 2019, 141, 3171 CrossRef CAS PubMed.
- Y. Jiao, L. Zhang, X. Gao, W. Si and C. Duan, Angew. Chem., Int. Ed., 2020, 59, 6021 CrossRef CAS PubMed.
- X. Li, X. Gao, W. Shi and H. Ma, Chem. Rev., 2014, 114, 590 CrossRef CAS PubMed.
- K. Boonkitpatarakul, J. Wang, N. Niamnont, B. Liu and L. Mcdonald, ACS Sens., 2015, 1, 144 CrossRef.
- S. M. Hwang, M. S. Kim, M. Lee and M. H. Lim, New J. Chem., 2017, 41, 15590 RSC.
- H. Y. Jeong, S. Y. Lee, J. Han and M. H. Lim, Tetrahedron, 2017, 73, 2690 CrossRef CAS.
- C. Balakrishnan, M. A. Neelakantan and S. Banerjee, Sens. Actuators, B, 2017, 253, 1012 CrossRef CAS.
- J. Fu, B. Li, H. Mei, Y. Chang and K. Xu, Spectrochim. Acta, Part A, 2019, 227, 117678 CrossRef PubMed.
- J. Lian, Q. Xu, Y. Wang and F. Meng, Front. Chem., 2020, 8, 1016 Search PubMed.
- L. Hao, Q. Qiu, W. Wang, L. Gu and H. Li, Chin. J. Chem., 2016, 34, 1109 CrossRef CAS.
- J. B. Song, P. Wang, L. Yan, L. Hao, M. A. Khan, G. L. Liu and H. Li, Dalton Trans., 2020, 49, 4358 RSC.
- P. Wang, L. Liu, F. Meng, M. A. Khan and H. Li, Front. Chem., 2020, 8, 1006 Search PubMed.
- A. Han, H. Su, G. Xu, M. A. Khan and H. Li, RSC Adv., 2020, 10, 23372 RSC.
- M. J. Frisch, G. W. Trucks, H. B. Schlegel, G. E. Scuseria, M. A. Robb, J. R. Cheeseman, G. Scalmani, V. Barone, B. Mennucci, G. A. Petersson, H. Nakatsuji, M. Caricato, X. Li, H. P. Hratchian, A. F. Izmaylov, J. Bloino, G. Zheng, J. L. Sonnenberg, M. Hada, M. Ehara, K. Toyota, R. Fukuda, J. Hasegawa, M. Ishida, T. Nakajima, Y. Honda, O. Kitao, H. Nakai, T. Vreven, J. A. Montgomery Jr, J. E. Peralta, F. Ogliaro, M. Bearpark, J. J. Heyd, E. Brothers, K. N. Kudin, V. N. Staroverov, R. Kobayashi, J. Normand, K. Raghavachari, A. Rendell, J. C. Burant, S. S. Iyengar, J. Tomasi, M. Cossi, N. Rega, J. M. Millam, M. Klene, J. E. Knox, J. B. Cross, V. Bakken, C. Adamo, J. Jaramillo, R. Gomperts, R. E. Stratmann, O. Yazyev, A. J. Austin, R. Cammi, C. Pomelli, J. W. Ochterski, R. L. Martin, K. Morokuma, V. G. Zakrzewski, G. A. Voth, P. Salvador, J. J. Dannenberg, S. Dapprich, A. D. Daniels, O. Farkas, J. B. Foresman, J. V. Ortiz, J. Cioslowski, and D. J. Fox, Gaussian 09, Revision. D.01, Gaussian, Inc., Wallingford CT, 2009 Search PubMed.
- N. M. O'Boyle, A. L. Tenderholt and K. M. Langner, J. Comput. Chem., 2008, 29, 839–845 CrossRef PubMed.
- O. V. Dolomanov, L. J. Bourhis, R. J. Gildea, J. A. Howard and H. Puschmann, J. Appl. Crystallogr., 2009, 42, 339 CrossRef CAS.
- G. M. Sheldrick, Acta Crystallogr., Sect. A: Found. Adv., 2015, 71, 3 CrossRef PubMed.
- G. M. Sheldrick, Acta Crystallogr., Sect. C: Struct. Chem., 2015, 71, 3 Search PubMed.
- S. Y. Lee, K. H. Bok, T. G. Jo, S. Y. Kim and C. Kim, Inorg. Chim. Acta, 2017, 461, 127 CrossRef CAS.
Footnote |
† Electronic supplementary information (ESI) available. CCDC 2064800. For ESI and crystallographic data in CIF or other electronic format see DOI: 10.1039/d1ra02582a |
|
This journal is © The Royal Society of Chemistry 2021 |
Click here to see how this site uses Cookies. View our privacy policy here.