DOI:
10.1039/D1RA04273D
(Review Article)
RSC Adv., 2021,
11, 24722-24746
Potentialities of bioinspired metal and metal oxide nanoparticles in biomedical sciences
Received
2nd June 2021
, Accepted 1st July 2021
First published on 15th July 2021
Abstract
To date, various reports have shown that metallic gold bhasma at the nanoscale form was used as medicine as early as 2500 B.C. in India, China, and Egypt. Owing to their unique physicochemical, biological, and electronic properties, they have broad utilities in energy, environment, agriculture and more recently, the biomedical field. The biomedical domain has been used in drug delivery, imaging, diagnostics, therapeutics, and biosensing applications. In this review, we will discuss and highlight the increasing control over metal and metal oxide nanoparticle structures as smart nanomaterials utilized in the biomedical domain to advance the role of biosynthesized nanoparticles for improving human health through wide applications in the targeted drug delivery, controlled release drug delivery, wound dressing, tissue scaffolding, and medical implants. In addition, we have discussed concerns related to the role of these types of nanoparticles as an anti-viral agent by majorly highlighting the ways to combat the Severe Acute Respiratory Syndrome Coronavirus-2 (SARS-CoV-2) pandemic, along with their prospects.
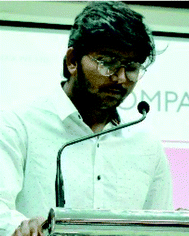 Kshitij R. B. Singh | Mr Singh is a Postgraduate in Biotechnology from Indira Gandhi National Tribal University, Amarkantak, Madhya Pradesh, India. He is currently working in the laboratory of Dr Ajaya Singh, Department of Chemistry, Government V.Y.T. PG Autonomous College Durg, Chhattisgarh, India. He has more than eight peer-reviewed publications to his credit and has authored more than ten book chapters published in the internationally reputed publication houses, namely Elsevier, Springer Nature, IOP Publishing, Wiley, and CRC Press. He has two published, edited books and is currently also involved in editing various books with international publishing houses, including CRC Press, IOP Publishing, Elsevier, and Springer Nature. His research interest is biotechnology, biochemistry, epidemiology, nanotechnology, nanobiotechnology, biosensors, and materials sciences. |
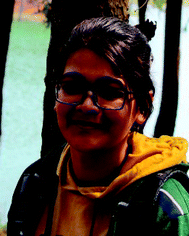 Vanya Nayak | Miss Vanya is pursuing a M.Sc. degree in Biotechnology from the Department of Biotechnology, Indira Gandhi National Tribal University, Amarkantak, Madhya Pradesh, India. She has peer-reviewed journal articles to her credit and has authored more than five book chapters in the internationally reputed press for publications, namely Elsevier, IOP Publication, and CRC Press. Her research interests include nanobiotechnology, nanotechnology, biotechnology, biochemistry, and material chemistry. |
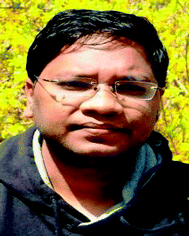 Jay Singh | Dr Singh is an Assistant Professor at the Department of Chemistry, Institute of Sciences, Banaras Hindu University, India, since 2017. He received his PhD degree from MNNIT Allahabad in 2010 and completed his M.Sc. and BSc studies at Allahabad University, Uttar Pradesh, India. He completed his postdoctoral fellowships at NPL, New Delhi, CBNU, South Korea, and DTU, Delhi. He has received many prestigious fellowships like CSIR (RA), DST-Young scientist, DST-INSPIRE faculty award, among others. Furthermore, he is actively engaged in developing smart and intelligent nanomaterials, nanobiocomposite, conducting polymer, and self-assembled monolayers based on clinically important nanobiosensors to estimate various bioanalytes. He has published over 60 international research papers and is editing many books for the internationally reputed press, namely Elsevier, Springer Nature, IOP Publishing, and CRC Press. His research has contributed significantly to understanding interfacial charge transfer processes and sensing aspects of metal nanoparticles. |
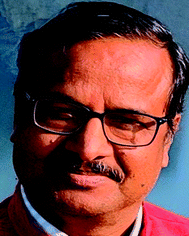 Ajaya Kumar Singh | Dr Ajaya Singh is a Professor of Chemistry at Govt. VYT PG Autonomous College, Chhattisgarh, India. His research interest includes the synthesis and characterization of nano-crystalline solid thin films, nano-oxides, quantum dots and their applications, perovskite materials for solar cells, heterogeneous Fenton's process for the removal of various pollutants, adsorption/removal of heavy metal ions, the biological activity of nanomaterials, and others. He has published more than 160 research papers in peer-reviewed journals and 15 book chapters of international repute. He serves as a reviewer of many reputed international journals. He is also a member of various National and International Chemical Societies. Recently, he became the elected member of the National Academy of Sciences India (MNASI) Allahabad, India. He received a project entitled “Ultrahigh-Efficiency lead-free Perovskite solar Cells” under India-Bulgaria Bilateral Scientific and Technological Cooperation, funded by the Department of Science & Technology. |
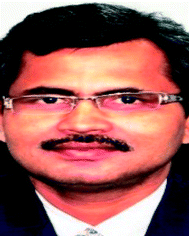 Ravindra Pratap Singh | Dr Singh completed his BSc studies from Allahabad University, India and his M.Sc. and PhD studies in Biochemistry from Lucknow University, India. He is working as an Assistant Professor at the Department of Biotechnology, Indira Gandhi National Tribal University, Amarkantak, India. He has previously worked as a scientist at various esteemed laboratories globally, such as Sogang University, South Korea and IGR, Paris. His work and research interests include biochemistry, biosensors, nanobiotechnology, electrochemistry, material sciences, and applications of biosensors in biomedical, environment, agriculture, and forensics. He has to his credit several reputed national and international honors and awards. Dr Singh authored over 30 articles in international peer-reviewed journals and more than 20 book chapters of international repute. He serves as a reviewer of many reputed international journals, and is also a member of many international societies. He has edited two books and is also editing various books for the internationally reputed publication houses, namely IOP Publishing, CRC Press, Elsevier, and Springer Nature. Moreover, he is the book series editor of “Emerging advances in Bionanotechnology,” CRC Press, Taylor and Francis Group, and the Guest managing editor of Materials Letters, Elsevier, to edit a Special Issue on “Smart and Intelligent Nanobiosensors: Multidimensional applications”. |
1. Introduction
Nanotechnology mainly focuses on the structures of matter from a dimension of the order of less than 100 nm. Nanoparticles with ranged lengths and specific ratios exhibit unique chemistry and physics, awakening abundant properties. In addition, the change in the surface-to-volume ratio is one of the main reasons behind more spontaneous effects. With the decrease in the structure size, the surface-to-volume ratio increases, leading to better chemistry and physics of the conformation than the bulk matter. Nevertheless, these nano-dimensional structures possess extraordinary properties, and it is evident from the fact that nanotechnology relies on the novel properties and behavior shown by nano-range structures of the bulk confirmation of the same matter. These nanoparticles are considered unique as they exist in small size, possess high surface area, surface chemistry and charges, and are therefore known for their multi-functionalities.
Moreover, these nanoparticles are considered reliable drug carriers with a robust, targeted delivery of therapeutic molecules, as they can be easily surface modified for the better attachment of the targeted ligands. Usually, a targeted drug delivery system (DDS) mainly includes four steps: retain, target, evade, and release. It also holds its constituents either by encapsulating, linking, or allowing them to escape the defense mechanism in the body.1–4 Metal and metal oxide nanoparticles and nanomaterials are found in different forms and dimensions (zero-dimensional nanoparticles, one-dimensional nanomaterials, two-dimensional nanomaterials, and three-dimensional nanomaterials). In addition to metal and metal oxide nanoparticles, several other forms of nanostructures, including polymers, liposomes, dendrimers, carbon, and silicon-based nanoparticles, have been successfully utilized for drug delivery and other therapeutic purposes.5,6 Furthermore, Table 1 shows several metal-based nanomaterials that are utilized in various fields for enormous applications.
Table 1 Represents various types of metal-based nanomaterials that are utilized in various domains
S. no. |
Nanoparticle |
Example |
Applications |
1 |
Metal-based nanoparticles |
Manganese (Mn), iron (Fe), silver (Ag), gold (Au), platinum (Pt), selenium (Se), zinc (Zn), and others |
Therapeutics, bio-imaging, electronics, magnetic resonance imaging (MRI), data storage, antimicrobial agent, and textile |
2 |
Doped metal nanoparticle |
Au–CuO, Pt–ZnO, and others |
Antimicrobial, drug delivery, sensors, and others |
3 |
Sulfide-based metal nanoparticle |
FeS, CuS, and others |
Bio-imaging, cancer therapy, drug delivery, diagnosis |
4 |
Metal oxide nanoparticle |
CeO3, ZnO, CuO, and others |
Antimicrobial, biomedical, electronics, optical, detection |
5 |
Metal organic frameworks (MOFs) |
Zn-MOF, Mn-MOF |
Solar cells, super capacitors, fuel cells, sensors, drug delivery, super capacitors, photoelectrocatalysis, and others |
Several works have been reported on nanostructured metal and metal oxides, which are considered future materials for nanobiosensors and exhibit potential applications in the biomedical domain.7–9 These metallic nanoparticles can be synthesized by various biological and physicochemical approaches, and are further studied for their applications. These synthesized nanoparticles show enormous biological properties, namely antimicrobial, anti-inflammatory, anticancer, and anti-angiogenesis, making them good agents to be considered in the biomedical field.10–12 Nowadays, to fight against drug-resistant bacteria, the metallic nanoparticle is combined with antibiotics that enhance the drug's antibacterial activity and are non-toxic to the other cells.13,14 Keeping the same idea in consideration, gentamicin–silver nanoparticles were reported to exhibit excellent antibacterial effects.15
Many years have passed with the successful utilization of metal and metal oxide nanoparticles in the biomedical field for diagnostics and therapeutics. Among all of the metallic nanoparticles, magnesium (Mg), gold (Au), silver (Ag), aluminum (Al), palladium (Pd), cerium (Ce), copper (Cu), iron (Fe), selenium (Se), platinum (Pt), and titanium (Ti) are the most widely used ones because they exhibit remarkable and distinct properties. Many researchers have reviewed the properties, applications, and their synthesis with many other details.16–18 In this review, the major focus is laid on the biomedical aspects of biogenic metal and metal oxide nanoparticles by covering their utilities in both diagnostics and therapeutic applications. The review also highlights metal and metal oxide nanoparticle properties, and simultaneously covers the biogenic synthesis route of metal and metal oxide nanoparticles. Furthermore, a brief overview of the utility of metal and metal oxide nanoparticles during the current pandemic [Severe Acute Respiratory Syndrome Coronavirus-2 (SARS-CoV-2)] has been discussed, as these metals and metal oxide nanoparticles exhibit great anti-viral properties. In short, the review has compiled the up-to-date research works performed on the biogenic metal and metal oxide nanoparticles, giving a detailed literature overview on the topic, and this review work is and will be one of its kind.
2. Physicobiochemical properties
The metal and its oxide-based nanoparticles have an enormous list of properties that include non-toxicity, antimicrobial activity, and anti-insecticidal activity. Therefore, they exhibit vast applications in the biomedical field for the diagnosis and treatment of life-threatening diseases.19 Various metals and metal oxides play an important role in maintaining life processes, and their slight deficiency in the body could lead to several disorders. Here, we focus on several essential biogenic metals and metal oxide nanoparticles that are mainly utilized in the biomedical domain by highlighting their importance in the human body. For instance, a minute amount of cerium can boost an organism's metabolic rate. This leads to enhanced antioxidant and antimicrobial activities, and therefore helps in combating cancer and Alzheimer's disease. Owing to their lack of stability in living systems, the use of nanoceria has often been limited.20–23 Although traditional solid-state reactions are required with some specialized techniques for synthesizing nanoceria, some methods persist with solution-based techniques that utilize hydrothermal, solvothermal, and co-precipitation reactions, providing stability to the nanoceria.24 Nanoceria shows antibacterial activity that acts as an effective oxidant in prophylactic protocols.25 The unique properties of nanoceria have been utilized in ultraviolet absorbance, oxygen sensing, and automotive catalytic converters; for example, nanoceria shows brilliant sensing performance towards methyl orange and can be used in nano-therapeutics to decrease mediators of chronic inflammation.26,27 However, there are reviews available that highlight the green synthesis of cerium oxide nanoparticles and their use in the biomedical domain by discussing their properties.28 Similarly, highly stable, fluorescent and water-resistant cerium oxide nanoparticles have been reported that enrich the soil by increasing catalase and ascorbate peroxidase activity.29
Furthermore, cobalt nanoparticles (CoNPs) have found use in various dimensions as they exhibit good magnetic, optical, mechanical, chemical, and electronic properties. The reduced dimensions of cobalt provide modified, but effective profits in the areas of industrial and biomedical applications.30,31 Compared to other nanoparticles, CoNPs have gained great interest from researchers over time, as they are known to possess high purity and quality, and are therefore widely utilized in magnetic resonance imaging (MRI) and drug delivery. Moreover, they also eliminate the alteration in response stability or magnetization.32 Usually, nanoparticles that show metallic nature showcase magnetic, optical, and catalytic properties, which can be used for developing sensor-based devices, as they possess special features such as small dimensions, high surface-to-volume ratio, and heat transfer. Furthermore, these metal and metal oxide nanoparticles can be utilized to develop anodes of different types that help them develop highly sensitive biosensors.33,34 Similarly, Au NPs exhibit distinctive physical and chemical features that support exploiting various arrays of solicitations in diagnostics, therapeutics, drug delivery, bio-labeling, biological and chemical sensing, imaging, nonlinear optics, photovoltaic and catalysis.35–38 On the other hand, physical properties and the surface plasmon resonance (SPR) band of silver nanoparticles (Ag NPs) depend on their physiology and morphological characters, including shape, size, core charge, surface ligand, and temperature. Similarly, iron nanoparticles' high reactivity with water and oxygen is their greatest drawback, but their catalytic nature helps them find various uses in industrial applications. Moreover, iron nanoparticles' magnetic properties contribute to manipulating the treatment and diagnosis of diseases and applications for fabricating electrical components, transducers, and sensors.39,40 Furthermore, magnesium oxide nanoparticles (MgONPs) possess numerous properties. They can act as anti-biofilm agents, i.e., they inhibit the growth of biofilm and remove pre-established biofilms. They also exhibit self-cleaning activity, helping in the degradation of methyl violet dye, as well as the removal of phosphorus from wastewater, which is one of the reasons for inhibiting plant growth.41–43
Quasi-one-dimensional nanomaterials, such as nanorods, nanotubes, and nanobelts, are widely used to produce chemoresistive nanosensors. Moreover, a drastic change was noticed in MgO nanocrystals when a small amount of base substance was added, showing that the crystals' size increased with increasing temperature and decreased with increasing pH.44 MgO and magnesium hydroxide nanoparticles possess excellent luminescence for photonic applications because they exhibit excellent thermal properties, biodegradability, and non-toxic nature. MgONPs also exhibit potential antibacterial activity, and are utilized in cryoinjury; therefore, they find their utility in medicine.14,45–50 Nickel nanoparticles (NiNPs) are usually very cheap and work even in milder conditions to obtain higher yields of products with less time for reaction. Usually, nickel oxide shows a bandgap from 3.6–4.0 eV, as it is a p-type semiconductor in nature. Nickel nanoparticles have anti-inflammatory properties, and so they are exploited more in the field of biomedicine. NiO has unique characteristics, such as high surface area, fast rate of metal ion release, and good absorption ability, which decreases their cytotoxic effects.51,52 Selenium is the most studied metal nanoparticle. It appears red, black, and metallic grey in color when it is in powder form, vitreous form and crystalline form, respectively. Furthermore, it is a semi-solid metal similar to sulfur or tellurium, and it exists in various oxidation states (2−, 2+, 4+ and 6+). Moreover, various selenoenzymes, such as glutathione peroxidase (GPx), thioredoxin reductases (TrxR), and deiodinases (DIO), have selenium as their core when they are involved in several physiological antioxidant defense systems. Depending on the dose, duration and oxidation state, selenium possesses unique pro-oxidant and antioxidant effects.53–56 Similarly, zinc oxide nanoparticles (ZnO NPs) possess exclusive optical, electrical, catalytic, and photochemical properties utilized mostly in the industrial and biomedical fields. These properties are adjusted by doping with other compounds and adjusting their synthesis conditions.57 These various properties of the metal and metal oxide nanoparticles also help them find their immense potentialities in energy domains. For example, they can be used to develop various energy storage devices, developing batteries58–60 that can be further utilized in the biomedical domain.
3. Biogenic synthesis
Nanoparticle synthesis is the process of creating nanoparticles, and is considered the most important part of nanotechnology because the synthesis route of a nanoparticle highly defines its properties and applications; this can be achieved through physical, chemical or biological routes. Some metals listed in the periodic table are highly utilized in nanoparticle synthesis, and employed in the biomedical field for diagnostic and therapeutic applications. Various techniques like top-down and bottom-up are mostly utilized to synthesize metallic nanoparticles. Top-down approaches mainly break a bulk-piece into nanoscale dimensions with the use of different techniques, like mechanical milling, etching, cutting, and grinding techniques via laser ablation, vapor-phase synthesis and pulsed wire discharge, whereas the bottom-up approach is the chemical reduction, sonochemical reduction, microemulsion, electrochemical, hydrothermal, sol–gel, polypol process, microwave-assisted and biological methods.11 Fig. 1 combines different approaches to synthesize metallic nanoparticles, and this review combines and presents various biogenic routes to synthesize metal and metal oxide nanoparticles. Furthermore, Fig. 2 illustrates the harmful effects of the chemical and physical synthetic routes, and highlights the benefits of the biogenic routes for the synthesis of the metal and metal oxide nanoparticle.
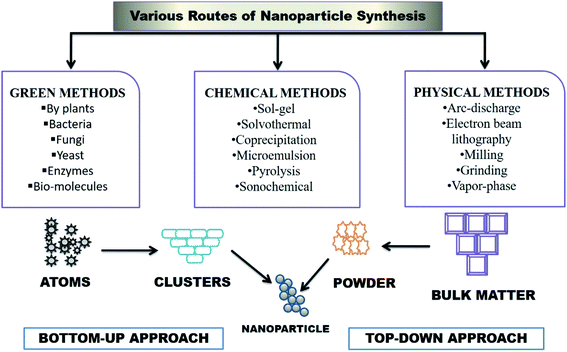 |
| Fig. 1 An illustration of nanoparticle synthesis via different biological/green, chemical, and physical methods, along with top-down and bottom-up. | |
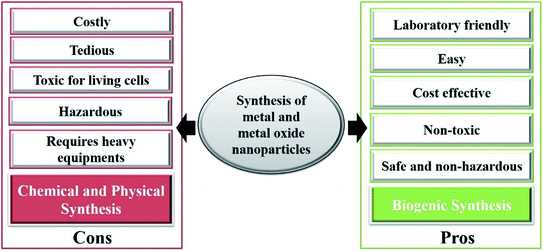 |
| Fig. 2 A schematic illustration showing the positive aspects of green synthesis techniques over chemical and physical routes. | |
A study on green approaches to synthesize metal and metal oxide nanoparticles exclusively includes the utilization of fungi, bacteria, yeasts, and plant extracts, in which plant extracts have been widely used. The various kinds of phytochemicals in plant extracts, namely terpenoids, ketones, flavonoids, aldehydes, amides, and carboxylic acids, play a crucial role in formulating and increasing the bioactivity of the nanoparticles. Unlike chemically synthesized nanomaterials, green synthesized nanomaterials show various distinctive properties, like mechanical, optical, thermal, surface, electrical, chemical, physical and biological. These help them show a variety of potentialities in various domains, like health care, agriculture, environment, robotics, energy, information technology, aeronautics, mass communication, heavy industry, consumer goods, and the development of various sensors, like biosensors, nanosensors, and nanobiosensors. Moreover, these green synthesized metal and metal oxide nanoparticles are used in designing different agents for various diagnostic purposes, therapeutic drug and gene delivery, the development of treatments/cures for several infectious and non-infectious diseases, and neurodegenerative and cardiovascular disorders.61–63 Furthermore, there is a lot of data available in the literature that briefly discuss the various properties, characterization methods, biogenic route of synthesis of metal and metal oxide nanoparticles, and highlight their utilities in various domains, like agriculture, environment, cosmetics, food industries, sensors, among others.19,64–66
The biological approach utilizes various biological molecules as alkaloids, flavonoids, and proteins derived from plant sources to synthesize metal nanoparticles that are mostly accountable for providing stability and the development of biologically active properties.8 Mainly using the extract of plant parts has arisen as a new, innovative, simple, environment-friendly, cheap, strong, and fast technique for synthesizing metal and metal oxide nanoparticles. This biological approach is also known as green chemistry methodology, and is more systematic and effective than using microorganisms and other physical or chemical methods. All plant parts from root to aerial, i.e., root hair, root, stem, leaves, flowers, barks, fruits, peels, seeds and gels, consist of various phytochemicals that provide an efficient base for the synthesis of metal and metal oxide nanoparticles by reducing the use of toxic chemicals, and additionally provides natural stabilizing, chelating, and capping agents. In the biological synthesis route, plant-mediated approaches are considered more as it reduces the extra time of identification, preparation of culture media, and isolation of microorganisms. Various biologically synthesized metal and metal oxide nanoparticles and their biomedical applications are summarized in Table 2.
Table 2 Different metal and metal oxide nanoparticles covered in this review, along with their biomedical applications
S. no. |
Source |
Metal and metal oxide |
Morphology |
Applications |
Ref. |
1 |
L-Glutathione |
Gold nanoclusters |
Size is 2 nm, with intense red fluorescence and high photostability |
Tumor targeted imaging, rapid imaging, fluorescence-imaging guided photothermal therapy of tumor |
67 |
2 |
Rose Bengal and dextran |
Gadolinium nanoparticles |
Paramagnetic behavior with 17 nm in size and spherical |
Bioimaging and tracking of cancer cells |
68 |
3 |
PLGA and glycol chitosan shell |
Iron oxide nanoparticles |
Superparamagnetic spherically shaped of 100–750 nm |
MRI contrasting agent |
69 |
4 |
Black beans (Phaseolus vulgaris) |
CuONPs |
Crystalline and uneven, spherical-shaped nanoparticles |
Inhibited the growth of cervical carcinoma cells by generating ROS |
70 |
5 |
Nepeta deflersiana |
AgNPs |
Spherical and 33 nm in size |
Inhibited the growth of cervical carcinoma cells |
71 |
6 |
Sargassum wightii |
MgONPs |
Spherical with 68.06 nm in size |
Cytotoxic activity against lung cancer cells |
72 |
Antimicrobial activity |
Photocatalytic activity |
7 |
Vaccinium arctostaphylos |
ZnONPs |
Spherical, 70–75 nm in size |
Antioxidant activity |
73 |
Anticancer activity |
Antibacterial activity |
8 |
Butea monosperma |
AgONPs |
Spherical with 35 nm |
Chemotherapeutic drug delivery |
74 |
9 |
Citrus paradise |
ZnONPs |
Spherical shaped 24.5 nm |
Anti-oxidant activity |
75 |
10 |
Moringa oleifera |
La2O3 NPs |
Spherical, 308 nm |
Antimicrobial, anti-oxidation activities and for drug delivery |
76 |
11 |
Bacterial cellulose |
TiO2 nanocomposites |
Dispersed nanoparticles of size between 20–30 nm |
Wound healing and antibacterial activity |
77 |
12 |
Artemisia annua |
ZnONPs |
Agglomerated, crystallized, spherical shaped, 20 nm in size |
Antimicrobial activity for effective mineralization and cytotoxic impact on MG-63 cells |
78 |
Additionally, plants are easily available, safe to handle, and possess various phytochemicals and different secondary metabolites, which act as an important material in synthesizing different metal and metal oxide nanoparticles by acting as reducing, capping, and stabilizing agents. Co-enzyme and co-factors act as desired, reducing the starting agent, and can also act as a precursor in the formation of the nanoparticle. On the other hand, enzymes and protein contents reduce the metal salts into their corresponding nanoparticles. It is proven that certain plants could naturally uptake and biologically reduce the metal ions into nanoparticles from soil that contain salts, minerals or ores by detoxification process and ultimately convert them into nanoparticles.11
The review highlights various biological approaches for synthesizing various metal and metal oxide nanoparticles, which are easy, cost-efficient, environment-friendly, and stable using various plant extracts as stabilizing, capping, and reducing agents. These biosynthesized metal and metal oxide nanoparticles have also established their profound uses in various biomedical applications to improve human health. Recently, the biological synthesis of nanoparticles using different plant extracts, fungi, and bacteria has greatly escalated as the synthesized nanoparticles from this method showed excellent polydispersity, dimensions, and stability. Nanoparticles can be utilized in therapeutics and diagnostics, optics, electronics, green energy, wastewater treatment and bioremediation. In this review, apart from the biological approaches for synthesizing metal and metal oxide nanoparticles, their potential applications in the biomedical field are also discussed. Nowadays, the biological synthesis of nanoparticles is more promoted over other physical and chemical methods due to rapid and fast synthesis, better control over size and shape, low toxicity, and cost- and eco-friendly approaches.8,11,79
3.1. Synthesis of nanoparticles using plant extracts
Different extracts of plant parts have been used to synthesize various nanoparticles that are mentioned in this review. The leaf extracts of chickpea have been used to synthesize AuNPs, in which the chickpea leaf extract acts as a reducing and capping agent.80 Furthermore, by using the Gnidia glauca flower extract, AuNPs can be synthesized.81 It was reported that the biosynthesis of both AuNPs and AgNPs could be achieved using the natural precursor clove (Syzygium aromaticum).82 Similarly, another method to synthesize bimetallic Au core–Ag shell nanoparticles is by utilizing Azadirachta indica (neem) leaf broth.83 Furthermore, the synthesis of gold nano-triangles and silver nanoparticles was completed using Aloe vera plant extract. It was also suggested that these gold nanotriangles could be used as various therapeutic agents.84 In another study, AuNPs synthesis was achieved by an aqueous extract of Mirabilis jalapa flowers,85 and AgNPs were synthesized via Cardiospermum helicacabum leaf extracts and Pulicaria glutinosa extract.86,87 Moreover, Annona squamosa was used to synthesize the copper oxide nanoparticle, which exhibited efficient antibacterial properties when tested against plant pathogenic bacteria. Furthermore, the experiment reported that the bio-synthesized CuONPs could be utilized for sensing H2O2, as it showed good electro-oxidation response towards H2O2.88 Crystalline and spherical-shaped selenium nanoparticles were synthesized using an aqueous Allium sativum extract that showed excellent pH stability, which found its potentialities in various biomedical utilities.89 Similarly, extracts of Asteriscus graveolens were also used to synthesize spherical-shaped selenium nanoparticles for targeted anticancer drug delivery.90 Furthermore, stable selenium nanoparticles were synthesized using Aloe vera extracts.91,92 Moreover, a comparative study was performed between aqueous Hordeum vulgare (monocotyledonous) extracts and Rumex acetosa (dicotyledonous) plants to synthesize iron oxide nanoparticles.93 An experiment reported the green-mediated synthesis of platinum nanoparticles using Diopyros kaki leaf extract.94 Furthermore, a systematic overview of the laboratory-based synthesis of various metal nanoparticles using plant extracts, fungi, bacteria, and algae is displayed in Fig. 3. These plant-based synthetic routes are more preferred as compared to the other chemical and physical routes as they are easy, laboratory-friendly, cost-effective, and the presence of various biological compounds makes them more suitable in the therapy of various diseases, like cardiovascular disease, neurodegenerative diseases, inflammatory diseases, wound healings, development of anti-microbial films and agents, among others.
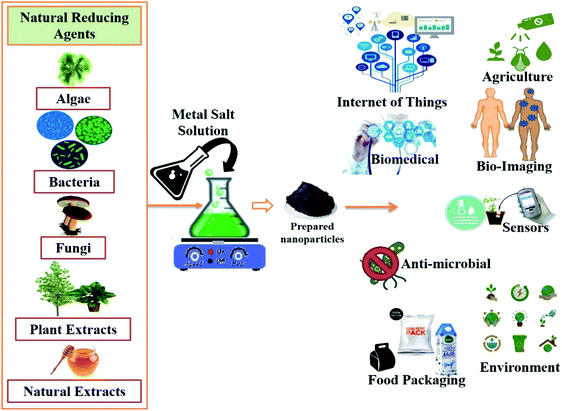 |
| Fig. 3 An illustration of the laboratory-based method to synthesize various metal and metal oxide nanoparticles using plant extracts, algae, fungi, bacteria, and natural extracts, along with the metal salt, which results in the production of the desired metal and metal oxide nanoparticles. Various applications of these biosynthesized metal and metal oxide nanoparticles are also shown in the figure. | |
3.2. Synthesis of nanoparticles using bacteria
The synthesis of nanoparticles using various bacterial species has also attracted the attention of researchers. For instance, it has been reported that by using Escherichia coli, AgNPs were biosynthesized and were found to be of a size of 50 nm. This process was found to be stable and cost-effective.95 Similarly, AgNPs were also synthesized by Bacillus thuringiensis, Corynebacterium strain SH09, Bacillus cereus, and extremophilic Ureibacillus thermosphaericus.96–99 Furthermore, Sintubin et al. reported that lactic acid bacteria acted as both reducing and capping agent to synthesize AgNPs.100 Cadmium sulfide nanocrystals were also reported to be biosynthesized using E. coli.101 Selenium-respiring bacteria, namely Sulfurospirillum barnesii, Selenihalanaero bactershriftii, and Bacillus selenitireducens, were used to synthesize uniform and stable nanospheres.102,103 Moreover, there are many metal and metal oxide nanoparticles that have been reported to be synthesized using different bacteria. Most of the bacteria-mediated syntheses were reported to be cost-effective, easy, and simple, and were also found to be non-toxic to the living cells, making them more suitable and efficient for use in therapeutic applications.
3.3. Synthesis of nanoparticles using fungi
The myogenic route for nanoparticle synthesis has been established for better nano factories over bacteria and plants from researchers worldwide, as they show better metal accumulation activity. Recent research on the few most commonly synthesizing nanoparticles using various fungi has been discussed. It has been reported that monodispersed AgNPs can be biosynthesized using Rhizopus stolonifer, and it showed good antibacterial activity, suggesting its utility as potential anti-bacterial agents.104 Similarly, in another study, it has been reported that AgNPs can be synthesized using Phoma glomerata. Furthermore, it was suggested that it acted as a better candidate for DDS, and exhibited antibacterial efficacy against resistant E. coli, P. aeruginosa, and S. aureus.105 However, several other fungi, like F. oxysporum, Fusarium solani, Pleurotussajorcaju, and Fusarium semitectum, can also be utilized for the biosynthesis of AgNPs.106–108 Several other studies have also reported the mycosynthesis of antimicrobial silver nanoparticles by the endophytic fungus Aspergillus clavatus, A. flavus, and A. flavus NJP08, respectively.96,109,110 Another study reported the biosynthesis of protein-capped AgNPs using fungus proteins of Coriolus versicolor, as the fungal proteins and glucose were responsible for the reduction. Moreover, these intracellularly synthesized AgNPs could be modified to exhibit both intracellular and extracellular AgNPs under alkaline conditions, whereby the surface S–H groups of the fungus played a major role.111 Similarly, Qian et al. reported the synthesis of AgNPs from an endophytic fungi Epicoccum nigrum isolated from the cambium of Phellodendron amurense.112 In another study, AgNPs were synthesized using the fungus F. oxysporum and reported to have antifungal activity against pathogenic yeasts.113 Various fungi have been reported to synthesize platinum nanoparticles using the fungi Neurospora crassa and F. oxysporum.114,115 Although fungi exhibit sensitivity towards selenium, as selenium nanoparticles show antifungal activity, certain fungi like Trichoderma viride, Chaetomium globosum, Aspergillus niger, and Pleurotus ostreatus can synthesize stable selenium nanoparticles.116 It was observed that these fungi-synthesized selenium nanoparticles exhibited enhanced biomedical activities. Similarly, Humicola sp. were used to synthesize cerium oxide nanoparticles.117
Various fungi are being utilized to synthesize gold nanoparticles, such as Penicillium sp., Geranium leaves and its endophytic fungus, edible mushroom Pleurotus florida, and the glucan content of mushroom.83,118–121 The enzyme-mediated biosynthesis of CdS nanoparticles was reported using the fungus F. oxysporum and S. cerevisae.122,123 CdTe quantum dots were synthesized using F. oxysporum, and also exhibited excellent antibacterial activity.124,125 Moreover, it was reported that the biosynthesis of cadmium crystal particles was achieved using the white-rot fungus C. versicolor.126 Several other metal and metal oxide nanoparticles were also synthesized via different fungi, like the nanosized magnetite from Mucor javanicus, F. oxysporum, and Verticellum sp.127,128 Similarly, A. alternate was used to synthesize selenium nanoparticles.116 F. oxysporum was used to synthesize strontium carbonate crystals,129 and this fungus was also used to synthesize titanium and silica nanoparticles.130 Jha et al. reported another approach to synthesize TiO2 nanoparticles by using S. cerevisiae, which exhibited efficient antibacterial activity against Gram-positive bacteria, making them a potential antibacterial agent for use in various biomedical purposes.131 Apart from these, various other biological routes, like using nutrient media (e.g., honey, egg, starch) and natural polymers (e.g., starch, pectin), are also utilized to synthesize various metal and metal oxide nanoparticles.
4. Applications
Effective attachment of the biological constituents in green synthesized materials enhances the therapeutic and diagnostic potential of metals and metal oxide nanoparticles. Fig. 4 shows the wide range of applications of biogenic metal and metal oxides nanoparticles in diagnostics and therapeutics. The following sections shall elaborate on the vast areas of biomedical sciences, where biogenic metal and metal oxide nanoparticles are of great concern.
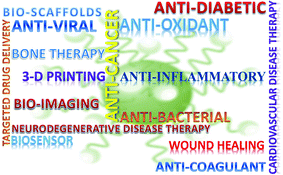 |
| Fig. 4 Schematic representation of the biomedical utility of bioinspired metal and metal oxide nanoparticles. | |
4.1. Diagnostics
There is an urgent need for accurate and precise analysis systems to understand mechanisms at the cellular and molecular levels, enabling the visualization and observation of cellular components, and therefore understanding their functions and alterations properly. Sensing various analytes and their metabolites shall be efficient using biogenic metal and metal oxide nanoparticles, which have characteristic SPR (surface plasmon resonance) with extreme sensitivity in the surrounding medium with brilliant sensing ability.
4.1.1. Bio-imaging. There are various types of nanoparticles, such as solid lipid nanoparticles, nanotubes, metallic nanoparticles, quantum dots, dendrimers, polymeric nanoparticles, and liposomes, used for biomedical imaging purposes. The diverse properties of nanoparticles, such as surface chemistry, magnetic, absorption, and emission properties, make them potential probes for detecting diseases.132 For example, red fluorescent Au nanoclusters were synthesized using L-glutathione, and were studied to detect cancer and non-cancer cells by combining them with porphyrin derivatives [tetrasodium pyrophosphate (TSPP)] for both in vivo and in vitro imaging purposes, as represented in Fig. 5. Moreover, these synthesized nanoclusters were so small in size that they could be easily excreted from the body, which eases the bio-imaging process by creating no toxic effects on the living cells. Therefore, researchers can explore this method to design new bio-imaging metal-based nanomaterials.67 Similarly, dextran-coated gadolinium oxide nanoparticles (Gd2O3 NPs) were synthesized using Bengal Rose extract, which exhibited excellent paramagnetic behavior, facilitating their utilization for bio-imaging and tracking purposes.68 In another experiment, biodegradable nanoparticles, i.e., hydrophobic poly(lactic-co-glycolic acid) [PLGA] core and a positively charged glycol chitosan shell, were used to fabricate the core–shell structure of a superparamagnetic iron oxide nanoparticle that was then utilized as an MRI contrasting agent.69 Similarly, PEGylated bismuth (PEG-Bi) nanoparticles were synthesized using methoxy[poly(ethylene glycol)]trimethoxy-silane (PEG-silane) and bismuth oxide (Bi2O3). The synthesized PEG-Bi nanoparticle showed excellent performance in X-ray computed tomography imaging and photothermal cancer therapy in vivo.133 Furthermore, in an experiment, the nanostructured bismuth oxide was utilized as a radiosensitizer and gadolinium nanoparticles as a contrasting agent for detecting glucose.134
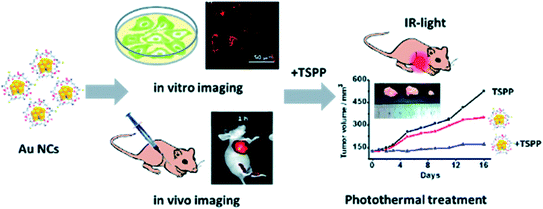 |
| Fig. 5 A schematic representation of Au nanoclusters combined with porphyrin derivative (TSPP) utilized for fluorescence-based bioimaging and photothermal treatment to detect cancer (reproduced with permission from Y. Zhang, J. Li, H. Jiang, C. Zhao and X. Wang, RSC Adv., 2016, 6, 63331–63337 (ref. 67)). | |
4.1.2. Biosensing. An analytical device used for analyzing different biological samples by converting their chemical response to an electrical signal is known as a biosensor. A biosensor comprises three components: a bio-element, the transducer, and an electronic unit. The bio-element constitutes bioreceptors, like enzymes, nucleic acids, amino acids, antibodies, and tissues. These bio-elements react with the biological sample and generate chemical signals, which are detected and converted into electrical signals by the transducers. The transducer efficiently detects the signals and converts them into electric signals, which are then further modified, processed, and displayed by the electronic unit. The different metal and metal oxide nanoparticles, like silver, copper, zinc, iron, cerium, manganese, titanium, zirconium, platinum, cobalt, gold, nickel, tungsten, and vanadium, have efficiently played a key role in the biosensing of chemical and biochemical analyses. For example, iron oxide nanoparticles were utilized to modify electrodes to detect glucose, H2O2, various heavy metals (like Pb, Zn and Cd); urea, nitrites and nitrates; dopamine and bisphenol-A. Furthermore, manganese oxide nanomaterials, namely MnO, MnO2, and Mn3O4, are the most researched and are found to be beneficial for bioelectrode materials in biosensing. However, the most extensively used metal oxide nanostructures considered for electrochemical sensing are copper(II) oxide (CuO) and copper(I) oxide (Cu2O). It was reported that cobalt oxide-doped copper oxide nanofibers were used as a sensing platform for the label-free detection of fructose.135 Similarly, it was also reported that CuO–Cu nanocomposites on graphene could be used for the detection of fructose,136 as the determination of fructose can be used for the early detection of various lifestyle diseases, like diabetes and digestive disorders. Furthermore, another sensor to detect glucose and fructose in blood serum was reported using the CuO/multi-walled carbon nanotube nanocomposite-modified glassy carbon electrode.137 A nanosensor was reported to detect glucose in drugs and human serum, which used immobilized glucose oxidase on an iron ferrite magnetic particle/chitosan composite-modified gold-coated glass electrode.138 Furthermore, it was reported that cholesterol oxidase, when co-immobilized with α-Fe2O3, showed a micro-pine-shaped hierarchical structures-based cholesterol biosensor.139 A hybrid material of chitosan (CS), fishbone-shaped Fe2O3 (f-Fe2O3), and electrochemically reduced graphene oxide (ErGO) was fabricated to act as a better sensing matrix for the electrochemical detection of gallic acid, which was further used to detect the effect of the antioxidant activity of the wine. The results suggested that CS-fFe2O3-ERGO/GCE can be efficiently used to develop electrochemical sensors.140It was proposed that the use of arc-discharging graphite rods containing copper wires to synthesize single-wall carbon nanotubes (SWCNTs) with nanocomposites of CuO would have good stability and linear glucose detection with higher sensitivity, quick response time and lower detection limit. Comparatively, the combination offered better performance in sensing, conductivity, and a higher response towards human serum samples.141 Another fabricated non-enzymatic electrochemical sensor for the simultaneous detection of glucose and fructose in hydrolyzed sucrose samples was proposed, which can also measure glucose in blood serum samples.137 Another study showed that the combined effect of copper nanoparticles and graphene sheets produced Cu-graphene sheets allowed for high selectivity, accuracy, and fast and stable amperometric sensing to detect glucose.142 The Cu-graphene sheets shall be an optimistic factor for the development of a non-enzymatic glucose sensor. Another study reported that the CuO2-like nano spindle combined with straight multi-walled carbon nanotubes-modified electrode sensed glucose with higher sensitivity and lower limit of detection.143 The metal oxide nanoparticle is considered a potential candidate for developing a new transducer capable of being utilized as an electrochemical biosensing platform.144 An electrochemical biosensor is an integral unit of biological elements, like an antibody, enzyme, proteins, and nucleic acid, with a transducer capable of efficiently sensing the element and transfer signal. Many studies have been conducted, which describe the potentialities of optical, electronic, and electrochemical properties of metal-oxide nanoparticles in suspended media and their surface activities at the nanobioelectrode for biosensing uses. The electron transfer rate highly relies on the type of reaction occurring between the biomolecules and metal-oxide nanoparticles, leading to an increased biosensing response signal.145 Moreover, these metal oxide nanoparticles can easily immobilize the targeted biomolecule on their surface, and are therefore considered more in electrochemical sensing. Fig. 6 (ref. 146) schematically describes the procedure of electrochemical biosensing by immobilizing the enzyme on the surface of a metal oxide nanofiber. Furthermore, the non-enzymatic biosensor with Cu(OH)2 dendritic structure fabricated from the boron-doped nanocrystalline diamond based on a Cu electrode for glucose detection reported better reproducibility, long-term stability, selectivity, and no interference from other oxidable species.147 The circular cobalt oxide nanorods were also found to be very efficient for easy non-enzymatic detection of glucose by amperometric method.148 Moreover, it was proved that the Ni nanoparticles-loaded TiO2 nanotube arrays (Ni-NPs/TiO2NTs) were first prepared by the anodization of the Ti foil, tracked by the electrodeposition method for better detection of glucose with a higher sensitivity, in addition to great analytical performance and simpler preparation method. This simple and effective method was helpful for the early detection of diabetes.149 Nano nickel oxide (NiO)-modified sensors with non-enzymatic sensing activity were also proposed for the assay of glucose with better electrochemical properties and electrocatalytic performance for glucose oxidation.150 Nowadays, many point-of-care devices have been developed, which eases the detection of various biomolecules (like glucose, fructose, and others), and can be used to develop effective therapeutic approaches for the treatment of various lifestyle diseases. Moreover, the onset of the internet of things (IoT) and wearable sensors have opened gates for researchers to develop and modify the traditional synthetic routes for the effective detection of biomolecules.
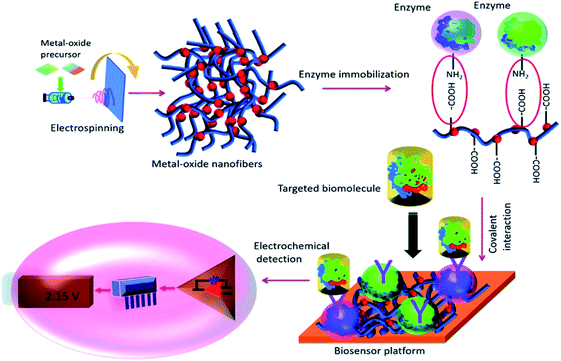 |
| Fig. 6 A schematic representation of the complete procedure of electrochemical biosensing utilizes the surface of the metal oxide nanofiber to immobilize the enzyme. This interaction between the enzyme and metal oxide nanofiber generates signals that are electrochemically detected and amplified (reproduced with permission from K. Mondal and A. Sharma, RSC Adv., 2016, 6, 94595–94616 (ref. 146)). | |
The minute amount of glucose was detected by an electrochemical biosensor made up of a ZnO nano tetrapod that utilized the multi-terminal ZnO nanostructure as a material adsorption.151 It was proposed that the electrodeposition of the chitosan gold nanocomposite (CGNC) and nickel hydroxide (Ni(OH)2) on a bare gold electrode with immobilized glucose oxidase and fabricated with electron transport for glucose-sensing would provide higher selectivity, sensitivity, and stability for better practical and clinical applications.152 Furthermore, it was ascertained that nanoflake ZnO, when immobilized with glucose oxidase, performed as a better potentiometric glucose biosensor.153 Similarly, it was mentioned that a novel enzymatic amperometric biosensor was formed when ZnO nanoparticles were electrodeposited on the glassy carbon electrode surface with multi-walled carbon nanotubes to immobilize the second layer of glucose oxidase with good stability and reproducibility.154 The nickel nanowire array and Pt-coated nickel nanowire array (Pt/NiNAE) can efficiently detect the glutamate electrochemically by enzyme-free sensing, and exhibit better electrocatalytic activity towards glutamate as compared to the pure Ni electrodes. This particular sensing promises a cost-efficient, enzyme-less, sensitive, accurate, selective, and stable sensor platform.155 Furthermore, the hemoglobin immobilized glassy carbon electrode was developed using the nanocomposites of Ag nanoparticles that were self-supported on the silver vanadium oxide (Ag2V4O11) nanobelts, and fabricated a critical sensitive biosensor for the detection of H2O2.156 Similarly, a modified glassy carbon electrode was developed using the reduced graphene oxide/Fe2O3 nanocomposite. This electrode was then used to prepare a very simple, novel, sensitive, and non-enzymatic electrochemical sensor to detect hydrogen peroxide that could efficiently display better sensing performance for the detection of H2O2 amperometric reduction.157 Furthermore, Wang et al. proved that hematite nanoparticles (α-Fe2O3) could be utilized for fabricating a non-enzymatic sensor that was applied in the catalytic reduction of H2O2, and thus results in the highly sensitive and selective reduction of H2O2. In another experiment, Wang et al. disclosed that Prussian blue-based (PB) nanocubes-nitrobenzene-reduced graphene oxide (rGO) nanocomposites (PB-nanocubes-nitrobenzene-rGO) detected H2O2 very promisingly.43
When novel graphene sheets were wrapped with CuO2 nanocubes, they exhibited good electrochemical stability, proving to be a good option for enzyme-free glucose and hydrogen peroxide sensors with good stability and sensitivity, selectivity, and faster amperometric response.158 It was observed that when photosynthesized silver nanoparticles were combined with fluorine-doped tin oxide in a conducting mode and were slightly modified with the help of zinc oxide nanorods, it worked well as an amperometric sensor for the detection of hydrogen peroxide.159 Furthermore, the synthesized γ-Fe2O3 nanoparticles by the one-pot method under hydrothermal conditions formed meso-tetrakis(4-carboxyphenyl)-porphyrin-functionalized γ-Fe2O3 nanoparticles, which were assumed to be a cheap, simple, convenient, selective, sensitive, accurate, and easy to handle colorimetric assay that could also catalyze the decomposition of H2O2 into H2O and O2.160 Another study by Low et al. demonstrated the strong interfacial bonding effect when bio-mineralized hydroxyl functionalized multi-walled carbon nanotubes were added onto calcium phosphate cement, which could be beneficial for fabricating electrodes for biosensing application with higher sensitivity and selectivity towards analytes of interest.161 To detect ricin, a silica-coated magnetic nanoparticle-based silver enhancement immunoassay was developed.162 Similarly, Tyagi et al. proposed a method to detect urea, in which a thin film NiO nanoparticle was deposited onto an indium tin oxide (ITO)-coated glass substrate that showed higher affinity and catalytic activity with redox behavior due to the great properties of NiO-NPs.163 Furthermore, by immobilizing uric acid, a potentiometric uric acid biosensor was developed, in which uricase was immobilized upon zinc oxide (ZnO) nanowires.164 It was observed that by conjugating biomolecules with colloidal nanoparticles of gold, the obtained signals from biosensors could be modified.165 The role of the ITO thin film modification methods and the immobilization of bio-recognition elements were studied. They demonstrated the utility of ITO for fabricating electrochemical metal and metal oxide nanomaterial-based ITO biosensors that were highly sensitive, conductive, and selective towards the analyte of interest.166 Owing to the distinguished properties of the metal and metal oxide nanoparticles, they have found profound use in both imaging and diagnostic applications. These metal and metal oxide nanoparticles are now considered as promising materials that can efficiently revolutionize the biomedical practices and researches.
4.2. Therapeutics
Nanostructures of biogenic metal and metal oxides can elicit tremendous therapeutic applications, such as anticancer, antidiabetic, anti-inflammatory, and antioxidant, which will be discussed in this section. Furthermore, the green synthesized nanoparticles have more demand due to their characteristics, such as bioavailability, cost-effectiveness, and environment-friendly nature.
4.2.1. Anti-cancer activity. Cancer is marked as one of the leading causes of death worldwide, and results in 8.2 million deaths per year. Furthermore, it has been estimated that the number of deaths due to cancer will increase each year, and it will reach about 13.2 million deaths per year by 2030. To date, nearly more than 200 different types of cancer are recognized, which exhibit six biological characteristics: replicative immortality, angiogenesis, invasion and metastasis, resistance to apoptosis, proliferative signaling, and evasion of growth.167 With the introduction of bionanotechnology, novel and innovative techniques have been developed to diagnose and treat different types of cancer. Furthermore, the fusion of nanotechnology with immunology has developed nano-immune-chemotherapy, which efficiently works in cancer treatment. Furthermore, cancer treatment effects are being restricted due to several factors, such as lack of bioavailability, drug resistance, and the non-specific toxic nature of chemotherapy. Thus, there is now a crucial demand for alternative strategies to combat cancer. Thus, various biogenic metal oxide nanoparticles have shown amazing results for cancer therapy by inducing cytotoxicity in cancerous cells, while not affecting the normal cells. Some biogenic metal and metal oxide nanoparticles that are widely used to treat cancer include black bean-synthesized copper oxide nanoparticles (CuONPs) and Nepeta deflersiana-synthesized AgNPs, which exhibit efficient anticancer activity when studied on HeLa cells by increasing the intracellular reactive oxygen species (ROS) in a concentration-dependent manner.70,71Moreover, MgONPs are known to exhibit biomedical properties as they show biocompatible nature, and are also highly stable under harsh conditions. They are mostly used for the relief of heartburns, inhibiting tumor-generating activities and others. Therefore, an experiment was performed that used aqueous extracts of brown seaweed Sargassum wightii to synthesize MgONPs, which exhibited good cytotoxic activity against lung cancer cell line A549 by increasing ROS generation, leading to apoptosis of the cancer cells. Sargassum wightii is a marine algae that is readily available. It is rich in polysaccharides, polyphenols, carotenoids, proteins, amino acids, vitamins, and minerals, which help them act as capping and reducing agents for the fabrication of metal and metal oxide nanoparticles. The synthesized MgONPs exhibited high zeta potential that enhanced their stability, and helped them show antimicrobial activity against both human pathogenic bacterial and fungal strains in a concentration-dependent manner. In addition, the synthesized MgONPs efficiently degraded the organic dye methylene blue under UV radiation and sunlight by showing photocatalytic activity.72
Furthermore, titanium oxide nanoparticles (TiO2) were synthesized using the bulb extract of Ledebouria revolute, which exhibited excellent anticancer activity against A549 cells. Ledebouria revolute was chosen as it is one of the medicinal plants, which is widely known to exhibit anticancer activity and antimicrobial properties, and also inhibits the larvicidal activity of Aedes aegypti.168 An advanced and cost-effective cancer therapy was developed using selenium nanoparticles. These selenium nanoparticles were synthesized using carboxylic acid, which efficiently generated cell apoptosis in the cancer cells.169 A comparative study was conducted to explore the anticancer potentiality of surface-modified selenium nanoparticles by different amino acids, mainly valine, lysine, and aspartic acid. This study showed that the aspartic acid and valine-decorated selenium nanoparticles exhibited less anticancer activity than the lysine-decorated selenium nanoparticles, suggesting that the lysine-decorated selenium nanoparticles can be used as a potential chemotherapeutic agent for combating cancer.170 Similarly, selenium nanoparticles exhibited good antitumor activity, and were functionalized by hyaluronic acid (HA-SeNPs). The HA-SeNPs efficiently controlled the immune-regulating properties and decreased the tumor mass.171–174 Moreover, a study reported that magnesium oxide nanoparticles combined with human serum albumin exhibited better plasma distribution and mediate apoptosis by ROS induced in the cell lines of cancer.175 Furthermore, a similar study on magnesium oxide (MgO) nanostructures showed excellent photocatalytic, antibacterial, and anticancer performance.176 Similarly, other metal and metal oxide nanoparticles (like gold, palladium, and zinc) synthesized via biogenic routes were utilized for obtaining better results in radiation oncology.177–179
4.2.2. Anti-diabetic activity. The production of insufficient insulin in the body does not allow the cells to reciprocate towards insulin, resulting in sugar accumulation in the blood, leading to a metabolic disorder known as diabetes. Therefore, diabetes can be both insulin-dependent and insulin-independent. Many enzymes interfere with this disease, upon which two of them are the key regulators: α-glucosidase and α-amylase. Here, we discuss some of the bio-synthesized nanoparticles of metal oxides that aid in treating diabetes by suppressing the secretion level of the enzymes. Ag NPs were biologically synthesized from Lonicera japonica, as the plant is known to consist of various medicinal properties, like antiviral, anti-inflammatory and anti-diabetic activities. The synthesized nanoparticle exhibited effective antidiabetic activity against carbohydrate digestive enzymes for diabetes, namely, α-amylase and α-glucosidase.180 Similarly, green synthesized silver nanoparticles utilizing the leaf extracts of Pouteria sapota inhibited the action on α-amylase, and the non-enzymatic glycosylation of hemoglobin confirmed the anti-diabetic activity.181Similarly, selenium nanoparticles are also known to cure diabetes, as they act as an anti-hypoglycemic agent by reducing oxidative damage and preventing hypoglycemic activity.182 Moreover, chitosan-stabilized selenium nanoparticles (Cs-SeNPs) exhibited anti-diabetic effects when observed in a rat model.183 It has also been observed that when selenium was combined with the cerium oxide nanoparticle, ROS levels were decreased with increasing insulin secretion, hence regulating the secretion of insulin and decreasing the oxidative stress.184–186 Similarly, ZnO NPs were synthesized through the microwave-assisted method with the help of Vaccinium arctostaphylos L. fruits extract that exhibited efficient anti-diabetic activities. They reduced the fasting blood glucose (FBS) levels and showed good control over the levels of some lipids in the treated diabetic rats. Moreover, the experiment reported that the green synthesized ZnO NPs exhibited better anti-diabetic properties than conventional synthesized ZnO NPs.73 Furthermore, there are many research studies that have been performed on the role of biogenic metal and metal oxide nanoparticles and their composites for anti-diabetic applications. However, there are very few commercialized products based on it, so there is a very urgent need for researchers working in this area to focus on developing commercial products based on biogenic metal and metal oxide nanoparticles and their composites for diabetes treatment. However, there are various plants that are known to possess anti-diabetic properties. Therefore, they can be considered for the preparation of the metal oxide nanoparticles to study their effective roles in combating diabetes.
4.2.3. Anti-inflammatory activity. Inflammation is the type of defense machinery shown by our body that responds to external stimuli, such as certain pathogens, allergens, irritants, and damaged cells. At times, this mechanism also lasts long after its beneficial activity, showing a prolonged effect. Metal-based nanoparticles exhibit excellent penetrating capacity in epithelial cells and inflammatory cells, which results in developing an effective and stable treatment for many diseases like cardiovascular diseases and gastric ulcers. Moreover, they exhibit better selectivity of target sites, such as inflammatory cells or tissues.187 There are many green synthesized metal and metal oxide nanoparticles that are known to exhibit anti-inflammation activity. The review discusses the anti-inflammation activity of various metal oxide nanostructures. Peptide-based Au NPs hybrid libraries have been used to inhibit Toll-like receptor (TLR) signaling, which was considered helpful in many acute and chronic human inflammatory diseases.188 The European cranberry bush is astringent and is consumed directly, and their fruit juice is the best-known product. It is a traditional drink in the Central Anatolia region (Turkey). The berries consist of high amounts of pectins, ascorbic acids, and flavanoids, which help them exhibit various medicinal properties. Moreover, they exhibit high antioxidant activity. Therefore, silver nanoparticles were synthesized from the European cranberry bush fruit (Viburnum opulus) extract that showed the effective release of IL-1α triggered by the irradiation of keratinocytes, and compared with the control and treated cells approving anti-inflammation activity.189 Enhanced anti-inflammatory activity was reported using AgNPs synthesized from the unripe fruit aqueous extract of Piper nigrum.190Moreover, it has been observed that the cerium oxide nanoparticle exhibits free radical scavenging activity, which makes them a suitable anti-inflammatory agent.191 Similarly, polysaccharide-modified selenium nanoparticles, along with inhibitory proteins like the Ik-B subunit, efficiently inhibited the phosphorylation of the JNK1/2, p38MAPK1 and NF-kB pathway (nuclear factor kappa B), which helped them show anti-inflammation activity.192 Moreover, gum-arabic stabilized selenium nanoparticles (GA-SeNPs) exhibited excellent anti-inflammatory activities, and thus prevented various inflammatory diseases.193 Many other properties of green synthesized selenium nanoparticles, like better anti-oxidant properties, also help them to exhibit anti-inflammation activity, making them suitable for treating cardiovascular diseases, wound treatment, and others. Other metals, like titanium oxide and zinc oxide nanoparticles, have also been reported to exhibit anti-inflammation properties in various mechanisms. For instance, the biofabricated zinc oxide nanoparticle shows anti-inflammatory activity by hindering the denaturation of albumin, inhibiting proteinase, and also possessing wound healing properties. Therefore, this suggests that green synthesized ZnONPs can be effectively used to treat wounds and can be used as a nano-ointment.194 Titanium oxide nanoparticles mostly exhibit anti-inflammation activity by decreasing platelets or increasing the thrombin-anti-thrombin levels. This is achieved by creating oxidative stress in the macrophages and increasing the TAT levels by suppressing the PAR pathways (Protease-Activated Receptors), respectively, which are responsible for generating inflammation in the body.195 Furthermore, many other metal and metal oxide nanoparticles synthesized via the biogenic way play an important role in the biomedical application and exhibit great anti-inflammatory activity. Thus, the researchers working toward this must focus more on finding the mode of mechanism of the biogenic metal and metal oxide nanoparticles for their great anti-inflammatory activity.
4.2.4. Antioxidant activity. Oxidative stress is generated inside the cell when an imbalance between the reactive oxygen species and excess nitrogen production occurs. Therefore, nanoparticles play an immense role in the biomedical domain, as they exhibit commendable antioxidant properties by hunting and killing free radicals. One of the highly researched nanoparticles is the cerium oxide nanoparticles, as they exist in two oxidation states. It was observed that when brain tissues of rats were exposed to cerium oxide nanoparticles, they increased the thiol content and started the caspase-3 activity, which proved that cerium oxide nanoparticles exhibited incredible antioxidant property.196 Similarly, cerium oxide nanoparticles were coated with levan polysaccharide. They exhibited mutual anti-oxidant activity when observed against H2O2 in the NIH3T3 cells as levan. Its derivatives are known to exhibit antioxidant, anti-tumor, and anti-inflammatory activities, which when combined with cerium oxide nanoparticles, provides them water solubility and stability. These properties of the green synthesized cerium oxide nanoparticle help them be used for various therapeutic applications.197Due to their small size and large surface-to-volume ratio, nanoparticles can act as synthetic antioxidants in the body that are suitable for several therapeutic applications. Here are a few examples showing nanoparticles as potential antioxidants in the body that would treat diseases caused by reactive oxygen species (ROS), disturbing the normal redox balance. Various experiments that have synthesized zinc oxide nanoparticles from different plant extracts, like Citrus paradise and Cassia fistula, have demonstrated excellent antioxidant activity of zinc oxide nanoparticles by 2,2-diphenyl-1-picrylhydrazyl (DPPH) radical scavenging.75,198 Therefore, it can be concluded that the citric acid-containing plants act as a better anti-oxidizing agent. Research can thus be conducted to synthesize various metal and metal oxide nanoparticles using citric acid plants that can be utilized as anti-oxidizing agents. Similar findings were reported in a comparative study of zinc oxide nanoparticles with zinc sulphate (ZnSO4), where zinc oxide nanoparticles were synthesized from the Lavandula vera leaf extract. This experiment showed that the green synthesized zinc oxide nanoparticle exhibited better antioxidant activities than zinc sulphate by increased DPPH radical scavenging activity.199 The antioxidant activity is required to prevent the damage caused by the free radicals. Like other metal and metal oxide nanoparticles, the green-synthesized copper oxide nanoparticles from the flower extract of Matricaria chamomilla, an aqueous root extract of Desmodium gangeticum and Tinospora cordifolia, have been reported to exhibit excellent antioxidant activity.11,200–202 Similarly, zinc oxide nanoparticles synthesized from the leaf extract of Mangifera indica and pulp of Vitis rotundifolia (hybrid grapes) exhibited good antioxidant activity due to the high concentration of phenolic groups in both plants, and their major plant parts showed anti-oxidant activity.203–205 The leaf extract of Moringa oleifera was used to synthesize lanthanum oxide nanoparticles (La2O3 NPs), which exhibited excellent antioxidant activity. Moringa oleifera is known to exhibit antioxidant activity and is also considered a medicinal plant. However, the synthesized La2O3 NPs from M. oleifera exhibited even better anti-oxidation activity, as they easily transported the free electrons to the free radicals of N2 present in DPPH.76,206
4.2.5. Anti-bacterial activity. Numerous antibiotics are being discovered for the treatment of microbial infection. However, the problem is with increasing antibiotic resistance and toxicity, due to which the reliability is limited. On the other hand, nanostructures of metal and metal oxides confer more potentiality to treat antibacterial infections than antibiotics. For example, the nanoceria-doped composite nanofibers show toxicity against Gram-positive and Gram-negative bacterial strains, and can be used in antibacterial treatment.27,207 Furthermore, Singh et al. reported various biosynthesis methods for cerium oxide nanoparticles and their effects on the living tissues, properties, and potentialities in diagnostic and therapeutic fields, highlighting their prospects and future outlook.28Furthermore, the fruit extract of Emblica officinalis was utilized to synthesize MgONPs. When the cotton fabric was treated with MgONPs, it showed strong antibacterial activity and could be used in medicine.48 Another method was reported using a bacterial strain to synthesize MgONPs that exhibited good antibacterial, anti-arthritic, and anticancer activity.208 Silver nanoparticles were synthesized from the culture supernatant of the endophytic fungus (Raphanus sativus), which proved to have a better antibacterial effect on Gram-negative and Gram-positive bacteria pathogens.79 The silver nanoparticles were synthesized using Aloe vera, Portulaca oleracea, Solanum nigrum, and Cynodon dactylon, and were used as bactericidal agents against human pathogens.209 To increase the antibacterial efficiency towards Gram-negative bacteria, selenium nanoparticles were stabilized with the help of the spider silk protein eADF4(k16), which provided the selenium nanoparticles with a positive charge on their surface.210 The aqueous leaf extract of Canthium dicoccum was used to synthesize zinc oxide nanoparticles, which exhibited enhanced antibacterial activity against Bacillus subtilis and least towards Staphylococcus aureus.211 Platinum nanoparticles exhibited antibacterial properties against various bacteria, namely Escherichia coli, Staphylococcus aureus, Salmonella typhi, and Artemia salina nauplii.212 Recently, it has been reported that to enhance the biological properties of the metal and metal oxide nanoparticles, they should be combined, capped, stabilized or doped with other elements like polysaccharides and metals. Hence, it was reported that when iron was doped with copper oxide nanoparticles, they exhibited enhanced antibacterial properties by inhibiting both bacterial colonies and biofilm formation.213 Similarly, chitosan-based copper nanoparticles exhibited efficient antibacterial activity against Vibrio parahaemolyticus.214 Furthermore, biogenic metal and metal oxide nanoparticles exhibited antibacterial efficiency by various methods like damaging the plasma membrane (which leads to the bursting of the bacterial cells), the generation of excess ROS in the bacterial cell (which will lead to the increase in oxidative stress inside the cell), or the slow release of the drug (which will target the organelle), as described in Fig. 7.
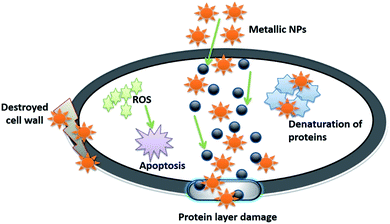 |
| Fig. 7 Illustration of the mode of mechanism followed by metal and metal oxides to exhibit antibacterial activity against bacteria, like denaturing the protein, generating ROS, increasing oxidative stress inside the cell, and damaging the cell wall. | |
4.2.6. Anticoagulant activity. Coagulation is when the blood turns from a liquid to a gel-like substance to form clots that stop bleeding at the injury site. Unfortunately, at times, this mechanism of protection can turn into a harmful event that causes harm to the body. Several diseases like allergies, injuries, and cardiovascular diseases require anticoagulation processes, as it turns the coagulation to malfunctioned state. In addition, increasing cardiovascular and cerebrovascular complications arise due to the formation of unusual clots in the blood vessels, leading to a phenomenon known as thrombosis.215 A cell-free extract of Bacillus safensis LAU 13 was used to synthesize biogenic silver nanoparticles that acted as an efficient blood coagulant and thrombolytic agent.216 Furthermore, the silver and gold nanoparticles synthesized from the biomass of the bacterium Brevibacterium casei demonstrated efficient anticoagulant activity.217An experiment was performed to study the time and concentration-dependent coagulation activity in the presence of cobalt oxide nanoparticles (Co3O4NPs) biologically synthesized from red algae that demonstrated great anti-coagulation activity. However, the experiment did not clearly explain the mechanism of the coagulation process, which needs to be determined by the researchers working in this area of research.218 Similarly, face-centered and crystalline nickel oxide nanoparticles were synthesized from the leaf extracts of Euphorbia heterophylla (Linn.), which also showed efficient anticoagulant activity.219 Cola nitida (Sterculiaceae) extracts were also used to synthesize titanium oxide nanoparticles (TiO2 NPs) that exhibited excellent anti-coagulant activity in vitro when observed on human blood. In addition, it was observed that these bioinspired titanium oxide nanoparticles maintained the morphology of red blood cells.220 Apart from the plants showing anti-coagulation activities, several other organisms (like Hirudinea) exhibit excellent anti-coagulation properties, and therefore can be utilized to synthesize various metal and metal oxide nanoparticles to exhibit anti-coagulation activity.
4.2.7. Drug delivery. The small size and large surface area of the nanoparticles help them exhibit advanced colloidal stability and bioavailability. These features help them cross the blood–brain barrier, pulmonary system and become absorbed through the endothelial cells. Specifically, metal and metal oxide nanoparticles possess some benefits like high stability, easy synthesis processes, easy fabrication to the desired size, shape and porosity, less swelling variations, advanced incorporation into hydrophobic and hydrophilic systems, and easy functionalization by various molecules due to the negative charge of the surface, which make them a promising tool for biomedical applications. Moreover, the metal and metal oxide nanoparticles react with in vivo systems differently depending on their size, shape, purity, stability, and surface properties. Therefore, it makes it necessary to characterize their morphology.221 Tumor targeting, photothermal therapy, imaging, and drug delivery can be achieved using fluorescent quantum dots, carbon nanotubes, metal and metal-oxide nanoparticles, and ceramic nanoparticles.222 The researchers have currently started focusing on the targeted DDS, which is now considered an important biomedical application as it targets to deliver various kinds of drugs to the specific sites of the body, and therefore avoids damage to nearby healthy cells. A brief overview of the targeted DDS using metal oxide nanoparticles loaded with the drug has been described in Fig. 8. Furthermore, the mode of mechanism for targeted DDS of the metal or metal oxide nanoparticles is that the desired drug is encapsulated or conjugated with the metallic nanoparticles. The metallic nanoparticle exhibits magnetic properties that help guide them through the external magnetic field to the specific site. After reaching the specific site, the drug is then released either through enzyme activity, or change in temperature or pH.223 The therapeutic metallic nanoparticles of iron oxide, silver, gold, and gadolinium nanoparticles were used for glioblastoma treatment.224 Similarly, the bismuth nanoparticle was used to induce autophagy and endocytic mechanisms in human kidney cells.225 The DDS using silver nanoparticle was synthesized from a plant extract of Butea monosperma loaded with doxorubicin. It showed great anti-cancer potentialities with targeted on-site drug delivery, and this is now an FDA-approved chemotherapeutic drug.74 The silver nanoparticles synthesized from Delftia sp. strain KCM-006 culture supernatant using antifungal drug miconazole effectually inhibited ergosterol biosynthesis and biofilm fungus formation, and can be used to develop anti-fungal medicines.226 The metal and metal oxide nanoparticles have eased the delivery of these drugs to the targeted cells, easing cancer treatment and eliminating the risk of generating side effects of chemotherapeutic drugs. Furthermore, the green synthesis approaches have made the development of metal and metal oxide nanoparticles easily cost-effective, and have filled the gap between the biomedical domain and nanotechnology.
 |
| Fig. 8 Systematic mode of the mechanism of metal oxide nanoparticles loaded with drugs for delivering at the target cell by denaturing the cell's surface proteins. | |
A study conducted to treat childhood neuroblastoma with dextran-coated cerium oxide nanoparticles loaded with curcumin was utilized. It was observed that these dextran-coated cerium oxide nanoparticle-loaded curcumin efficiently induced cellular toxicity in the neuroblastoma cells and did not affect the normal cells.227 Moreover, cerium oxide nanoparticles exhibit redox properties that help them be considered an efficient drug-delivery agent.228 Similarly, selenium nanoparticles are also considered a suitable agent to deliver drugs as they have efficiently delivered various inorganic cancer therapeutic drugs, like propylene oxide-modified ruthenium complexes and ethylene oxide copolymer.229 Furthermore, transferrin-conjugated selenium nanoparticles efficiently delivered doxorubicin-cisplatin into the mammalian breast cancer cell line (MCF-7), leading to apoptosis in cancer cells.230 It has been observed that cancer cells show a more acidic nature at high-temperature than normal tissues and blood. Thus, it is important to consider pH while developing a cancer-related DDS. For the same, ZnO–quercetin nanocomposite was developed to deliver quercetin in the cancer cells at two different pH values, 5.5 and 7.4, because pH plays a crucial role during the delivery of the quercetin in cancer cells. The results of this experiment showed that the quercetin was found to be stable at pH 7.4 as compared to pH 5.5. This is because at pH 7.4, the hard ligands (–OH groups) present in quercetin survived in the ionized form (O−) and acted as an active ligand during the chelate formation. In contrast, at pH 5.5, these hard ligands existed in an unionized form, which led to the instability of the quercetin. Furthermore, it was noted that the controlled and slow release of quercetin at pH 7.4 could maintain the ZnO–quercetin nanocomposite in the bloodstream for a certain time without causing any side effects.231
4.2.8. Neuroregenerative therapy. The maintenance of neuronal activity plays a major role in neurodegenerative diseases, leading to new restorative and neuroprotective treatments in Parkinson's disease.232,233 Furthermore, the biologically synthesized nanoparticles of several metal and metal oxides impart tremendous applications for treating various neurodegenerative diseases. Biosynthesized spherical silver nanoparticles were also studied with differentiated human neuroblastoma cells. The result of this study indicated extraordinary neurodegenerative activity.234 Moreover, it has been observed that treatment with selenium can efficiently reduce the risk of generating neurodegenerative diseases when observed in different animal models.235–237 Furthermore, both elemental and modified selenium nanoparticles efficiently exhibit antioxidant activity on neurons and the brain, as they are present as a cofactor in glutathione peroxidase (GPx), which helps reduce H2O2 and prevents oxidative damage.28 Furthermore, it was observed that patients affected with diseases such as Huntington's disease and Alzheimer's disease exhibited selenium deficiency in their body, resulting in the loss of neuron cells and brain dysfunction.238,239 Therefore, early detection of selenium in the body can help develop therapeutic approaches to treat various neurodegenerative diseases.The major cause of the generation of neurodegenerative diseases, such as Parkinson's, Huntington, and Ischemic Strokes, is due to a rise in oxidative stress and production of free radicals.173 The distinctive pharmacological and biological properties of cerium have helped them treat various diseases for more than a century.240 It has been observed that cerium oxide nanoparticles are considered as an excellent antioxidant agent because they exhibit excellent neutron shielding effects by restricting free radical generation and affect the signal transduction pathways, which can be used as a therapeutic agent to treat neurodegenerative diseases.241–244 Another common cause of death globally is ischemic stroke or cerebral stroke, in which lack of blood flow is caused due to the formation of hemorrhage or clots in the brain. It was observed that cerium oxide nanoparticles, when enfolded with phospholipid-polyethylene glycol, helped in protecting from ischemic stroke.245 However, not much data is available on the green synthesized metal and metal oxide nanoparticles, which can treat neurodegenerative diseases. Thus, there is plenty of room for researchers working on therapeutic aspects to develop bioinspired metal and metal oxide nanoparticles to treat neurodegenerative diseases efficiently.
4.2.9. Cardiovascular diseases. Vascular ailments, structural abnormalities, and blood clots are known as cardiovascular diseases, for example, coronary heart diseases, atherosclerosis, angina pectoris, and myocardial infarction. It was reported that acute myocardial infarction could be diagnosed using the biomarker myoglobin quantified by electrochemical nanobiosensors, which direct electron transfer between Fe(III)-heme and electrode modified by gold nanoparticles/didodecyldimethylammonium bromide (DDAB/Au)-antibody (anti-myoglobin).246Furthermore, the AuNPs can inhibit vascular endothelial growth factor-stimulated angiogenesis in vivo and in vitro.247 The Ag NPs synthesized using Bacillus licheniformis efficiently inhibit IL-1β molecules and vascular endothelial growth factor (VEGF) in porcine retinal endothelial cells and reduce vascular permeability, thus showing better anti-angiogenic properties.248 Furthermore, the AgNPs synthesized from plant extract Salvia officinalis reduce hemoglobin content in chick chorioallantoic membrane blood, proving to have good antiangiogenic properties.249 The decreased VEGF-mediated cell proliferation, tube formation and migration are inhibited by the P13K/Akt signaling pathway utilizing Ag NPs synthesized in bovine retinal endothelial cells.95 Furthermore, many biogenic metals and metal oxide nanoparticles can be used to treat cardiovascular diseases due to their extraordinary properties. Thus, researchers working on cardiovascular disease treatment aspects can validate the utility of bioinspired metal and metal oxide nanoparticles to treat this deadly disease. They must also work on determining the mode of mechanism data of these nanoparticles for curing this disease.
4.2.10. Bone-related and dental therapeutics. The application of natural and synthetic polymer nanocomposites in bone tissue regeneration has been reported using nano zirconia and silver. It has been reported that CeO2-incorporated mesoporous calcium-silicate (CeO2-MCS) stimulates the proliferation and alkaline phosphatase activity of osteoblast cells. Therefore, CeO2-MCS materials with drug delivery would be appropriate for bone regeneration.250 Osteoporosis has gained much attention from researchers as it has become a major medical concern. Until now, no effective treatment has been reported. Osteoporosis is a musculoskeletal ailment marked by decreased bone mass or low bone mineral density (BMD) in the body, and majorly affects women over the age of 60 or women experiencing menopause. There is now an urgent requirement to develop effective and permanent medical treatments for osteoporosis. Therefore, ZnO NPs for the treatment of osteoporosis have been considered as they find their immense potentialities in the biomedical domain. Artemisia annua was selected as it is known for its traditional medicinal activities like anti-hyperlipidemic, anti-plasmodial, anti-convulsant, anti-inflammatory, anti-microbial, and anti-cholesterolemic activities. Furthermore, its biochemical analysis shows that it consists of 123 phytoconstituents in various parts (leaves, stem, and roots), such as terpenoids, flavonoids, caffeoylquinic acids, coumarins, acetylenes and sterols. These synthesized ZnO NPs were found to be stable and effectively exhibited stimulatory effects on the differentiation in MG-63 cells in terms of the viability of the cell, alkaline phosphatase (ALP) expression activity, collagenogenesis, and mineralization, which indicates that A. annua-synthesized ZnO-NPs could induce osteoblast differentiation in MG-63 cells.78 However, there is scant scientific research related to the utility of biogenic metal and metal oxide nanoparticles for solving bone-related complications. Hence, researchers working in this field should explore more potentialities of these biogenic nanoparticles for bone-related disease.Nowadays, the dental sector utilizes nanoparticles to a vast scale as it is biologically reliable when it comes to nanoparticles obtained via green synthesis. When a dental implant is substituted by biocompatible hydroxyapatite and titanium embedded into the alveolar bone and artificial tooth instead of using ceramic materials, it promises safety. New implants created using alumina/zirconia nanocomposites exhibited better efficacy than ceramic materials.251 Furthermore, it was observed that zirconia oxide nanoparticles showed good anti-biofilm activity against bacteria like Enterococcus faecalis, and simultaneously acted as polishing agents in dental practices.252 Many other metal and metal oxides synthesized via the biogenic route can also solve dental problems, but they need to be researched further. A few of the potential metal and metal oxide nanoparticles that can be used in dental complications are silver, copper, cerium, zinc, magnesium, and vanadium, owing to their extraordinary antimicrobial properties. However, before utilization, the researchers or scientists working on this aspect have to explore their cytotoxicity and mode of mechanism data.
4.2.11. Wound healing activity. Hydrogel Ag NPs synthesized from the Arnebia nobilis root extract act as a beneficial wound healing agent, eco-friendly, and lack side effects.253 Similarly, glucuronoxylan-mediated Ag NPs synthesized from the seeds of Mimosa pudica exhibited vast potential in antibacterial activity against a range of Gram-positive and Gram-negative bacteria, and demonstrated remarkable wound healing properties in rabbits.254 Recent studies have shown that the in vivo use of microporous Ag/ZnO NPs, when loaded with chitosan, greatly accelerates the wound healing process at the starting state when observed in mice.255 Bacterial cellulose (BC-temporary skin substitute)-loaded TiO2 nanocomposites showed excellent wound healing progress when observed in the mice model. This BC-TiO2 nanocomposite treatment efficiently promoted the healing process via fibroblast migration and increasing growth of the epithelial cells in the blood supply, and it also formed new blood vessels.77 Apart from the above-mentioned biogenic metal and metal oxide nanoparticles, there are many others that can also be very efficient for wound healing activity, but they need to be explored before utility.
5. Anti-viral activity of various metal and metal oxide nanoparticles
Viral infections are considered one of the major root causes of mortality worldwide, which have led to significant losses in social, economic, and human lives over the years.256 Until now, the occurrence of sudden viral outbreaks has not been tracked, and can be settled down to the limited detection techniques and the rapid adaptive nature of viruses consisting of pretty big genomes.257 Therefore, it has become necessary to develop rapid, sensitive and specific diagnostic tools to detect viral strains and control their mass spreading.258 Many literature-based reviews have also started highlighting various impacts of SARS-CoV-2, and many types of research are dedicated to developing vaccines and sensors to combat this pandemic quickly.259 The spread of viruses generally takes place either through direct contact or via aqueous environment. However, with the sudden outbreak of the novel coronavirus (CoV), the researchers have now started focusing on finding new ways and methods for the early detection of viruses and their related treatments. SARS-CoV-2 also belongs to the RNA virus family. It is known to cause several diseases in mammals and birds, and is spread through direct contact with patient.260 The current strain of coronavirus is known as COVID-19 or SARS-CoV-2. It is closely related to Severe Acute Respiratory Syndrome (SARS), which appeared in 2002 and 2003, infecting ∼8000 people. COVID-19 affects differently than other COVID strains and is now considered a pandemic, affecting millions of patients worldwide in just a year.261
Currently, nanotechnology is dominating various domains in the science and technology fields, and has also found its profound use during the outbreak of the SARS-CoV-2 pandemic. Nanotheranostics is considered an innovative fusion of diagnostic and therapeutic functions combined in a unit multifunctional nanoplatform.262,263 Similarly, nanoparticles are also considered promising theranostic tools used in drug delivery, diagnostics, and the development of vaccines. Moreover, they have been approached to visualize and track the mechanism and treatment of various diseases, particularly viral infection.264 In order to develop a propitious and precise “theranostics based nano-platform”, three major factors should be precisely selected, including the correct therapeutic agent, the nanoparticles that will act as carriers, and the imaging agent.265 Therefore, several biogenic metals and metal oxide nanoparticles exhibit anti-viral activity and virus diagnosis potentiality.266–268 The modifications of the nanoparticles enhance their biological, chemical, surface, physical, and optical properties, making them more suitable for various domains. Moreover, it was observed that when lanthanide nanoparticles were doped with polystyrene, it efficiently detected anti-SARS-CoV-2 Immunoglobulin G (IgG) from human serum, therefore making a rapid sensitive and accurate nano-based diagnosis of SARS-CoV-2.269 Many companies like MIT-spinout startup and World Nano Foundation (WNF) are designing an antibody-based COVID-19 kit consisting of gold nanoparticle-based strips that can rapidly detect Immunoglobulin M (IgM) and IgG. The main idea behind this kit is to detect SARS-CoV-2 directly from blood or urine samples by the color change, as these strips will be covered with antibodies conjugated with gold nanoparticles that will interact with the viral antigens (SARS-CoV-2) present in the sample. The interaction between the antigen and antibody will lead to the change in color being visible within 5 minutes, as represented in Fig. 9.258,270,271 These gold nanoparticle-based strips are commercially used in the lateral flow assay with a clinical sensitivity of 57 and 81%, 100% specificity, and 69 and 86% accuracy for IgG and IgM, respectively.271 Although the major limitation possessed by this test is that it does not specify a particular strain of virus; rather, it only detects the presence of infection. In order to subdue this limitation, researchers from MIT have designed a screening device that can easily distinguish between the two similar gene sequences so that SARS-CoV-2 can be accurately detected.272 The biogenic copper nanoparticle exhibits good anti-oxidation properties, making them a potential antiviral agent against SARS-CoV-2.273,274 The iron oxide nanoparticles exhibited efficient antiviral activity, and were examined on both SARS-CoV-2 and Hepatitis-C virus (HCV) via molecular docking studies. The results showed that the iron oxide nanoparticle efficiently interacted with the spike protein receptor-binding domain (S1-RBD) of SARS-CoV-2 and glycoproteins: E1 and E2 of HCV. The stable complex was formed (Fe3O4 with S1-RBD of SARS-CoV-19), whereas Fe2O3 formed a stable complex with glycoprotein E1 and E2 of HCV. These visible conformational modifications in the structural protein can finally lead to the death of the virus. Therefore, iron oxide nanoparticles can find their profound use in combating SARS-CoV-2, as they could be used to produce antimicrobial fabrics like lab coats, masks, gloves, bedsheets, pillow covers, and oversheets. These antimicrobial fabrics can safely be used in hospitals to control the spread of viral and nosocomial infections in hospitals.275 Zinc-oxide nanoparticles (ZnO NPs) have previously been studied for their potential antimicrobial role. Another study showed their potential role in antiviral activity against SARS-CoV-2 by inducing oxidative stress in the cellular membranes of SARS-CoV-2, and these ZnO NPs were utilized as a potential disinfectant spray. ZnO NPs can produce cytotoxicity. Therefore, modifying them with polyethylene glycol can enhance their antiviral activity, and at the same time, decrease their cytotoxicity towards normal cells. It has also been suggested that ZnO NP can greatly enhance the immune response against the virus, which can lead to potential therapeutic properties that will help fight against SARS-CoV-2.261 Furthermore, there are many other potential biogenic metal and metal oxide nanoparticles, like selenium, cerium, magnesium, vanadium, lanthanum, copper, iron, and metal–metal nanocomposites that demonstrate unique antimicrobial potential. However, their utility towards combating SARS-CoV-2 must be validated by material scientists working towards fulfilling biomedical applications, as it is the need of the hour for working to achieve this objective due to the devastating conditions caused by the SARS-CoV-2 pandemic.
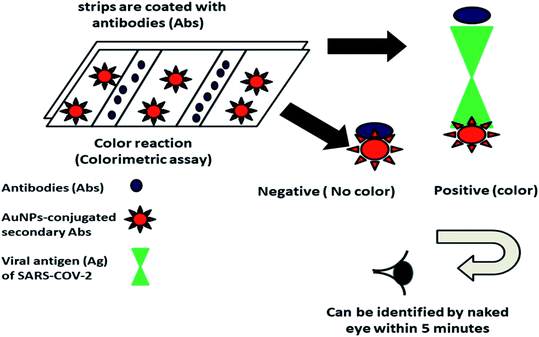 |
| Fig. 9 Schematic illustration showing the colorimetric detection of SARS on the AuNP-based strips. Strips are coated with antibodies (Abs) that hold the capacity to actively bind with viral antigens (Ag) present on SARS-COV-2 and can subsequently form a conjugate with AuNPs-conjugated secondary Abs. This conjugation will finally result in a color change, indicating the positivity of the tested sample (reproduced with permission from G. Ibrahim Fouad, Bull. Natl. Res. Cent., 2021, 45, 36 [CC BY 4.0] (ref. 258)). | |
6. Conclusion and prospects
Today, many types of nanoparticles are researched, like dendrimers, metallic nanoparticles, and liposomes, and this review attempts to explore the various biomedical applications explicated by the biogenic metal and metal oxide nanoparticles. Furthermore, the biogenic nanoparticles have evolved with time. Even today, they are advancing at a faster pace in various important aspects of clinical fields, such as diagnosing and treating cancer and neurodegenerative diseases, antibacterial and antioxidant activities, and biomolecular detection of analytes. Furthermore, modifying these biogenic metal and metal oxide nanoparticles will contribute a lot towards the eternal scope of research due to their magnificent combination of chemical and mechanical properties. A better understanding of the properties and modulations in their morphology shall pave the way to explore the nanoparticles' potential to cross the hurdles of many biomedical fields, like cytotoxicity and bioavailability.
The synthesis approach of nanoparticles plays a crucial role in defining their properties and utilities in different domains. Therefore, nanoparticle synthesis plays a vital role in the development of nanotechnology. Furthermore, biogenic/bioinspired synthesis has captured much attention nowadays, as they are eco-friendly, cost-effective, and easy to use. This synthesis method utilizes natural products (e.g., plant extracts, microbes), which help eliminate the excessive use of chemicals and open new avenues for the utility of metallic nanoparticles. Moreover, the biological approaches to synthesize nanoparticles mainly consist of plants, algae, yeasts, fungi, and bacteria, but the plants are preferred over the other biological routes, as they are easy to find and no culture media maintenance are required. In the plant-based synthesis of nanoparticles, various phytochemicals of plants act as stabilizing, caping, and reducing agents, which replace chemical-based stabilizers and reducers. Furthermore, the biologically synthesized nanostructures are considered more for research, as they confer very little or lack any chances of toxicity. Due to this property, these bioinspired nanoparticles play a vital part in the diagnostics and therapeutics of certain life-threatening disorders. These bioinspired metal and metal oxide nanoparticle properties have helped them gain much attention during the current pandemic, as they are not only used in detecting the coronavirus, but are also being used to produce disinfectant sprays and the formation of antimicrobial films. Furthermore, the bioinspired metal and metal oxides have found their profound use in producing safety equipment, kits and suits.
This review is one of a kind, as it mainly focuses on the biologically derived metallic nanoparticles and their utilities in both diagnostic and therapeutic applications. It will also help in gaining knowledge about different kinds of biogenic metal and metal oxide nanoparticles. The current pandemic calls for advancement in public health research, biotechnology, nanotechnology, and environmental technology. Thus, the review enhances the knowledge in biomedical fields and the use of nanotechnology in developing and enhancing this field. It will also shed light on the various uses of bioinspired metal and metal oxide nanoparticles during pandemic times by discussing their biomedical utility. Hence, this review will, in detail, provide an up-to-date literature review of biogenic metal and metal oxide nanoparticles for biomedical (diagnostics and therapeutics) applications, along with their properties, biosynthesis and role during the SARS-CoV-2 pandemic.
Funding
This review did not receive any specific grant from any funding agencies in the public, commercial, or not-for-profit sectors.
Author contributions
K. R. B. S. contributed to the conceptualization, data curation, investigation, resources, validation, visualization, and writing an original draft. V. N. contributed to the data curation, visualization, and writing an original draft. A. K. S. and J. S. contributed to the supervision and reviewing, & editing the manuscript draft. R. P. S. contributed to the conceptualization, validation, project administration, supervision, and reviewing & editing the manuscript draft.
Conflicts of interest
The authors declare no conflict of interest for this work.
Acknowledgements
K. R. B. S. and A. K. S. are thankful to Principal, Govt. V. Y. T. PG. Autonomous College, Durg, Chhattisgarh, India, for providing apt support throughout this manuscript draft's writing process. J. S. expresses gratitude for the DST-INSPIRE faculty Fellowship, BHU (IoE grant), and UGC, New Delhi, for financial support. Finally, R. P. S. and V. N. are thankful to Hon'ble Vice-chancellor, Indira Gandhi National Tribal University, Amarkantak, M. P., India, for providing financial assistance to work smoothly and diligently.
References
- M. Liong, J. Lu, M. Kovochich, T. Xia, S. G. Ruehm, A. E. Nel, F. Tamanoi and J. I. Zink, ACS Nano, 2008, 2, 889–896 CrossRef CAS.
- I. Brigger, C. Dubernet and P. Couvreur, Adv. Drug Deliv. Rev., 2002, 54, 631–651 CrossRef CAS.
- L. Mora, K. Y. Chumbimuni-Torres, C. Clawson, L. Hernandez, L. Zhang and J. Wang, J. Control. Release, 2009, 140, 69–73 CrossRef CAS.
- R. Raliya, T. Singh Chadha, K. Haddad and P. Biswas, Curr. Pharm. Des., 2016, 22, 2481–2490 CrossRef CAS PubMed.
- J. Das, J. W. Han, Y.-J. Choi, H. Song, S.-G. Cho, C. Park, H. G. Seo and J.-H. Kim, Sci. Rep., 2016, 6, 29197 CrossRef CAS PubMed.
- P. Dong, K. P. Rakesh, H. M. Manukumar, Y. H. E. Mohammed, C. S. Karthik, S. Sumathi, P. Mallu and H.-L. Qin, Bioorg. Chem., 2019, 85, 325–336 CrossRef CAS PubMed.
- P. R. Solanki, A. Kaushik, V. V. Agrawal and B. D. Malhotra, NPG Asia Mater., 2011, 3, 17–24 CrossRef.
- R. P. Singh, Int. J. Electrochem., 2011, 2011, 1–30 CrossRef.
- R. P. Singh, in Food Safety and Human Health, Elsevier, 2019, pp. 285–318 Search PubMed.
- R. P. Singh, in Nanotechnology, ed. R. Prasad, M. Kumar and V. Kumar, Springer Singapore, Singapore, 2017, pp. 293–303 Search PubMed.
- R. P. Singh, in Plant Nanobionics, Springer, Cham, 2019, pp. 115–176 Search PubMed.
- R. P. Singh, J.-W. Choi, A. Tiwari and A. C. Pandey, in Biomedical Materials and Diagnostic Devices, John Wiley & Sons, Inc., Hoboken, NJ, USA, 2012, pp. 215–262 Search PubMed.
- S. Gurunathan, J. W. Han, D.-N. Kwon and J.-H. Kim, Nanoscale Res. Lett., 2014, 9, 373 CrossRef.
- M. R. Anilkumar, H. P. Nagaswarupa, H. Nagabhushana, S. C. Sharma, Y. S. Vidya, K. S. Anantharaju, S. C. Prashantha, C. Shivakuamra and K. Gurushantha, Spectrochim. Acta, Part A, 2015, 149, 703–713 CrossRef CAS.
- M. Smekalova, V. Aragon, A. Panacek, R. Prucek, R. Zboril and L. Kvitek, Vet. J., 2016, 209, 174–179 CrossRef CAS.
- T. Klaus, R. Joerger, E. Olsson and C.-G. Granqvist, Proc. Natl. Acad. Sci. U. S. A., 1999, 96, 13611–13614 CrossRef CAS PubMed.
- Y. Konishi, K. Ohno, N. Saitoh, T. Nomura, S. Nagamine, H. Hishida, Y. Takahashi and T. Uruga, J. Biotechnol., 2007, 128, 648–653 CrossRef CAS PubMed.
- I. Willner, R. Baron and B. Willner, Adv. Mater., 2006, 18, 1109–1120 CrossRef CAS.
- R. P. Singh and K. R. Singh, Nanomaterials in Bionanotechnology, CRC Press, Boca Raton, 2021 Search PubMed.
- S. Patil, A. Sandberg, E. Heckert, W. Self and S. Seal, Biomaterials, 2007, 28, 4600–4607 CrossRef CAS.
- J.-S. Lee and S.-C. Choi, Mater. Lett., 2004, 58, 390–393 CrossRef CAS.
- V. Kumar and S. K. Yadav, J. Chem.
Technol. Biotechnol., 2009, 84, 151–157 CrossRef CAS.
- N. K. Renuka, J. Alloys Compd., 2012, 513, 230–235 CrossRef CAS.
- N. Thakur, P. Manna and J. Das, J. Nanobiotechnology, 2019, 17, 84 CrossRef.
- O. L. Pop, A. Mesaros, D. C. Vodnar, R. Suharoschi, F. Tăbăran, L. Mageruan, I. S. Tódor, Z. Diaconeasa, A. Balint, L. Ciontea and C. Socaciu, Nanomaterials, 2020, 10, 1614 CrossRef CAS PubMed.
- S. M. Hirst, A. S. Karakoti, R. D. Tyler, N. Sriranganathan, S. Seal and C. M. Reilly, Small, 2009, 5, 2848–2856 CrossRef CAS.
- K. Krishnamoorthy, M. Veerapandian, L.-H. Zhang, K. Yun and S. J. Kim, J. Ind. Eng. Chem., 2014, 20, 3513–3517 CrossRef CAS.
- K. R. Singh, V. Nayak, T. Sarkar and R. P. Singh, RSC Adv., 2020, 10, 27194–27214 RSC.
- M. I. Morales, C. M. Rico, J. A. Hernandez-Viezcas, J. E. Nunez, A. C. Barrios, A. Tafoya, J. P. Flores-Marges, J. R. Peralta-Videa and J. L. Gardea-Torresdey, J. Agric. Food Chem., 2013, 61, 6224–6230 CrossRef CAS.
- J. Lim and S. A. Majetich, Nano Today, 2013, 8, 98–113 CrossRef CAS.
- N. T. K. Thanh and L. A. W. Green, Nano Today, 2010, 5, 213–230 CrossRef CAS.
- L. M. Parkes, R. Hodgson, L. T. Lu, L. D. Tung, I. Robinson, D. G. Fernig and N. T. K. Thanh, Contrast Media Mol. Imaging, 2008, 3, 150–156 CrossRef CAS.
- Y. Jiang, B. Wang, P. Liu, B. Wang, Y. Zhou, D. Wang, H. Liu and S. Dou, Nano Energy, 2020, 77, 105308 CrossRef CAS.
- C. Bao, B. Wang, P. Liu, H. Wu, Y. Zhou, D. Wang, H. Liu and S. Dou, Adv. Funct. Mater., 2020, 30, 2004891 CrossRef CAS.
- Y. Zhang, X. Li, Z. Huang, W. Zheng, C. Fan and T. Chen, Nanomedicine, 2013, 9, 74–84 CrossRef CAS PubMed.
- H. Acay, Prep. Biochem. Biotechnol., 2021, 51, 127–136 CrossRef CAS PubMed.
- A. M. Youssef, M. S. Abdel-Aziz and S. M. El-Sayed, Int. J. Biol. Macromol., 2014, 69, 185–191 CrossRef CAS PubMed.
- Y.-C. Yeh, B. Creran and V. M. Rotello, Nanoscale, 2012, 4, 1871–1880 RSC.
- M. L. Bruschi and L. A. S. de Toledo, Magnetochemistry, 2019, 5, 50 CrossRef CAS.
- Z. Duriagina, R. Holyaka, T. Tepla, V. Kulyk, P. Arras and E. Eyngorn, in Biomaterials in Regenerative Medicine, InTech, 2018 Search PubMed.
- G. S. Hikku, K. Jeyasubramanian and S. Vignesh Kumar, J. Ind. Eng. Chem, 2017, 52, 168–178 CrossRef CAS.
- J. M. Chimenos, A. I. Fernández, G. Villalba, M. Segarra, A. Urruticoechea, B. Artaza and F. Espiell, Water Res., 2003, 37, 1601–1607 CrossRef CAS.
- L. Wang, Y. Ye, X. Lu, Y. Wu, L. Sun, H. Tan, F. Xu and Y. Song, Electrochim. Acta, 2013, 114, 223–232 CrossRef CAS.
- G. Korotcenkov, Nanomaterials, 2020, 10, 1392 CrossRef CAS.
- T. Lopez, J. Catal., 1991, 127, 75–85 CrossRef CAS.
- D. S. Heroux, A. M. Volodin, V. I. Zaikovski, V. V. Chesnokov, A. F. Bedilo and K. J. Klabunde, J. Phys. Chem. B, 2004, 108, 3140–3144 CrossRef CAS.
- F. Al-Hazmi, F. Alnowaiser, A. A. Al-Ghamdi, A. A. Al-Ghamdi, M. M. Aly, R. M. Al-Tuwirqi and F. El-Tantawy, Superlattices Microstruct., 2012, 52, 200–209 CrossRef CAS.
- K. Ramanujam and M. Sundrarajan, J. Photochem. Photobiol., B, 2014, 141, 296–300 CrossRef CAS.
- Y. Haldorai and J.-J. Shim, Appl. Surf. Sci., 2014, 292, 447–453 CrossRef CAS.
- M. Fernandes, Adv. Mater. Lett., 2020, 11, 20081543 CrossRef CAS.
- P. K. Sasi, B. Gopchandran and K. G. Manoj, Vacuum, 2003, 68, 149–154 CrossRef.
- G. A. Sudhasree, S. Shakila Banu, A. Brindha and P. Kurian, Toxicol. Environ. Chem., 2014, 95, 743–754 CrossRef.
- M. E. Weeks, J. Chem. Educ., 1932, 9, 474 CrossRef CAS.
- V. N. Gladyshev, E. S. Arnér, M. J. Berry, R. Brigelius-Flohé, E. A. Bruford, R. F. Burk, B. A. Carlson, S. Castellano, L. Chavatte, M. Conrad, P. R. Copeland, A. M. Diamond, D. M. Driscoll, A. Ferreiro, L. Flohé, F. R. Green, R. Guigó, D. E. Handy, D. L. Hatfield, J. Hesketh, P. R. Hoffmann, A. Holmgren, R. J. Hondal, M. T. Howard, K. Huang, H.-Y. Kim, I. Y. Kim, J. Köhrle, A. Krol, G. V. Kryukov, B. J. Lee, B. C. Lee, X. G. Lei, Q. Liu, A. Lescure, A. V. Lobanov, J. Loscalzo, M. Maiorino, M. Mariotti, K. Sandeep Prabhu, M. P. Rayman, S. Rozovsky, G. Salinas, E. E. Schmidt, L. Schomburg, U. Schweizer, M. Simonović, R. A. Sunde, P. A. Tsuji, S. Tweedie, F. Ursini, P. D. Whanger and Y. Zhang, J. Biol. Chem., 2016, 291, 24036–24040 CrossRef CAS PubMed.
- A. P. Fernandes and V. Gandin, Biochim. Biophys. Acta, Gen. Subj., 2015, 1850, 1642–1660 CrossRef CAS PubMed.
- V. Nayak, K. R. Singh, A. K. Singh and R. P. Singh, New J. Chem., 2021, 45, 2849–2878 RSC.
- A. Sirelkhatim, S. Mahmud, A. Seeni, N. H. M. Kaus, L. C. Ann, S. K. M. Bakhori, H. Hasan and D. Mohamad, Nano-Micro Lett., 2015, 7, 219–242 CrossRef CAS.
- B. Wang, T. Ruan, Y. Chen, F. Jin, L. Peng, Y. Zhou, D. Wang and S. Dou, Energy Storage Mater., 2020, 24, 22–51 CrossRef.
- F. Wang, B. Wang, J. Li, B. Wang, Y. Zhou, D. Wang, H. Liu and S. Dou, ACS Nano, 2021, 15, 2197–2218 CrossRef CAS.
- F. Jin, B. Wang, J. Wang, Y. Wang, Y. Ning, J. Yang, Z. Zhang, P. Liu, Y. Zhou, D. Wang, H. Liu and S. Dou, Matter, 2021, 4, 1768–1800 CrossRef.
- K. R. Singh, P. Sridevi and R. P. Singh, Eng. Rep., 2020, 2, e12238 CAS.
- K. P. Singh and S. Gupta, RSC Adv., 2014, 4, 13215–13230 RSC.
- R. Pratap Singh, J. Bioanal. Biomed., 2016, 8(4) DOI:10.4172/1948-593X1000e143.
- Bionanomaterials, ed. R. P. Singh and K. R. Singh, IOP Publishing, 2021 Search PubMed.
- K. R. Singh, P. R. Solanki, B. D. Malhotra, A. C. Pandey and R. P. Singh, in Nanomaterials in Bionanotechnology, CRC Press, Boca Raton, 2021, pp. 1–35 Search PubMed.
- K. R. Singh, V. Nayak and R. P. Singh, in Bionanomaterials, IOP Publishing, 2021 Search PubMed.
- Y. Zhang, J. Li, H. Jiang, C. Zhao and X. Wang, RSC Adv., 2016, 6, 63331–63337 RSC.
- S. Kumar, V. K. Meena, P. P. Hazari, S. K. Sharma and R. K. Sharma, Eur. J. Pharm. Sci., 2018, 117, 362–370 CrossRef CAS.
- E. S. M. Lee, B. Shuter, J. Chan, M. S. K. Chong, J. Ding, S.-H. Teoh, O. Beuf, A. Briguet, K. C. Tam, M. Choolani and S.-C. Wang, Biomaterials, 2010, 31, 3296–3306 CrossRef CAS PubMed.
- P. C. Nagajyothi, P. Muthuraman, T. V. M. Sreekanth, D. H. Kim and J. Shim, Arab. J. Chem., 2017, 10, 215–225 CrossRef CAS.
- E. S. Al-Sheddi, N. N. Farshori, M. M. Al-Oqail, S. M. Al-Massarani, Q. Saquib, R. Wahab, J. Musarrat, A. A. Al-Khedhairy and M. A. Siddiqui, Bioinorg. Chem. Appl., 2018, 2018, 1–12 CrossRef.
- A. Pugazhendhi, R. Prabhu, K. Muruganantham, R. Shanmuganathan and S. Natarajan, J. Photochem. Photobiol., B, 2019, 190, 86–97 CrossRef CAS.
- A. Bayrami, S. Parvinroo, A. Habibi-Yangjeh and S. Rahim Pouran, Artif. Cells, Nanomedicine, Biotechnol., 2018, 46, 730–739 CrossRef CAS.
- S. Pattanayak, M. M. R. Mollick, D. Maity, S. Chakraborty, S. K. Dash, S. Chattopadhyay, S. Roy, D. Chattopadhyay and M. Chakraborty, J. Saudi Chem. Soc., 2017, 21, 673–684 CrossRef CAS.
- B. Kumar, K. Smita, L. Cumbal and A. Debut, Bioinorg. Chem. Appl., 2014, 2014, 1–7 Search PubMed.
- G. Maheshwaran, M. Malai Selvi, R. Selva Muneeswari, A. Nivedhitha Bharathi, M. Krishna Kumar and S. Sudhahar, Adv. Powder Technol., 2021, 32(6), 1963–1971 CrossRef CAS.
- A. Khalid, H. Ullah, M. Ul-Islam, R. Khan, S. Khan, F. Ahmad and T. Khan, RSC Adv., 2017, 7, 47662 RSC.
- D. Wang, L. Cui, X. Chang and D. Guan, J. Photochem. Photobiol., B, 2020, 202, 111652 CrossRef CAS PubMed.
- T. Singh, K. Jyoti, A. Patnaik, A. Singh, R. Chauhan and S. S. Chandel, J. Genet. Eng. Biotechnol., 2017, 15, 31–39 CrossRef PubMed.
- R. Singh, P. Wagh, S. Wadhwani, S. Gaidhani, A. Kumbhar, J. Bellare and B. A. Chopade, Int. J. Nanomed., 2013, 201, 4277–4290 Search PubMed.
- S. Ghosh, S. Patil, M. Ahire, R. Kitture, D. D. Gurav, A. M. Jabgunde, S. Kale, K. Pardesi, V. Shinde, J. Bellare, D. D. Dhavale and B. A. Chopade, J. Nanobiotechnology, 2012, 10, 17 CrossRef CAS PubMed.
- A. K. Singh, M. Talat, D. P. Singh and O. N. Srivastava, J. Nanoparticle Res., 2010, 12, 1667–1675 CrossRef CAS.
- S. S. Shankar, A. Rai, A. Ahmad and M. Sastry, J. Colloid Interface Sci., 2004, 275, 496–502 CrossRef CAS PubMed.
- S. P. Chandran, M. Chaudhary, R. Pasricha, A. Ahmad and M. Sastry, Biotechnol. Prog., 2006, 22, 577–583 CrossRef CAS.
- P. S. Vankar and D. Bajpai, Indian J. Biochem. Biophys., 2010, 47, 157–160 CAS.
- A. Annamalai, B. Mitra, D. Vishnudas and S. B. Sant, Drug Invent. Today, 2012, 4, 340–344 Search PubMed.
- M. Khan, M. Khan, S. F. Adil, M. N. Tahir, W. Tremel, H. Z. Alkhathlan, A. Al-Warthan and M. R. H. Siddiqui, Int. J. Nanomed., 2013, 8(1), 1507–1516 Search PubMed.
- P. Singh, K. R. Singh, J. Singh, S. N. Das and R. P. Singh, RSC Adv., 2021, 11, 18050–18060 RSC.
- K. Anu, G. Singaravelu, K. Murugan and G. Benelli, J. Clust. Sci., 2017, 28, 551–563 CrossRef CAS.
- S. Y. S. Zeebaree, A. Y. S. Zeebaree and O. I. H. Zebari, Sustainable Chem. Pharm., 2020, 15, 100210 CrossRef.
- J. Vyas and S. Rana, Int. J. Curr. Pharm. Res., 2017, 9, 147 CrossRef CAS.
- B. Fardsadegh and H. Jafarizadeh-Malmiri, Green Process. Synth., 2019, 8, 399–407 CAS.
- V. V. Makarov, S. S. Makarova, A. J. Love, O. V. Sinitsyna, A. O. Dudnik, I. V. Yaminsky, M. E. Taliansky and N. O. Kalinina, Langmuir, 2014, 30, 5982–5988 CrossRef CAS PubMed.
- J. Y. Song, E.-Y. Kwon and B. S. Kim, Bioprocess Biosyst. Eng., 2010, 33, 159–164 CrossRef CAS PubMed.
- S. Gurunathan, K. Kalishwaralal, R. Vaidyanathan, D. Venkataraman, S. R. K. Pandian, J. Muniyandi, N. Hariharan and S. H. Eom, Colloids Surf. B Biointerfaces, 2009, 74, 328–335 CrossRef CAS PubMed.
- K. S. L. Jain, S. Kachhwaha, R. Jain and G. Srivastava, Indian J. Exp. Biol., 2010, 48, 1152–1156 Search PubMed.
- Z. S. Zhang, Q. Li, Y. Lu, D. Sun, X. Lin, X. Deng and N. He, J. Chem. Technol. Biotechnol., 2005, 80, 285–290 CrossRef.
- M. M. Ganesh Babu and P. Gunasekaran, Colloids Surf. B Biointerfaces, 2009, 74, 191–195 CrossRef CAS PubMed.
- M. M. Juibari, S. Abbasalizadeh, G. S. Jouzani and M. Noruzi, Mater. Lett., 2011, 65, 1014–1017 CrossRef CAS.
- L. Sintubin, W. De Windt, J. Dick, J. Mast, D. van der Ha, W. Verstraete and N. Boon, Appl. Microbiol. Biotechnol., 2009, 84, 741–749 CrossRef CAS.
- R. Y. Sweeney, C. Mao, X. Gao, J. L. Burt, A. M. Belcher, G. Georgiou and B. L. Iverson, Chem. Biol., 2004, 11, 1553–1559 CrossRef CAS PubMed.
- R. S. Oremland, M. J. Herbel, J. S. Blum, S. Langley, T. J. Beveridge, P. M. Ajayan, T. Sutto, A. V. Ellis and S. Curran, Appl. Environ. Microbiol., 2004, 70, 52–60 CrossRef CAS.
- V. Bansal, A. Bharde, R. Ramanathan and S. K. Bhargava, Adv. Colloid Interface Sci., 2012, 179–182, 150–168 CrossRef CAS.
- R. V. Afreen and E. Ranganath, Int. J. Environ. Sci., 2011, 1, 1582–1592 Search PubMed.
- S. S. Birla, V. V. Tiwari, A. K. Gade, A. P. Ingle, A. P. Yadav and M. K. Rai, Lett. Appl. Microbiol., 2009, 48, 173–179 CrossRef CAS PubMed.
- M. Karbasian, S. M. Atyabi, S. D. Siadat, S. B. Momen and D. Norouzian, Am. J. Agric. Biol. Sci., 2008, 3(1), 433–437 CrossRef.
- A. Ahmad, P. Mukherjee, S. Senapati, D. Mandal, M. I. Khan, R. Kumar and M. Sastry, Colloids Surf. B Biointerfaces, 2003, 28, 313–318 CrossRef CAS.
- A. Ingle, M. Rai, A. Gade and M. Bawaskar, J. Nanoparticle Res., 2009, 11, 2079–2085 CrossRef CAS.
- V. C. Verma, R. N. Kharwar and A. C. Gange, Nanomedicine, 2010, 5, 33–40 CrossRef CAS PubMed.
- N. Vigneshwaran, N. M. Ashtaputre, P. V. Varadarajan, R. P. Nachane, K. M. Paralikar and R. H. Balasubramanya, Mater. Lett., 2007, 61, 1413–1418 CrossRef CAS.
- R. Sanghi and P. Verma, Bioresour. Technol., 2009, 100, 501–504 CrossRef CAS PubMed.
- Y. Qian, H. Yu, D. He, H. Yang, W. Wang, X. Wan and L. Wang, Bioprocess Biosyst. Eng., 2013, 36, 1613–1619 CrossRef CAS.
- K. Ishida, T. F. Cipriano, G. M. Rocha, G. Weissmüller, F. Gomes, K. Miranda and S. Rozental, Mem. Inst. Oswaldo Cruz, 2013, 109, 220–228 CrossRef PubMed.
- E. Castro-Longoria, J. Microbiol. Biotechnol., 2012, 22, 1000–1004 CrossRef CAS PubMed.
- T. L. Riddin, M. Gericke and C. G. Whiteley, Nanotechnology, 2006, 17, 3482–3489 CrossRef CAS PubMed.
- J. Sarkar, P. Dey, S. Saha and K. Acharya, Micro & Nano Lett., 2011, 6, 599 CrossRef CAS.
- S. A. Khan and A. Ahmad, Mater. Res. Bull., 2013, 48, 4134–4138 CrossRef CAS.
- X. Zhang, X. He, K. Wang, Y. Wang, H. Li and W. Tan, J. Nanosci. Nanotechnol., 2009, 9, 5738–5744 CrossRef CAS.
- R. Balagurunathan, M. Radhakrishnan, R. B. Rajendran and D. Velmurugan, Indian J. Biochem. Biophys., 2011, 48, 331–335 CAS.
- R. Bhat, V. G. Sharanabasava, R. Deshpande, U. Shetti, G. Sanjeev and A. Venkataraman, J. Photochem. Photobiol., B, 2013, 125, 63–69 CrossRef CAS PubMed.
- S. Senthamilselvi, P. Kumar, A. L. Prabha and M. Govindaraju, Nano Biomed. Eng., 2013, 5(2), 102–106 Search PubMed.
- A. Ahmad, P. Mukherjee, D. Mandal, S. Senapati, M. I. Khan, R. Kumar and M. Sastry, J. Am. Chem. Soc., 2002, 124, 12108–12109 CrossRef CAS PubMed.
- K. Prasad and A. K. Jha, J. Colloid Interface Sci., 2010, 342, 68–72 CrossRef CAS.
- A. Syed and A. Ahmad, Spectrochim. Acta, Part A, 2013, 106, 41–47 CrossRef CAS.
- S. A. Kumar, A. A. Ansary, A. Ahmad and M. I. Khan, J. Biomed. Nanotechnol., 2007, 3, 190–194 CrossRef CAS.
- G.-Q. Chen, Z.-J. Zou, G.-M. Zeng, M. Yan, J.-Q. Fan, A.-W. Chen, F. Yang, W.-J. Zhang and L. Wang, Chemosphere, 2011, 83, 1201–1207 CrossRef CAS.
- X. Meng, G. Xu, Q.-L. Zhou, J.-P. Wu and L.-R. Yang, Food Chem., 2014, 143, 319–324 CrossRef CAS.
- A. Bharde, D. Rautaray, V. Bansal, A. Ahmad, I. Sarkar, S. M. Yusuf, M. Sanyal and M. Sastry, Small, 2006, 2, 135–141 CrossRef CAS.
- D. Rautaray, A. Sanyal, S. D. Adyanthaya, A. Ahmad and M. Sastry, Langmuir, 2004, 20, 6827–6833 CrossRef CAS PubMed.
- V. Bansal, D. Rautaray, A. Bharde, K. Ahire, A. Sanyal, A. Ahmad and M. Sastry, J. Mater. Chem., 2005, 15, 2583 RSC.
- A. K. Jha, K. Prasad and A. R. Kulkarni, Colloids Surf. B Biointerfaces, 2009, 71, 226–229 CrossRef CAS.
- S. K. Nune, P. Gunda, P. K. Thallapally, Y.-Y. Lin, M. Laird Forrest and C. J. Berkland, Expert Opin. Drug Deliv., 2009, 6, 1175–1194 CrossRef CAS PubMed.
- W. Xu, P. Cui, E. Happonen, J. Leppänen, L. Liu, J. Rantanen, D. Majda, A. Saukko, R. Thapa, T. Nissinen, T. Tynkkynen, J. Töyräs, L. Fan, W. Liu and V.-P. Lehto, ACS Appl. Mater. Interfaces, 2020, 12, 47233–47244 CrossRef CAS.
- S. Farahani, N. Riyahi alam, E. Gorji, R. Rahnamafar, S. Fazli, H. Khosravi, M. Pakravan, V. Shahabian and S. Haghgoo, Radiother. Oncol., 2017, 123, S963–S964 CrossRef.
- Y. Wang, W. Wang and W. Song, Electrochim. Acta, 2011, 56, 10191–10196 CrossRef CAS.
- S. Zhou, D. Wei, H. Shi, X. Feng, K. Xue, F. Zhang and W. Song, Talanta, 2013, 107, 349–355 CrossRef CAS PubMed.
- H. Shekarchizadeh, M. Kadivar and A. A. Ensafi, Chin. J. Catal., 2013, 34, 1208–1215 CrossRef CAS.
- K. Khun, Z. H. Ibupoto, J. Lu, M. S. AlSalhi, M. Atif, A. A. Ansari and M. Willander, Sensor. Actuator. B Chem., 2012, 173, 698–703 CrossRef CAS.
- A. Umar, R. Ahmad, S. W. Hwang, S. H. Kim, A. Al-Hajry and Y. B. Hahn, Electrochim. Acta, 2014, 135, 396–403 CrossRef CAS.
- F. Gao, D. Zheng, H. Tanaka, F. Zhan, X. Yuan, F. Gao and Q. Wang, Mater. Sci. Eng. C, 2015, 57, 279–287 CrossRef CAS.
- N. Quoc Dung, D. Patil, H. Jung and D. Kim, Biosens. Bioelectron., 2013, 42, 280–286 CrossRef CAS PubMed.
- J. Luo, S. Jiang, H. Zhang, J. Jiang and X. Liu, Anal. Chim. Acta, 2012, 709, 47–53 CrossRef CAS PubMed.
- X. Zhou, H. Nie, Z. Yao, Y. Dong, Z. Yang and S. Huang, Sensor. Actuator. B Chem., 2012, 168, 1–7 CrossRef CAS.
- M. M. Rahman, A. J. S. Ahammad, J.-H. Jin, S. J. Ahn and J.-J. Lee, Sensors, 2010, 10, 4855–4886 CrossRef CAS.
- A. E. Nel, L. Mädler, D. Velegol, T. Xia, E. M. Hoek, P. Somasundaran, F. Klaessig, V. Castranova and M. Thompson, Nat. Mater., 2009, 8, 543–557 CrossRef CAS.
- K. Mondal and A. Sharma, RSC Adv., 2016, 6, 94595–94616 RSC.
- H. Sim, J.-H. Kim, S.-K. Lee, M.-J. Song, D.-H. Yoon, D.-S. Lim and S.-I. Hong, Thin Solid Films, 2012, 520, 7219–7223 CrossRef CAS.
- C.-W. Kung, C.-Y. Lin, Y.-H. Lai, R. Vittal and K.-C. Ho, Biosens. Bioelectron., 2011, 27, 125–131 CrossRef CAS PubMed.
- S. Yu, X. Peng, G. Cao, M. Zhou, L. Qiao, J. Yao and H. He, Electrochim. Acta, 2012, 76, 512–517 CrossRef CAS.
- Y. Mu, D. Jia, Y. He, Y. Miao and H.-L. Wu, Biosens. Bioelectron., 2011, 26, 2948–2952 CrossRef CAS PubMed.
- Y. Lei, X. Yan, N. Luo, Y. Song and Y. Zhang, Colloid. Surface. Physicochem. Eng. Aspect., 2010, 361, 169–173 CrossRef CAS.
- M. Mathew and N. Sandhyarani, Electrochim. Acta, 2013, 108, 274–280 CrossRef CAS.
- A. Fulati, S. M. U. Ali, M. H. Asif, N. H. Alvi, M. Willander, C. Brännmark, P. Strålfors, S. I. Börjesson, F. Elinder and B. Danielsson, Sensor. Actuator. B Chem., 2010, 150, 673–680 CrossRef CAS.
- F. Hu, S. Chen, C. Wang, R. Yuan, Y. Chai, Y. Xiang and C. Wang, J. Mol. Catal. B Enzym., 2011, 72, 298–304 CrossRef CAS.
- M. Jamal, M. Hasan, A. Mathewson and K. M. Razeeb, Biosens. Bioelectron., 2013, 40, 213–218 CrossRef CAS PubMed.
- C. Lu, Q. Shen, X. Zhao, J. Zhu, X. Guo and W. Hou, Sensor. Actuator. B Chem., 2010, 150, 200–205 CrossRef CAS.
- M. A. Karimi, F. Banifatemeh, A. Hatefi-Mehrjardi, H. Tavallali, Z. Eshaghia and G. Deilamy-Rad, Mater. Res. Bull., 2015, 70, 856–864 CrossRef CAS.
- M. Liu, R. Liu and W. Chen, Biosens. Bioelectron., 2013, 45, 206–212 CrossRef CAS PubMed.
- C.-Y. Lin, Y.-H. Lai, A. Balamurugan, R. Vittal, C.-W. Lin and K.-C. Ho, Talanta, 2010, 82, 340–347 CrossRef CAS.
- Q. Liu, L. Zhang, H. Li, Q. Jia, Y. Jiang, Y. Yang and R. Zhu, Mater. Sci. Eng., C, 2015, 55, 193–200 CrossRef CAS PubMed.
- K. L. Low, S. H. S. Zein, S. H. Tan, D. S. McPhail and A. R. Boccaccini, Ceram. Int., 2011, 37, 2429–2435 CrossRef CAS.
- J. Zhuang, T. Cheng, L. Gao, Y. Luo, Q. Ren, D. Lu, F. Tang, X. Ren, D. Yang, J. Feng, J. Zhu and X. Yan, Toxicon, 2010, 55, 145–152 CrossRef CAS PubMed.
- M. Tyagi, M. Tomar and V. Gupta, Biosens. Bioelectron., 2013, 41, 110–115 CrossRef CAS PubMed.
- S. M. Usman Ali, N. H. Alvi, Z. Ibupoto, O. Nur, M. Willander and B. Danielsson, Sensor. Actuator. B Chem., 2011, 152, 241–247 CrossRef CAS.
- X. Cao, Y. Ye and S. Liu, Anal. Biochem., 2011, 417, 1–16 CrossRef CAS.
- E. B. Aydın and M. K. Sezgintürk, Trac. Trends Anal. Chem., 2017, 97, 309–315 CrossRef.
- R. L. Siegel, K. D. Miller and A. Jemal, Ca - Cancer J. Clin., 2016, 66, 7–30 CrossRef.
- R. Aswini, S. Murugesan and K. Kannan, Int. J. Environ. Anal. Chem., 2020, 1–11 CrossRef.
- S. Kumar, M. S. Tomar and A. Acharya, Colloids Surf. B Biointerfaces, 2015, 126, 546–552 CrossRef CAS.
- Y. Feng, J. Su, Z. Zhao, W. Zheng, H. Wu, Y. Zhang and T. Chen, Dalton Trans., 2014, 43, 1854–1861 RSC.
- J. Zou, S. Su, Z. Chen, F. Liang, Y. Zeng, W. Cen, X. Zhang, Y. Xia and D. Huang, Artif. Cells, Nanomedicine, Biotechnol., 2019, 47, 3456–3464 CrossRef CAS.
- Y. Ren, T. Zhao, G. Mao, M. Zhang, F. Li, Y. Zou, L. Yang and X. Wu, Int. J. Biol. Macromol., 2013, 57, 57–62 CrossRef CAS PubMed.
- J. Emerit, M. Edeas and F. Bricaire, Biomed. Pharmacother., 2004, 58, 39–46 CrossRef CAS PubMed.
- R. P. Singh, S. Sharad and S. Kapur, J. Indian Acad. Clin. Med., 2004, 5, 218–225 Search PubMed.
- E. Behzadi, R. Sarsharzadeh, M. Nouri, F. Attar, K. Akhtari, K. Shahpasand and M. Falahati, Int. J. Nanomed., 2019, 14, 257–270 CrossRef CAS PubMed.
- K. Karthik, S. Dhanuskodi, C. Gobinath, S. Prabukumar and S. Sivaramakrishnan, J. Photochem. Photobiol., B, 2019, 190, 8–20 CrossRef CAS PubMed.
- K. Srinivasan, J. Jabaseelan Samuel, V. Poopathi and N. Grace, Mater. Today: Proc., 2019, 9, 428–437 CAS.
- F. Gulbagca, A. Aygün, M. Gülcan, S. Ozdemir, S. Gonca and F. Şen, Appl. Organomet. Chem., 2018, 14, 257–270 Search PubMed.
- G. Sharmila, M. Thirumarimurugan and C. Muthukumaran, Microchem. J., 2019, 145, 578–587 CrossRef CAS.
- K. Balan, W. Qing, Y. Wang, X. Liu, T. Palvannan, Y. Wang, F. Ma and Y. Zhang, RSC Adv., 2016, 6, 40162–40168 RSC.
- S. Prabhu, S. Vinodhini, C. Elanchezhiyan and D. Rajeswari, J. Diabetes, 2018, 10, 28–42 CrossRef CAS PubMed.
- A. Abdel Moneim, S. Al-Quraishy and M. A. Dkhil, Int. J. Nanomed., 2015, 6741 CrossRef PubMed.
- H. H. Ahmed, M. D. Abd El-Maksoud, A. E. Abdel Moneim and H. A. Aglan, Biol. Trace Elem. Res., 2017, 177, 267–280 CrossRef CAS PubMed.
- A. C. Maritim, R. A. Sanders and J. B. Watkins, J. Biochem. Mol. Toxicol., 2003, 17, 24–38 CrossRef CAS PubMed.
- N. Pourkhalili, A. Hosseini, A. Nili-Ahmadabadi, S. Hassani, M. Pakzad, M. Baeeri, A. Mohammadirad and M. Abdollahi, World J. Diabetes, 2011, 2, 204 CrossRef PubMed.
- N. Pourkhalili, A. Hosseini, A. Nili-Ahmadabadi, M. Rahimifard, M. Navaei-Nigjeh, S. Hassani, M. Baeeri and M. Abdollahi, Toxicol. Mech. Methods, 2012, 22, 476–482 CrossRef CAS PubMed.
- A. Viscido, A. Capannolo, G. Latella, R. Caprilli and G. Frieri, J. Crohns Colitis, 2014, 8, 903–918 CrossRef PubMed.
- H. Yang, S.-Y. Fung, S. Xu, D. P. Sutherland, T. R. Kollmann, M. Liu and S. E. Turvey, ACS Nano, 2015, 9, 6774–6784 CrossRef CAS PubMed.
- B. Moldovan, L. David, A. Vulcu, L. Olenic, M. Perde-Schrepler, E. Fischer-Fodor, I. Baldea, S. Clichici and G. A. Filip, Mater. Sci. Eng. C, 2017, 79, 720–727 CrossRef CAS PubMed.
- A. M. K. Seethalakshmi, J. Nanomed. Nanotechnol., 2015, 3(2), 1–5 Search PubMed.
- A. Gojova, J.-T. Lee, H. S. Jung, B. Guo, A. I. Barakat and I. M. Kennedy, Inhal. Toxicol., 2009, 21, 123–130 CrossRef CAS PubMed.
- J. Wang, Y. Zhang, Y. Yuan and T. Yue, Food Chem. Toxicol., 2014, 68, 183–189 CrossRef CAS.
- H. Kong, J. Yang, Y. Zhang, Y. Fang, K. Nishinari and G. O. Phillips, Int. J. Biol. Macromol., 2014, 65, 155–162 CrossRef CAS.
- E. Yadav, D. Singh, P. Yadav and A. Verma, RSC Adv., 2018, 8, 21621–21635 RSC.
- H. Agarwal, A. Nakara and V. K. Shanmugam, Biomed. Pharmacother., 2019, 109, 2561–2572 CrossRef CAS PubMed.
- A. Ranjbar, S. Soleimani Asl, F. Firozian, H. Heidary Dartoti, S. Seyedabadi, M. Taheri Azandariani and M. Ganji, J. Mol. Neurosci., 2018, 66, 420–427 CrossRef CAS.
- S.-J. Kim and B. H. Chung, Carbohydr. Polym., 2016, 150, 400–407 CrossRef CAS PubMed.
- D. Suresh, P. C. Nethravathi, Udayabhanu, H. Rajanaika, H. Nagabhushana and S. C. Sharma, Mater. Sci. Semicond. Process., 2015, 31, 446–454 CrossRef CAS.
- Z. Salari, A. Ameri, H. Forootanfar, M. Adeli-Sardou, M. Jafari, M. Mehrabani and M. Shakibaie, J. Trace Elem. Med. Biol., 2017, 39, 116–123 CrossRef CAS.
- F. Duman, I. Ocsoy and F. O. Kup, Mater. Sci. Eng. C, 2016, 60, 333–338 CrossRef CAS.
- G. A. K. Rohit Guin and A. Shakila Banu, Int. J. Res. Pharm. Sci., 2015, 7, 60–65 Search PubMed.
- Udayabhanu, P. C. Nethravathi, M. A. Pavan Kumar, D. Suresh, K. Lingaraju, H. Rajanaika, H. Nagabhushana and S. C. Sharma, Mater. Sci. Semicond. Process., 2015, 33, 81–88 CrossRef CAS.
- S. Rajeshkumar, S. V. Kumar, A. Ramaiah, H. Agarwal, T. Lakshmi and S. M. Roopan, Enzyme Microb. Technol., 2018, 117, 91–95 CrossRef CAS PubMed.
- G. Spigno, L. Tramelli and D. M. De Faveri, J. Food Eng., 2007, 81, 200–208 CrossRef CAS.
- K. Brindhadevi, M. S. Samuel, T. N. Verma, S. Vasantharaj, S. Sathiyavimal, M. Saravanan, A. Pugazhendhi and P. A. Duc, Biocatal. Agric. Biotechnol., 2020, 28, 101730 CrossRef.
- D. Das, B. C. Nath, P. Phukon and S. K. Dolui, Colloids Surf. B Biointerfaces, 2013, 101, 430–433 CrossRef CAS PubMed.
- A. R. Unnithan, A. Ramachandra Kurup Sasikala, Y. Sathishkumar, Y. S. Lee, C. H. Park and C. S. Kim, Ceram. Int., 2014, 40, 12003–12012 CrossRef CAS.
- B. Balraj, N. Senthilkumar, I. Vetha Potheher and M. Arulmozhi, Mater. Sci. Eng. B, 2018, 231, 121–127 CrossRef CAS.
- C. Vijilvani, M. R. Bindhu, F. C. Frincy, M. S. AlSalhi, S. Sabitha, K. Saravanakumar, S. Devanesan, M. Umadevi, M. J. Aljaafreh and M. Atif, J. Photochem. Photobiol., B, 2020, 202, 111713 CrossRef CAS PubMed.
- T. Huang, S. Kumari, H. Herold, H. Bargel, T. B. Aigner, D. E. Heath, N. M. O'Brien-Simpson, A. J. O'Connor and T. Scheibel, Int. J. Nanomed., 2020, 15, 4275–4288 CrossRef CAS.
- C. Mahendra, M. N. Chandra, M. Murali, M. R. Abhilash, S. B. Singh, S. Satish and M. S. Sudarshana, Process Biochem., 2020, 89, 220–226 CrossRef CAS.
- S. Rajendran, S. S. Prabha, R. J. Rathish, G. Singh and A. Al-Hashem, in Nanotoxicity, Elsevier, 2020, pp. 275–281 Search PubMed.
- A. Pugazhendhi, S. S. Kumar, M. Manikandan and M. Saravanan, Microb. Pathog., 2018, 122, 84–89 CrossRef CAS.
- N.-Y. Nguyen, B. N. An, M.-V. Le and H. A. Hoang, Biocontrol Sci., 2020, 25, 159–165 CrossRef CAS PubMed.
- K. W. E. Denson, Toxicon, 1969, 7, 5–11 CrossRef CAS PubMed.
- A. Lateef, S. A. Ojo and S. M. Oladejo, Process Biochem., 2016, 51, 1406–1412 CrossRef CAS.
- K. Kalishwaralal, V. Deepak, S. Ram Kumar Pandian, M. Kottaisamy, S. BarathManiKanth, B. Kartikeyan and S. Gurunathan, Colloids Surf. B Biointerfaces, 2010, 77, 257–262 CrossRef CAS PubMed.
- J. S. Ajarem, S. N. Maodaa, A. A. Allam, M. M. Taher and M. Khalaf, J. Clust. Sci., 2021 DOI:10.1007/s10876-021-02004-9.
- K. Lingaraju, H. Raja Naika, H. Nagabhushana, K. Jayanna, S. Devaraja and G. Nagaraju, Arab. J. Chem., 2020, 13, 4712–4719 CrossRef CAS.
- P. O. Akinola, A. Lateef, T. B. Asafa, L. S. Beukes, A. S. Hakeem and H. M. Irshad, Heliyon, 2020, 6, e04610 CrossRef CAS PubMed.
- P. Sanchez-Moreno, J. L. Ortega-Vinuesa, J. M. Peula-Garcia, J. A. Marchal and H. Boulaiz, Curr. Drug Targets, 2018, 19, 339–359 CrossRef CAS PubMed.
- H.-C. Huang, S. Barua, G. Sharma, S. K. Dey and K. Rege, J. Control. Release, 2011, 155, 344–357 CrossRef CAS PubMed.
- P. Tartaj, M. P. Morales, S. Veintemillas-Verdaguer, T. lez-Carreo and C. J. Serna, J. Phys. D Appl. Phys., 2003, 36, R182–R197 CrossRef CAS.
- S. Pinel, N. Thomas, C. Boura and M. Barberi-Heyob, Adv. Drug Deliv. Rev., 2019, 138, 344–357 CrossRef CAS PubMed.
- Y. Liu, J. Zhuang, X. Zhang, C. Yue, N. Zhu, L. Yang, Y. Wang, T. Chen, Y. Wang and L. W. Zhang, Toxicol. Lett., 2017, 275, 39–48 CrossRef CAS PubMed.
- C. G. Kumar and Y. Poornachandra, Colloids Surf. B Biointerfaces, 2015, 125, 110–119 CrossRef CAS.
- I. Kalashnikova, J. Mazar, C. J. Neal, A. L. Rosado, S. Das, T. J. Westmoreland and S. Seal, Nanoscale, 2017, 9, 10375–10387 RSC.
- F. Muhammad, A. Wang, W. Qi, S. Zhang and G. Zhu, ACS Appl. Mater. Interfaces, 2014, 6, 19424–19433 CrossRef CAS PubMed.
- T. Liu, L. Zeng, W. Jiang, Y. Fu, W. Zheng and T. Chen, Nanomedicine Nanotechnology, Biol. Med., 2015, 11, 947–958 CrossRef CAS PubMed.
- W. Liu, X. Li, Y.-S. Wong, W. Zheng, Y. Zhang, W. Cao and T. Chen, ACS Nano, 2012, 6, 6578–6591 CrossRef CAS PubMed.
- P. Sathishkumar, Z. Li, R. Govindan, R. Jayakumar, C. Wang and F. Long Gu, Appl. Surf. Sci., 2021, 536, 147741 CrossRef CAS.
- R. J. Casson, G. Chidlow, A. Ebneter, J. P. M. Wood, J. Crowston and I. Goldberg, Clin. Exp. Ophthalmol., 2012, 40, 350–357 CrossRef.
- S. B. Dunnett and A. Björklund, Nature, 1999, 399, A32–A39 CrossRef CAS PubMed.
- A. A. Dayem, B. Kim, S. Gurunathan, H. Y. Choi, G. Yang, S. K. Saha, D. Han, J. Han, K. Kim, J.-H. Kim and S.-G. Cho, Biotechnol. J., 2014, 9, 934–943 CrossRef PubMed.
- H. Vural, H. Demirin, Y. Kara, I. Eren and N. Delibas, J. Trace Elem. Med. Biol., 2010, 24, 169–173 CrossRef CAS PubMed.
- B. Rita Cardoso, V. Silva Bandeira, W. Jacob-Filho and S. M. Franciscato Cozzolino, J. Trace Elem. Med. Biol., 2014, 28, 422–426 CrossRef PubMed.
- M. K. Yu, J. Park and S. Jon, Theranostics, 2012, 2, 3–44 CrossRef CAS PubMed.
- A. Shahar, K. Patel, R. D. Semba, S. Bandinelli, D. R. Shahar, L. Ferrucci and J. M. Guralnik, Mov. Disord., 2010, 25, 1909–1915 CrossRef PubMed.
- B. Huang, J. Zhang, J. Hou and C. Chen, Free Radic. Biol. Med., 2003, 35, 805–813 CrossRef CAS.
- K. Apel and H. Hirt, Annu. Rev. Plant Biol., 2004, 55, 373–399 CrossRef CAS PubMed.
- B. A. Rzigalinski, K. Meehan, R. M. Davis, Y. Xu, W. C. Miles and C. A. Cohen, Nanomedicine, 2006, 1, 399–412 CrossRef CAS PubMed.
- M. Das, S. Patil, N. Bhargava, J.-F. Kang, L. M. Riedel, S. Seal and J. J. Hickman, Biomaterials, 2007, 28, 1918–1925 CrossRef CAS PubMed.
- D. Schubert, R. Dargusch, J. Raitano and S.-W. Chan, Biochem. Biophys. Res. Commun., 2006, 342, 86–91 CrossRef CAS PubMed.
- J. Geng, M. Li, J. Ren, E. Wang and X. Qu, Angew. Chem., Int. Ed., 2011, 50, 4184–4188 CrossRef CAS PubMed.
- C. K. Kim, T. Kim, I.-Y. Choi, M. Soh, D. Kim, Y.-J. Kim, H. Jang, H.-S. Yang, J. Y. Kim, H.-K. Park, S. P. Park, S. Park, T. Yu, B.-W. Yoon, S.-H. Lee and T. Hyeon, Angew. Chem., Int. Ed., 2012, 51, 11039–11043 CrossRef CAS PubMed.
- E. Suprun, T. Bulko, A. Lisitsa, O. Gnedenko, A. Ivanov, V. Shumyantseva and A. Archakov, Biosens. Bioelectron., 2010, 25, 1694–1698 CrossRef CAS PubMed.
- P. Mukherjee, R. Bhattacharya, P. Wang, L. Wang, S. Basu, J. A. Nagy, A. Atala, D. Mukhopadhyay and S. Soker, Clin. Cancer Res., 2005, 11, 3530–3534 CrossRef CAS PubMed.
- S. Sheikpranbabu, K. Kalishwaralal, K. Lee, R. Vaidyanathan, S. H. Eom and S. Gurunathan, Biomaterials, 2010, 31, 2260–2271 CrossRef CAS PubMed.
- J. Baharara, F. Namvar, M. Mousavi, T. Ramezani and R. Mohamad, Molecules, 2014, 19, 13498–13508 CrossRef PubMed.
- J. Zhang and Y. Zhu, Microporous Mesoporous Mater., 2014, 197, 244–251 CrossRef CAS.
- R. Benzaid, J. Chevalier, M. Saâdaoui, G. Fantozzi, M. Nawa, L. A. Diaz and R. Torrecillas, Biomaterials, 2008, 29, 3636–3641 CrossRef CAS PubMed.
- J. M. Guerreiro-Tanomaru, A. Trindade-Junior, B. Cesar Costa, G. F. da Silva, L. Drullis Cifali, M. I. Basso Bernardi and M. Tanomaru-Filho, Sci. World J, 2014, 2014, 1–6 CrossRef PubMed.
- S. Garg, A. Chandra, A. Mazumder and R. Mazumder, Asian J. Pharm., 2014, 8, 95 CrossRef CAS.
- G. Muhammad, M. A. Hussain, M. Amin, S. Z. Hussain, I. Hussain, S. N. Abbas Bukhari and M. Naeem-ul-Hassan, RSC Adv., 2017, 7, 42900–42908 RSC.
- Z. Lu, J. Gao, Q. He, J. Wu, D. Liang, H. Yang and R. Chen, Carbohydr. Polym., 2017, 156, 460–469 CrossRef CAS PubMed.
- D. M. Morens, G. K. Folkers and A. S. Fauci, Nature, 2004, 430, 242–249 CrossRef CAS PubMed.
- J. Frenk, O. Gómez-Dantés and F. M. Knaul, Infect. Dis. Clin., 2011, 25, 593–599 CrossRef PubMed.
- G. Ibrahim Fouad, Bull. Natl. Res. Cent., 2021, 45, 36 CrossRef PubMed.
- K. E. Ukhurebor, K. R. R. Singh, V. Nayak and G. UK-Eghonghon, Environ. Sci.: Process. Impacts, 2021 10.1039/D1EM00154J.
- S. Perlman and J. Netland, Nat. Rev. Microbiol., 2009, 7, 439–450 CrossRef CAS PubMed.
- S. M. El-Megharbel, M. Alsawat, F. A. Al-Salmi and R. Z. Hamza, Coatings, 2021, 11, 388 CrossRef CAS.
- C. Kundu, India Today, 2020 Search PubMed https://www.indiatoday.in/fact-check/story/has-covid19-lockdown-returned-dolphins-swans-italian-waterways-1658457-2020-03-22.
- S. Luo, C. Ma, M.-Q. Zhu, W.-N. Ju, Y. Yang and X. Wang, Front. Cell. Neurosci., 2020, 14 DOI:10.3389/fncel.2020.00021.
- A. F. A. Itani and M. Tobaiqy, Theranostics, 2020, 10, 5932–5942 CrossRef PubMed.
- V. S. Madamsetty, A. Mukherjee and S. Mukherjee, Front. Pharmacol., 2019, 10 DOI:10.3389/fphar.2019.01264.
- M. Ovais, A. Khalil, M. Ayaz, I. Ahmad, S. Nethi and S. Mukherjee, Int. J. Mol. Sci., 2018, 19, 4100 CrossRef PubMed.
- L. Ma, J. Liu, W. Su, X. Zeng, X. Liu, W. Li, J. Deng and J. Tang, J. Nanosci. Nanotechnol., 2018, 18, 8133–8141 CrossRef CAS PubMed.
- S. K. Vemuri, R. R. Banala, S. Mukherjee, P. Uppula, G. P. V. Subbaiah, A. V. Gurava Reddy and T. Malarvilli, Mater. Sci. Eng. C, 2019, 99, 417–429 CrossRef CAS PubMed.
- Z. Chen, Z. Zhang, X. Zhai, Y. Li, L. Lin, H. Zhao, L. Bian, P. Li, L. Yu, Y. Wu and G. Lin, Anal. Chem., 2020, 92, 7226–7231 CrossRef CAS PubMed.
- M. Chakravarty and A. Vora, Drug Deliv. Transl. Res., 2021, 11(3), 748–787 CrossRef CAS PubMed.
- B. Udugama, P. Kadhiresan, H. N. Kozlowski, A. Malekjahani, M. Osborne, V. Y. C. Li, H. Chen, S. Mubareka, J. B. Gubbay and W. C. W. Chan, ACS Nano, 2020, 14, 3822–3835 CrossRef CAS PubMed.
- A. D. Chintagunta, M. Sai Krishna, S. Nalluru and N. S. Sampath Kumar, Emergent Mater., 2021, 4, 119–130 CrossRef CAS PubMed.
- M. C. Sportelli, M. Izzi, E. A. Kukushkina, S. I. Hossain, R. A. Picca, N. Ditaranto and N. Cioffi, Nanomaterials, 2020, 10, 802 CrossRef CAS PubMed.
- N. van Doremalen, T. Bushmaker, D. H. Morris, M. G. Holbrook, A. Gamble, B. N. Williamson, A. Tamin, J. L. Harcourt, N. J. Thornburg, S. I. Gerber, J. O. Lloyd-Smith, E. de Wit and V. J. Munster, N. Engl. J. Med., 2020, 382, 1564–1567 CrossRef PubMed.
- Y. Abo-zeid, N. S. M. Ismail, G. R. McLean and N. M. Hamdy, Eur. J. Pharm. Sci., 2020, 153, 105465 CrossRef CAS PubMed.
|
This journal is © The Royal Society of Chemistry 2021 |
Click here to see how this site uses Cookies. View our privacy policy here.