DOI:
10.1039/D1RA07821F
(Paper)
RSC Adv., 2021,
11, 38961-38976
Retracted Article: Enhanced antimicrobial treatment by a clay-based drug nanocarrier conjugated to a guanidine-rich cell penetrating peptide†
Received
22nd October 2021
, Accepted 29th November 2021
First published on 6th December 2021
Abstract
In this study, a novel and efficient drug delivery system is proposed for the enhancement of antimicrobial properties of antibiotic medications such as vancomycin (VCM) and levofloxacin (OFX). The architecture of the designed drug carrier is based on halloysite nanotubes (HNTs) with a rolled-laminate shape, suitable for the encapsulation of drug and further release. In order to make them capable for magnetic direction to the target tissue, the exterior surface of the tubes is composed of iron oxide nanoparticles (Fe3O4 NPs), via an in situ process. The main role in the antimicrobial activity enhancement is played by a cell-penetrating peptide (CPP) sequence synthesized in the solid phase, which contains three arginine–tryptophan blocks plus a cysteine as the terminal amino acid (C(WR)3). The drug content values for the prepared nanocargoes named as VCM@Fe3O4/HNT–C(WR)3 and OFX@Fe3O4/HNT–C(WR)3, have been estimated at ca. 10 wt% and 12 wt%, respectively. Also, the drug release investigations have shown that above 90% of the encapsulated drug is released in acetate buffer (pH = 4.6), during a 90 minutes process. Confocal microscopy has corroborated good adhesion and co-localization of the particles and the stained living cells. Moreover, in vitro antimicrobial assessments (optical density, zone of inhibition, and minimum inhibitory concentration) have revealed that the bacterial cell growth rate is significantly inhibited by suggested nanocargoes, in comparison with the individual drugs in the same dosage. Hence, administration of the presented nanocargoes is recommended for the clinical treatment of the infected target organ.
1. Introduction
One of the permanent challenges in the administration of antibiotic compounds is resistance of bacteria to the anti-infection drugs.1 For instance, an acquired bacterial resistance to antibiotic vancomycin (called vancomycin-resistant enterococci) is a well-known type in Enterococcus bacterium caused by alteration of the peptidoglycan synthetic pathway.2 As another example, bacterial resistance to beta-lactam antibiotics such as penicillin and cephalosporin via secretion of metallo-beta lactamase enzymes can be mentioned.3 As a traditional resolution for this issue, co-administration of the antibiotic drug with an assistant has been suggested (like co-amoxiclave).4 Today, with the advent of nanotechnology, the therapeutic effects of the useless medications have been revived. Briefly, tiny size drug carriers, surface-functionalization with the various protein facilitators, and enhanced internalization into the cells led to reuse the abandoned drugs.5–7 In fact, via encapsulation of the aimed drug into the nanostructures, destructive influences of the bacteria on the antibiotic drugs that lead to deactivation, is decreased.8,9 All of the mentioned properties of the nanoscale drug delivery systems help to overcome the bacterial resistance, as well. In this regard, Huh and Kwon have published a review on the advances in treatment of the bacterial infections by the nanomaterials as a new paradigm known as “nanoantibiotics”.10 Moreover, Cardoso and co-workers have reported a nanoscale therapeutic containing tetracycline antibiotic into silica nanoparticles to overcome the bacterial resistances.11
In addition to bacterial resistance, the use of nanomaterials also affects drug release, cell internalization, and dose control strategies.12 Briefly, using the porous nanomaterials or polymeric matrices in the composite form provides this great opportunity to control the drug release process in the target cells; for example extended release by the layered structures,13 and controlled release in a specific condition through using pH/temperature responsive polymers.14 Among different types of the nanomaterials, halloysite which is a clay-based species with the rolled tubular structure has shown interesting properties in the recent therapeutic substances.15,16 As the first advantage, a layer-by-layer paradigm can be executed for the loading and subsequent release process of the aimed drug.17 Through using the halloysite nanotubes (HNTs), a determined dosage of the drug is wrapped and delivered to the target cells.18 Moreover, there is a strong hydrogen binding (H-bind) network between the layers of the HNTs resulting in the formation of the stable cages for encapsulation of the drugs. This H-bind network origins from the silanol and aluminol groups onto the exterior and interior surfaces of the rolled wall, respectively.19 Also, the hydroxyl (–OH) groups located onto the surfaces of the HNTs act as the suitable sites for chemical conjugations.20
As another useful species of the nanomaterials, iron oxide nanoparticles (Fe3O4 NPs) has been widely used in different scopes such as drug delivery,21,22 catalysis,23,24 diagnosis,25 medical imaging,26 sensing,27 removal of the pollutants,28 and etc.29,30 The first and foremost excellence of the Fe3O4 NPs is its impressive magnetic property which leads to extensive use in different applications.31,32 In the field of drug delivery, magnetic behavior of the nano-carriers provides high convenience in both preparation and application processes. The conduction of the magnetic cargoes to the target tissue through applying an external magnetic field has been introduced as an efficient strategy for the enhancement of the targeted delivery.33 Moreover, Fe3O4 NPs with the mean size of 35 nm provide an extreme surface area due to having a huge surface to volume ratio.34,35 Also, the surface of the Fe3O4 NPs could be chemically functionalized due to the presence of the –OH groups.36,37 Magnetization of the different species through combination with the Fe3O4 NPs via incorporation into the pores or H-bind interactions, is a common practice.38–40
Another potential resolution for the bacterial resistance in the antimicrobial therapy is submitted based on the use of antimicrobial peptides (AMPs).41,42 For instance, peptide nucleic acids (PNAs) as synthetic analogs of DNA with 2-([2-aminoethyl]amino)acetic acid backbone have been used due to their inhibitory effect on transcription and translation processes. The PNAs are able to complementary bind to DNA/RNA with high selectivity. Therefore, they have been widely used in diagnosis and treatment of various diseases.43,44 As another species of AMPs, cell-penetrating peptides (CPPs) have been recognized as the active structures in cellular uptake process. Concisely, through electrostatic interactions between the positive-charged amino acids and negative-charged ingredients in the cell membrane, cell adhesion and subsequent penetration are enhanced by the AMPs.45 Typically, arginine (Arg) is used for this purpose due to containing a guanidine functional group at the end of the side chain.46 As a reasonable justification, since the guanidine group includes more positive-charged nitrogen centers in its chemical structure (HNC(NH2)2), the electrostatic interactions with the phosphate and sulphate ingredients (present in cell membrane), are enhanced.47 So far, so many reports have been published about the AMP–drug conjugations, in which an antimicrobial drug is covalently linked to an AMP, resulted in the enhanced anti-infection properties.48–50
Based on the advantages described above, herein, we suggest a novel tiny-sized system constructed of the HNTs decorated with the Fe3O4 NPs onto the surfaces, utilizable for magnetic drug delivery in the antimicrobial treatments. To overcome the bacterial resistance, the antibiotic drugs are encapsulated inside and between the rolled layers of the tubes, and then well wrapped via freeze drying. For this purpose, vancomycin (VCM) and ofloxacin (OFX) as two mostly administrated medications for the bacterial infections (specifically diarrhea), have been used. In order to demonstrate the synergistic therapeutic effects between the encapsulated drugs and the AMPs, the surfaces of the VCM@Fe3O4/HNT and OFX@Fe3O4/HNT particles are covalently conjugated to a guanidine-rich peptide chain, synthesized on a solid resin. The structure of the target peptide chain includes an initial cysteine (C) plus three repeated blocks of tryptophan–arginine (WR). Finally, the antimicrobial properties of the prepared VCM@Fe3O4/HNT–C(WR)3 and OFX@Fe3O4/HNT–C(WR)3 therapeutics have been evaluated by the optical density experiment at the wavelength of 600 nm (OD600), zone of inhibition (ZOI), and disk counter experiments on Staphylococcus aureus (S. aureus) and Escherichia coli (E. coli) as Gram-positive and Gram-negative bacteria cell lines, respectively. Briefly, it has been observed that the growth rate of the bacterial cells is inhibited for above 70% by VCM@Fe3O4/HNT–C(WR)3 and OFX@Fe3O4/HNT–C(WR)3 nanocargoes, during a 90 minutes process. Also, minimum inhibitory concentration (MIC) and minimum bactericidal concentration (MBC) values have been estimated for the prepared nanocargoes and compared with the individual VCM and OFX. The obtained results have exhibited significant enhancement of the antimicrobial effect through incorporation of the low dosages of VCM and OFX drugs into the Fe3O4/HNT–C(WR)3 nano-carrier.
2. Experimental
2.1 Materials and equipment
All chemical and instruments used in this project are listed in Tables S1 and S2, in ESI file.†
2.2 Preparation and synthetic methods
2.2.1 Preparation of the activated HNTs. HNTs powder (5.0 g) was ground via ball-milling (50 Hz) for 30 min, using zirconia grinding bowls and balls of 0.1 mm in diameter. Then, the calcination process was performed on the ground particles via a programmed heating up to 600 °C, during 4 h in the furnace. After cooling down to room temperature, the obtained neat powder of the HNTs was transferred into a sterilized glass flask, then N2 gas was purged and the flask was well sealed. Further, 2.0 g of the neat HNTs was placed into a round-bottom flask (100 mL) and 50 mL of hydrochloric acid (HCl, 1.0 M) was added into the flask. To obtain a fine dispersion of the HNTs in the acid medium, ultrasound bath (50 Hz, 100 W L−1) was used, for 30 min at room temperature. Afterward, the mixture was stirred for 24 h, at 80 °C under reflux conditions. Next, the activated HNTs were collected via centrifugation (4000 rpm, 15 min), and rinsed with deionized water for three times. Finally, the obtained powder was dried in the vacuum oven and stored in a sterilized glass flask, under N2 gas.51
2.2.2 Preparation of Fe3O4 NPs. In a round-bottom flask (100 mL), FeCl2·4H2O (1.0 mmol) and FeCl3·6H2O (1.3 mmol) were dissolved in deionized water (30 mL) and stirred at 80 °C, under N2 atmosphere for 2 h. Then, a solution of NH3·H2O (15 mL, 25 wt%) was dropwise added into the flask during the stirring. Then the reaction mixture was cooled down to room temperature and the obtained magnetic NPs were magnetically collected and washed for several times with deionized water. Finally, they were dried in oven at 60 °C.52
2.2.3 In situ composition of Fe3O4 NPs with HNTs. For this purpose, the same procedure as the above section was performed with this difference that the neat HNTs (0.5 g) were dispersed in the solution of the iron salts, before addition of the NH3·H2O solution.53
2.2.4 Functionalization of Fe3O4/HNTs nanocomposite with mercaptopropyl silane (MPS). In a double-necked round-bottom flask (50 mL), Fe3O4/HNTs particles (0.5 g) were dispersed in toluene (10 mL) via ultrasonication. Then, the mixture was stirred under N2 atmosphere at room temperature, and 3-mercaptopropyl trimethoxysilane (MPTMS) (7.0 mL) was added to the flask drop-by-drop using a glass syringe fitted in septum. Next, the mixture was stirred for 12 h under N2 atmosphere, under reflux conditions (105 °C). Afterward, the obtained Fe3O4/HNT–MPS particles were magnetically collected and washed with hot ethanol (10 mL) for several times. Ultimately, the particles were dried in oven at 60 °C.53,54
2.2.5 Incorporation of the VCM and OFX in nano-carriers. In four separate conical tubes (15 mL, Falcon Centrifuge Tubes, polypropylene), the aqueous solutions of VCM and OFX (5.0 mL, 20 mg mL−1) were placed and then Fe3O4/HNT–MPS particles (0.1 g) were dispersed in the tubes via ultrasonication (50 Hz, 5 min), at room temperature. Next, the tubes were sealed and covered by the foil, and the content of the tubes were shaken for 24 h. After completion of the process, the obtained nanocargoes (VCM@Fe3O4/HNT–MPS and OFX@Fe3O4/HNT–MPS) were separated via centrifugation (4000 rpm, 15 min), and washed for two times with deionized water, via vortex mixing and centrifugation. Finally, the obtained particles were redispersed in the deionized water, transferred to the glass Petri dishes, and dried by freeze drier, for 48 h.55
2.2.6 Synthesis of the C(WR)3 peptide chain. The C(WR)3 peptide sequence was synthesized in the solid phase; where 2-chlorotrityl chloride (CTC, loading 0.8, 0.5 g) was well washed with DMF (10 mL) via shaking, swollen in DCM (10 mL), and dried by the vacuum pump. Then, the first amino acid (Fmoc–Trp(Trt)–OH, 1.0 mmol) was dissolved in DMF (10 mL) and DIEA (8.0 mmol). Then, the prepared mixture was added to the swollen CTC placed in a glass vessel, and shaken for 2 h, at room temperature. Afterward, removal of the Fmoc protecting group was carried out with piperidine solution (25% in DMF). Further, next amino acid (Fmoc–Arg(Pbf)–OH) was activated by dissolving in a solution of DMF (10 mL), 2-(1H-benzotriazole-1-yl)-1,1,3,3-tetramethylaminium tetrafluoroborate (TBTU, 1.3 mmol), and N,N-diisopropylethylamine (DIPEA, 0.8 mL). The obtained solution was then added into the vessel and shaken for 2 h, at room temperature. This process was continued for the next amino acids, and after completion of the process (attachment of Fmoc–Cys(Trt)–OH), the synthesized peptide chain was separated from the CTC resin by a solution of TFA 96%, TES 2.0% and deionized water 2.0%. Then, the CTC resin was removed via filtration, and TFA was evaporated by rotary evaporator, under reduced pressure. Next, cold diethyl ether was added to form a white powder, and the mixture was stirred in an ice bath, for 1 h. Finally, the white powder was obtained through paper filtration.55
2.2.7 Preparation of VCM@Fe3O4/HNT–C(WR)3 and OFX@Fe3O4/HNT–C(WR)3 nanocargoes. For disulfide bond conjugation, after dispersion of 0.1 g of the VCM@Fe3O4/HNT–MPS or OFX@Fe3O4/HNT–MPS particles in 3.0 mL of cold deionized water (4 °C), H2O2 (30 wt%, 0.2 mL) and Cys-containing peptide chain (3.0 mL, 0.5 M, in ethanol) were added to the mixture drop-by-drop using two separate syringes, during the shaking for 1.5 h.56 After completion of the process, the obtained VCM@Fe3O4/HNT–C(WR)3 and OFX@Fe3O4/HNT–C(WR)3 nanocargoes were separated via centrifugation (4000 rpm, 15 min), and washed for two times with deionized water, via vortex mixing and centrifugation. Finally, the particles were redispersed in the deionized water, transferred to the glass Petri dishes, and dried by freeze drier, for 48 h.
2.3 Confocal microscopy
Initially, all glassware were sterilized with autoclave, at 120 °C. In a glass tube with a threaded cap, 0.05 mg of VCM@Fe3O4/HNT–C(WR)3 particles was dispersed in 1.0 mL of Dulbecco's modified Eagle's medium (DMEM), and the bacterial cells (S. aureus-ATCC 12600 or E. coli-ATCC 9637) with the density of 3 × 108 CFU mL−1 were added. The content of the tube was incubated at 37 °C, at 95% humidity. After 90 min incubation, cell staining was performed using three drops of diluted crystal violet in phosphate buffer saline (PBS, 0.1 M), and a drop of Lugol's solution. Then, 30 μL of the sample was poured on a laminated glass slide and dried.55
2.4 Antimicrobial experiments
2.4.1 Cell cultivation. For cultivation, S. aureus-ATCC 12600 and E. coli-ATCC 9637 cells were incubated in the LB broth medium with density value of 106–107 CFU mL−1. Cultivation was done in the sterilized glassware at 37 °C and 95% humidity. Incubation was carried out in a shaking incubator with 180 rpm.55
2.4.2 Optical density experiments. Cell cultures were carried out in the presence of the VCM@Fe3O4/HNT–C(WR)3 and OFX@Fe3O4/HNT–C(WR)3 particles (50 μg mL−1, in DMEM), dispersed via ultrasonication (50 kHz, 100 W L−1) for 2 min. A dispersion of related particles was used as blank control. Also, culture at particle-free medium was used as a control. More dispersions including Fe3O4/HNTs, Fe3O4/HNT–C(WR)3, and also individual C(WR)3 peptide sequence, VCM and OFX with the same concentration as the main samples were assessed. Three samples for each condition were prepared in a 96-well plate. Ultimately, the prepared samples were studied by a UV-vis spectroscopy at 30, 60, and 90 min.55
2.4.3 Zone of inhibition experiments. Initially, appropriate dispersions of the solid particle samples including VCM@Fe3O4/HNT–C(WR)3, OFX@Fe3O4/HNT–C(WR)3, HNTs, Fe3O4/HNTs, and Fe3O4/HNT–C(WR)3 were prepared in DMEM medium, with the concentration of 10 mg mL−1. Also, the individual VCM, OFX, and C(WR)3 peptide sequence were dissolved in DMEM with the same concentration as the particles, and the sterilized disks were used for the subjection to the cells. Cultivation was performed in the sterilized Petri dishes containing the LB broth liquid medium and the bacterial cells, via incubation at 37 °C for 24 h. The formed zones of inhibition were evaluated and reported in centimeter unit. Three samples for each compound were prepared and studied to check reproducibility of results.55
3. Results and discussion
3.1 Preparation of VCM@Fe3O4/HNT–C(WR)3 and OFX@Fe3O4/HNT–C(WR)3 nanocargoes
Initially, to prepare a uniform collection of the tubes, HNTs were ground by ball-milling. Then, to remove the unwanted fillers, calcination was performed at high temperatures. Next, in order to activate silanol and aluminol groups, treatment of the tubes with HCl was carried out.35,51 To magnetize the tubes, co-deposition of the iron(II) and (III) chloride salts in an alkaline condition was performed through an in situ manner, resulting in F3O4/HNTs nanocomposite. For this purpose, the HNTs were initially well dispersed via ultrasonication.57 To functionalize the surface of the F3O4/HNTs, mercapto silane compound were used. Due to the presence of the propyl groups in the structure of mercapto propyl silane (MPS), the hydrophobicity of the surfaces is a bit increased (video file no. 1), which is appropriate for prevention of drug leaching. In this stage, the surface of the particles are covalently functionalized through silicon–oxygen bonds.58–60 In the next stage, the silanized-Fe3O4/HNTs nanoparticles were dispersed in the concentrated solutions of VCM and OFX drugs, and then shaken in the darkness. Light protection was preformed via insulation of the reaction flask with the pieces of foil, as ordered in the analytical papers of the VCM and OFX for preservation. On the other hand, C(WR)3 peptide sequence was synthesized using solid-phase peptide synthesis techniques, described in literature.55 For disulfide bond conjugation between the F3O4/HNT–MPS particles and C(WR)3 peptide sequence, a cold solution of hydrogen peroxide was used.56 Scheme 1 presents the successive stages of the preparation route of VCM@Fe3O4/HNT–C(WR)3 and OFX@Fe3O4/HNT–C(WR)3 nanocargoes.
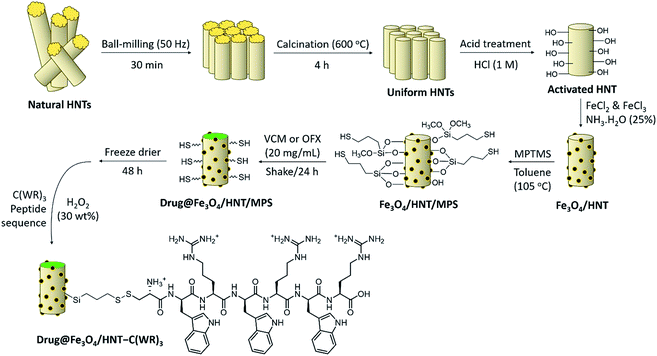 |
| Scheme 1 Schematic presentation of the successive stages of the preparation route of VCM@Fe3O4/HNT–C(WR)3 and OFX@Fe3O4/HNT–C(WR)3 nanocargoes. | |
The C(WR)3 peptide sequence was synthesized on CTC resin, respect to the approved procedures for solid-phase peptide synthesis. Briefly, CTC seeds were well washed with DMF, and then swollen in DCM. Shaking and drying by the vacuum pump have been used within the successive stages of the synthesis. Fmoc–Arg(pbf)–OH was dissolved in DMF and DIEA, and the prepared solution was added to the swollen CTC placed in a glass vessel, and shook. Afterward, removing of the Fmoc-protecting group was carried out using piperidine solution (25% in DMF). Next, Fmoc–Trp(boc)–OH was dissolved in a mixture of DMF, TBTU (as a coupling reagent), and N,N-diisopropylethylamine (DIPEA), and then added into the vessel and shook. This process was repeated for the next amino acids until three successive blocks of arginine–tryptophan (RW) was obtained. As the last amino acid, Fmoc–Cys(Trt)–OH was added to the sequence. Finally, the synthesized peptide sequence (C(WR)3) was separated from the resin using a mixture of TFA (96%), triethyl silane (TES, 2.0%), and deionized water (2.0%). The synthesized peptide sequence was identified by liquid chromatography-mass spectrometry (LC-MS) (Fig. S1, in ESI section†).
3.2 Characterization of VCM@Fe3O4/HNT–C(WR)3 and OFX@Fe3O4/HNT–C(WR)3 nanocargoes
3.2.1 FTIR spectroscopy. As the first method for characterization of the products, Fourier-transform infrared (FTIR) spectroscopy was used to investigate functionalization of the HNT cargo after successive stages of the preparation. As is observed in Fig. 1, successful formation of the Fe3O4 NPs is confirmed by two indicative peaks; one at ca. 580 cm−1 related to Fe–O bond, and another one at 3000–3500 cm−1 belonging to the hydroxyl groups (O–H) present on the surface of the particles (spectrum 1).61 HNTs are generally characterized by a sharp peak at ca. 1100 cm−1, which is related to the stretching vibrations of Si–O–Si bands in the silica network. The broad peak of the hydroxyl groups (silanol and aluminol) is also appeared at 3100–3600 cm−1 (spectrum 2).62 In the same spectrum, there are also some other peaks with weak intensity appeared at ca. 1635, 1750, 2960, and 2990 cm−1, which are attributed to the unwanted materials inside the tubes. In spectrum 3 that belongs to the ground HNTs, this is clearly seen that the similar peaks are appeared at the same areas, confirming well preservation of the silica network during the ball-milling process. After acid treatment of the HNTs (spectrum 4), two separate peaks have been appeared at ca. 3624 and 3695 cm−1 that are attributed to the stretching vibrations of AlO–H bonds, present onto the interior surface of the rolled walls of HNTs.63 Activation of these hydroxyl groups before encapsulation of drug into the tubes is of high importance, since they play a key role in the formation of the H-bond network between the walls and wrapping the cargo.64 In spectrum 5 that is related to the magnetized HNTs, this is observed that a broad peak has been added in the area of 3000–3600 cm−1, corroborating the presence of the hydroxyl groups of Fe3O4 NPs. Moreover, the sharp peak of Fe–O bond at ca. 580 cm−1 has been added to the spectrum, verifying well composition of Fe3O4 NPs with the HNTs. Furthermore, appearance of a weak peak at ca. 2972 cm−1 verifies the presence of the C–H bonds with hybridation sp3, coming from mercaptopropyl silane (MPS). Finally, in spectrum 6 belonging to VCM@Fe3O4/HNT–C(WR)3 nanocargo, a tangible increase in the peak intensity of C–Hsp3 at ca. 2959 cm−1, and also appearance of the new peaks in a range of 1600–1800 cm−1 coming from carbonyl groups in the structure of VCM and C(WR)3 peptide sequence, clearly corroborate successful addition of the VCM and C(WR)3 to the composite.
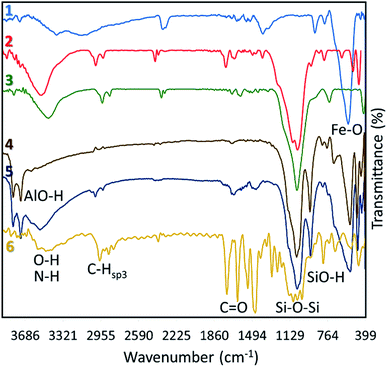 |
| Fig. 1 FTIR spectra of (1) Fe3O4 NPs, (2) natural HNTs, (3) ground HNTs, (4) activated HNTs, (5) Fe3O4/HNTs–MPS nanocomposite, and (6) VCM@Fe3O4/HNT–C(WR)3 nanocargo. | |
3.2.2 EDX and XRD spectroscopy. One of the mostly used methods for investigation of the new ingredients added to the nanostructures, is energy-dispersive X-ray (EDX) analysis. Via this method, the presence of the expected elements with the related quantities in the samples is confirmed. Fig. S2 (in ESI section†) illustrates the obtained EDX spectra of eight samples including natural, calcinated, and acid-activated HNTs, magnetized HNTs with Fe3O4 NPs, MPS-functionalized Fe3O4/HNT, VCM@Fe3O4/HNT–MPS, VCM@Fe3O4/HNT–C(WR)3 and OFX@Fe3O4/HNT–C(WR)3 composite structures. As is seen, all essential elements exist in the spectra of each sample, corroborating successful combination of the materials. From a comparison between the obtained spectra for the individual HNTs, this is quickly found out that a cleaner structure has been resulted after the calcination and acid-treatment processes. As shown, only two peaks are observed in the EDX spectrum of activated HNTs, verifying successful removal of the unwanted materials such as calcium, sodium, and carbon. Moreover, increase in the peak intensity correlates with the abundance of the elements. As presented by quantitative results tables, weight percentage of carbon and nitrogen atoms has raised after conjugation of the Fe3O4/HNTs to the C(WR)3 peptide structure. Also, the peaks related to chlorine and fluorine elements have emerged at the areas of ca. 2.6 and 0.6 keV, after incorporation of VCM and OFX in the tubes, respectively.For further confirmation of successful composition of Fe3O4 NPs and HNTs, X-ray diffraction (XRD) patterns were provided and compared together. As demonstrated in Fig. 2, all reference peaks related to the structure of Fe3O4 NPs (JCPDS no. 19-0629) have been appeared in the pattern of Fe3O4/HNTs, corroborating well combination of the spherical particles with the nanotubes. These indicative peaks have appeared at 2θ = 31.1°, 35.4°, 43.3°, 53.5°, 57.3°, and 62.6°. Also, in the pattern of Fe3O4/HNTs composite, there are two peaks at 2θ = 20.2° and 23.8° marked with Miller indices (0 2 0) and (0 0 2), respectively, which verify the presence of HNTs (JCPDS no. 29-1489).
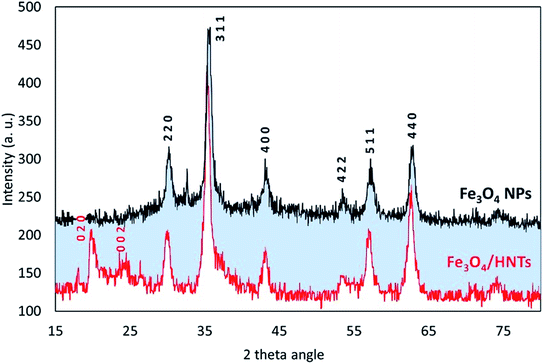 |
| Fig. 2 XRD patterns of Fe3O4 NPs and Fe3O4/HNTs composite. | |
3.2.3 TGA analysis. Thermogravimetric analysis (TGA) was performed on the samples to investigate decomposition states of the used ingredients via thermal degradation. From a quick look at Fig. 3a, the structural differences between Fe3O4/HNTs, VCM@Fe3O4/HNT–C(WR)3, and OFX@Fe3O4/HNT–C(WR)3 composites are found out. In all three curves, a partial increase in the weight ratio has occurred at the beginning of study. This increase is most likely coming from the adsorption of the moisture in the air by hot surfaces of the particles. For Fe3O4/HNTs composite, almost a gradual descending trend is observed until 800 °C, in which ca. 8% of the weight has been lost. This amount of weight loss is attributed to the removal of the water molecules from the structure at the early stages of degradation. At higher temperatures, the captured water by the underlying layers (especially alumina and silica networks) are separated.65 For the VCM@Fe3O4/HNT–C(WR)3 and OFX@Fe3O4/HNT–C(WR)3 structures, this is clearly observed that more values of the weight loss occurred during thermal degradation process in a range of 50–800 °C. For VCM@Fe3O4/HNT–C(WR)3 and OFX@Fe3O4/HNT–C(WR)3 structures, ca. 4% and 3% of the weight have been lost, respectively, due to separation of the water molecules. Then, the weight ratios of both samples gradually reduced to ca. 90% and 86% by increase in the temperature to around 580 °C, for VCM@Fe3O4/HNT–C(WR)3 and OFX@Fe3O4/HNT–C(WR)3, respectively. At this stage, the organic structures including C(WR)3 and parts of the encapsulated VCM and OFX drugs are decomposed.66 Afterward, the rolled structure of HNTs are probably dissociated and the combined Fe3O4 NPs start to degradation. Simultaneously, the entrapped VCM and OFX inside the underlying layers of the rolled tubes start to release and decomposition.
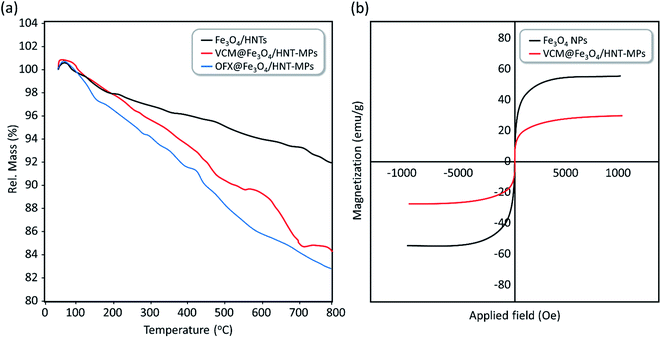 |
| Fig. 3 (a) TGA curves of Fe3O4/HNTs, VCM@Fe3O4/HNT–C(WR)3, and OFX@Fe3O4/HNT–C(WR)3, composites, under air atmosphere. (b) Room-temperature magnetic-hysteresis curves of Fe3O4 NPs and VCM@Fe3O4/HNT–C(WR)3 composite. | |
3.2.4 VSM analysis. To investigate magnetic properties of the formulated nanocargoes, vibrating-sample magnetometer (VSM) analysis was used. As shown in Fig. 3b, magnetization saturation of the neat Fe3O4 NPs occurs at 57 emu g−1, through application of a 10 K Oe magnetic field. Whereas, this value reduced to ca. 22 emu g−1 for VCM@Fe3O4/HNT–C(WR)3 composite. Obviously, since other combined components such as HNTs, VCM, and C(WR)3 are not magnetic, the magnetic property of the final product is much less than the neat Fe3O4 NPs. However, this value of magnetization saturation is adequate for execution of the magnetic direction of nanocargoes in the body.67
3.2.5 SEM/TEM imaging. To investigate size distribution and composition states of the formed particles, scanning-electron and transmission-electron microscopy (SEM and TEM) were employed. As illustrated in Fig. 4a, the neat Fe3O4 NPs with spherical morphology and average size of ca. 42 nm have been successfully synthesized. As is seen in the prepared SEM image, agglomeration of the particles is observed in some areas that is most likely due to super-paramagnetic property of the particles. Next, the tubular structure of the natural HNTs is recognized in Fig. 4b. Also, the existence of the excess materials with the HNTs is clearly verified in the prepared SEM image (b). As described in the preparation section, to make a uniform and clean collection of the HNTs, grinding by ball-milling was performed. As shown in Fig. 4c, well uniformity in size and shape of the HNTs has been obtained after the grinding process. Generally, estimation of the mean size of the particles is carried out via two ways; first, high-resolution (HR) TEM imaging and selected area (electron) diffraction (SAED) are performed on the sample.68,69 Second, a zoomed-out image is selected and imported into Digimizer software, then the size of the particles that are distinguishable by eyes is estimated.70 In this work, we used the second method and selected an area for evaluation of size distribution of for the neat HNTs (green box in image c). The resulted size distribution diagram has been given in Fig. 4i, presenting the mean size of the neat HNTs around 1600–2100 nm before the grinding process. To corroborate the efficiency of the grinding stage, size distribution state of a suspension of HNTs in the aqueous medium has been investigated by Zetasizer analysis. Fig. S3 (in the ESI section†) demonstrates the obtained intensity-based dynamic-light scattering (DLS) curves related to the HNTs suspension samples before and after grinding process, confirming breakage of the tubes into smaller pieces. The average size of the HNTs has been estimated to be ca. 400 nm, after the grinding process. Obviously, the mean size of the particles in the colloidal form is less than the solid state, since irradiation of the ultrasound waves is used for dispersion of the particles in the liquid medium. Fig. 4d illustrates the neat structure of the HNTs after acid treatment and grinding processes. Fig. 4e and f confirms successful composition of the Fe3O4 NPs with the HNTs. Fig. 4g presents the TEM image of the synthesized Fe3O4 NPs, in which dark spots come from the spherical nanoparticles. In Fig. 4h, incorporation of the Fe3O4 NPs onto the exterior surfaces of the HNTs in the final structure of VCM@Fe3O4/HNT–C(WR)3 is verified. In this image, the presence of the dark spots onto the surface of the tubes well corroborate combination of the particles with the tubes.
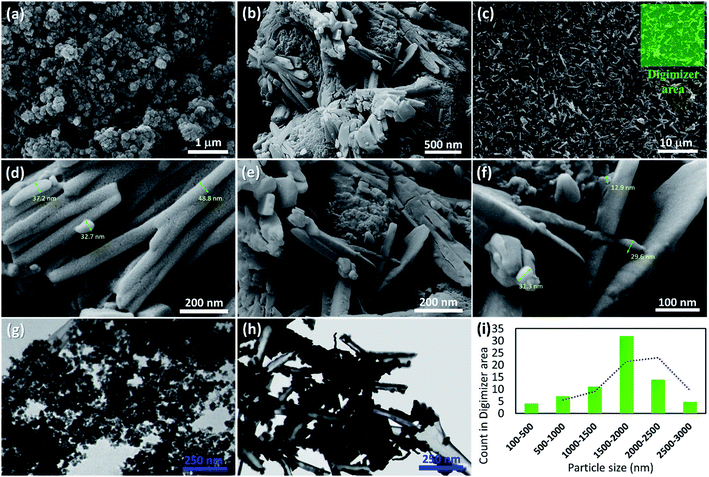 |
| Fig. 4 SEM images of (a) Fe3O4 NPs, (b) natural HNTs, (c) acid-treated HNTs, (d) ground HNTs, and (e and f) Fe3O4/HNTs composite. TEM images of (g) Fe3O4 NPs, and (h) VCM@Fe3O4/HNT–C(WR)3 composite. (i) Size-distribution diagram of acid-treated HNTs, related to the green box in the image (c). | |
3.2.6 UV-DRS spectroscopy. Fig. 5 shows UV-vis differential reflectance spectroscopy (UV-DRS) curves related to neat HNTs, individual VCM, VCM@HNT, and VCM@Fe3O4/HNT composites, prepared for comparison and investigation of drug loading into the tubes. All samples were well rinsed with deionized water and ethanol for several times to remove any excess compounds from the surfaces. As is observed, the maximum values of the UV-vis reflectance activity of the neat HNTs (blue line) and individual VCM (violet line) have been obtained at ca. 225–325 and 235 nm, respectively. From the curve of VCM@HNT (gray line), it is concluded that the VCM has been successfully incorporated into the tubes, as it shows reflectance activity in a wavelength range of ca. 220–330 nm. In the curve related to VCM@Fe3O4/HNT composite (red line), this is seen that an additional sharp shoulder has been added in the area of 335–425 nm. This peak is attributed to the Fe3O4 NPs that have been incorporated into the composite structure.71
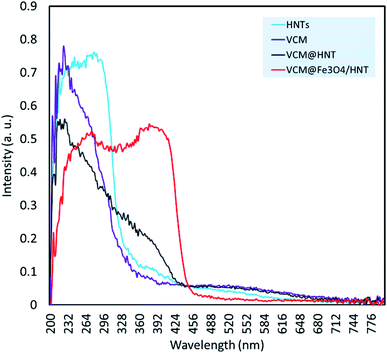 |
| Fig. 5 UV-DRS curves of the neat HNTs, individual VCM, VCM@HNT, and VCM@Fe3O4/HNT composites. | |
3.3 Therapeutic properties of VCM@Fe3O4/HNT–C(WR)3 and OFX@Fe3O4/HNT–C(WR)3 nanocargoes
3.3.1 Drug content of VCM@Fe3O4/HNT–C(WR)3 and OFX@Fe3O4/HNT–C(WR)3 nanocargoes. To make an approximate estimation of drug content loaded into the Fe3O4/HNT–C(WR)3 nanocarrier, UV-vis absorbance spectroscopy was used. For this purpose, two calibration curves (absorption by concentration) were drawn using five standard solutions of VCM and OFX in phosphate buffer saline (PBS, 0.1 M, pH = 6.8), with the concentrations of 5, 10, 15, 20, and 25 ppm. From the obtained line equations, concentration of the unknown solutions (in ppm) can be the obtained through putting the UV-vis absorbance values (y) in the equations (Fig. 6).72 For preparation of the samples, 50 mg of VCM@Fe3O4/HNT–C(WR)3 and OFX@Fe3O4/HNT–C(WR)3 particles were put in two separate flasks, then ultrasonicated in 25 mL of dimethyl sulfoxide (DMSO), for an hour at room temperature. Simultaneously, the same procedure was carried out for a sample of Fe3O4/HNT–C(WR)3 particles to provide an appropriate solution for the base line. After completion of the process, the particles were collected by an external magnet, and separated. The excess particles were removed by centrifugation (4K rpm) and paper filtration, until a clear solution was obtained. Then, dilution of the obtained solutions with the PBS medium was performed through transfer of 1.0 mL of the sample solution to 25 mL of the PBS. Finally, the obtained solutions were studied by UV-vis spectroscopy at 281 and 298 nm for VCM and OFX, respectively. As summarized in Table 1, drug content values for VCM@Fe3O4/HNT–C(WR)3 and OFX@Fe3O4/HNT–C(WR)3 nanocargoes have been estimated (10.0 ± 0.8) wt% and (12.0 ± 1.1) wt%, respectively.
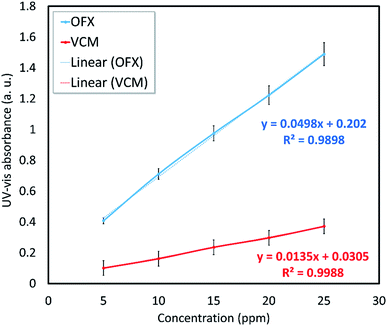 |
| Fig. 6 UV-vis absorbance–concentration calibration curves of VCM and OFX standard solutions in PBS (0.1 M, pH = 6.8), obtained in five points. Error bars represent standard deviations for three samples of each concentration (n = 3). | |
Table 1 The obtained results from drug content study on VCM@Fe3O4/HNT–C(WR)3 and OFX@Fe3O4/HNT–C(WR)3 samples
Sample |
Aa (a. u.) |
Cb (mg mL−1) |
Drug contentc (wt%) |
Rel. E.d (%) |
UV-vis absorbance activity of the filtered solutions after separation of the particles, at 281 and 298 nm. Concentration of VCM or OFX in the initial samples containing 50 mg of particles and 25 mL of DMSO. Drug content values were estimated via calculation methods given in the ESI section. Relative errors were obtained by considering the values for three samples for each case (n = 3). |
VCM@Fe3O4/HNT–C(WR)3 |
0.138 |
0.20 |
10.0 |
8.1 |
OFX@Fe3O4/HNT–C(WR)3 |
0.680 |
0.24 |
12.0 |
9.2 |
3.3.2 Drug release behavior of VCM@Fe3O4/HNT–C(WR)3 and OFX@Fe3O4/HNT–C(WR)3 nanocargoes. To do investigations on drug release process from VCM@Fe3O4/HNT–C(WR)3 and OFX@Fe3O4/HNT–C(WR)3 nanocargoes, similar portions of the particles were well dispersed in three buffered media, and stirred at 37 °C. For this purpose, PBS (0.1 M) with the pH values of 6.8 and 9.0, and acetate buffer (AcB, 0.1 M) with pH = 4.6 were used. This is an important point for the prepared cargoes to release majority of the encapsulated drug in the acidic medium, since the cargoes will experience a similar condition into the cytoplasm environment after the internalization process.73,74 According to literature, there are hydrogen bond (H-bond) interactions between the silanol and aluminol groups in the layered structure of HNTs, which provides a suitable substrate for drug encapsulation. In the acidic conditions, this H-bond network is dissociated and consequently the loaded drug is released.51 This structural feature of the HNTs results in the huge prevention of drug leaching in the pH-neutral environments. The release amount of VCM and OFX were monitored by UV-vis absorbance spectroscopy, via the same procedure explained in the section of drug content estimation. Also, the calibration curves and line equations presented in Fig. 6 were used for this purpose. Table 2 reports the obtained results from drug release screening experiments. According to the table, as expected, the major release; (91.3 ± 4.7)% for VCM and (92.9 ± 3.3)% for OFX, occurs in the acidic conditions provided by AcB, during a 90 minutes process.
Table 2 The obtained results from drug release study on VCM@Fe3O4/HNT–C(WR)3 and OFX@Fe3O4/HNT–C(WR)3 samples
Sample |
Conditiona |
Ab (a. u.) |
Time (min) |
Releasec (%) |
Rel. E.d (%) |
All samples were prepared in 2.0 mg mL−1 concentration, and stirred at 37 °C. Ultrasonication (50 kHz, 100 W L−1) has been applied for 2 minutes, to make a fine dispersion of the particles. UV-vis absorbance activity of the filtered solutions were recorded at 281 and 298 nm for VCM and OFX, respectively. All absorbance digits were compared with the maximum values (A0 = 0.138 and 0.680) obtained in drug content experiment. The percentage of the released amounts have been calculated via the following equation, A/A0 × 100. Relative errors were obtained by considering the values for three samples for each case (n = 3). |
VCM@Fe3O4/HNT–C(WR)3 |
PBS (6.8) |
<0.01 |
60 |
Partial |
10.9 |
PBS (6.8) |
0.033 |
90 |
23.9 |
11.1 |
PBS (9.0) |
0.058 |
60 |
42.0 |
10.2 |
PBS (9.0) |
0.092 |
90 |
66.7 |
7.4 |
AcB (4.6) |
0.108 |
60 |
78.2 |
5.5 |
AcB (4.6) |
0.126 |
90 |
91.3 |
5.2 |
OFX@Fe3O4/HNT–C(WR)3 |
PBS (6.8) |
0.061 |
60 |
8.8 |
9.3 |
PBS (6.8) |
0.109 |
90 |
16.0 |
8.2 |
PBS (9.0) |
0.309 |
60 |
45.4 |
4.7 |
PBS (9.0) |
0.412 |
90 |
60.6 |
5.8 |
AcB (4.6) |
0.566 |
60 |
83.2 |
4.2 |
AcB (4.6) |
0.632 |
90 |
92.9 |
3.6 |
3.3.3 Cell attachment and uptake of the nanocargoes. To investigate cellular uptake process for the prepared therapeutic nanocargoes, VCM@Fe3O4/HNT–C(WR)3 particles were studied on two cell lines; S. aureus, as a Gram-positive and E. coli, as a Gram-negative bacterial strain. To make a meaningful comparison, individual VCM was also subjected to the cells as a control. For this purpose, a 90 minutes process was executed at 37 °C and 90% humidity. In order to make the target cells visible in confocal microscopy, cell-membrane staining was carried out using crystal violet, which shows the photoluminescence emission activity at ca. 590 nm.75 Generally, the merge panels reveal cell-attachment and co-localization ratios, where a compilation of two different colors appears. Also, the intensity of the merged colors origins from the compilation ratio.76 Fig. 7 illustrates the obtained images from confocal microscopy after incubation of the compounds with the cells. As is observed, for the S. aureus cells, formation of more amounts of purple color with high intensity in the merge panel of VCM-nanocargo confirms higher degree of co-localization in comparison with the individual VCM. In the same way, for E. coli strain, formation of the white color in the merge panel corroborates the overlap of the yellow and blue colors, originating from co-localization of the VCM and the living cells. As is seen in the merge panels, the white color is observed in more areas of the image of VCM-nanocargo, than the individual VCM. These observations well disclose that cell adhesion as the most important stage of the therapeutic program is significantly enhanced through conjugation of the C(WR)3 peptide sequence to the drug carriers.
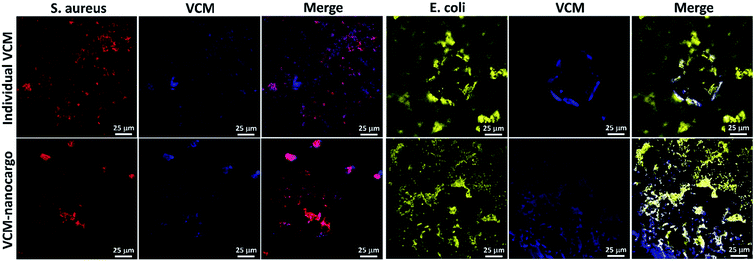 |
| Fig. 7 Confocal microscopy images of the co-localized VCM@Fe3O4/HNT–C(WR)3 nanoparticles (blue color) with S. aureus (red color) and E. coli (yellow color) bacteria cells, during 90 minutes incubation (temperature: 37 °C, humidity: 90%). Cell staining has been carried out using crystal violet (emission at 590 nm). | |
3.3.4 Antimicrobial behavior of VCM@Fe3O4/HNT–C(WR)3 and OFX@Fe3O4/HNT–C(WR)3 nanocargoes.
3.3.4.1 Optical density experiment. In order to evaluate antimicrobial potency of the prepared VCM@Fe3O4/HNT–C(WR)3 and OFX@Fe3O4/HNT–C(WR)3 nanocargoes, optical density experiments (OD600) were performed on the samples.77 For this purpose, the same concentration (50 μg mL−1) of the nanocargoes and related controls including Fe3O4/HNTs, Fe3O4/HNT–C(WR)3, and individual C(WR)3 peptide sequence, VCM and OFX were assessed over S. aureus and E. coli, as Gram-positive and Gram negative bacteria strains, respectively. The OD activity of the samples was studied at four times; 0, 30, 60, and 90 minutes. Initially, cell colonies were cultivated in LB broth medium with a cell density value of ca. 107 CFU mL−1, via incubation for 24 hours at 37 °C and humidity of 95%. It should be noted that sterilization of the tools at 120 °C before starting the work is essential. Dulbecco's modified Eagle's medium (DMEM) was used for preparation of the dispersions of the particles. As presented in diagrams (Fig. 8), significant growth inhibitions occurred by VCM@Fe3O4/HNT–C(WR)3 and OFX@Fe3O4/HNT–C(WR)3 nanocargoes, compared respect to the controls. During a 90 minutes process, (75.2 ± 1.9)% and (64.8 ± 1.5)% growth inhibition have been obtained by subjection of VCM@Fe3O4/HNT–C(WR)3 particles to the S. aureus and E. coli cells, respectively. These values increased to (78.0 ± 1.6)% and (70.2 ± 1.4)% for the OFX@Fe3O4/HNT–C(WR)3 particles. This difference may be due to more loading ratio of OFX into the tubes than the VCM (Section 3.3.1). For the individual VCM and OFX with the same concentration as the particles, (60.1 ± 1.9)% and (62.9 ± 2.0)% on S. aureus, and (48.8 ± 2.8)% and (46.0 ± 1.5)% on E. coli, bacteriostatic effects have been observed, during 90 minutes. Since, there have been no noticeable antimicrobial effects for the neat Fe3O4/HNTs, individual C(WR)3, and Fe3O4/HNTs–C(WR)3, the observed difference between the individual drugs and the final products (nanocargoes) is attributed to the efficacy of the C(WR)3 peptide sequence. As is seen, there was also no remarkable growth inhibition effect for the individual C(WR)3, concluding that this compound is playing a role just in the cell-penetration process. Partial antimicrobial effects have been exhibited for the individual C(WR)3 and Fe3O4/HNTs–C(WR)3 on both cell lines. These amounts of growth inhibition can be ascribed to the sulfur and disulfide bonds, which are able to induce the antimicrobial trait.78 Since the prepared nanocargoes will be suggested for the clinical use, it is essential to evaluate the cytotoxicity levels of the products on eukaryotic cells, as well. For this purpose, 3T3 fibroblast strain was chosen as a human normal cell line. Briefly, the same procedure as the bacterial cells was implemented at which the 3T3 cells were cultured in LB broth medium with a cell density value of ∼107 CFU mL−1, and the same concentration (50 μg mL−1) of the individual VCM, OFX, and both nanocargoes were subjected to the cells after well dispersion in DMEM by ultrasound waves. As shown in Fig. S4 (in ESI file†), no significant growth inhibition was observed after subjection of the nanocargoes during a 90 min incubation process. As is seen, after passing 30 minutes of incubation, the growth rate has been a bit affected by the individual VCM and OFX. This partial influence can be attributed to the change of pH to acidic values after dissolution of VCM and OFX.79
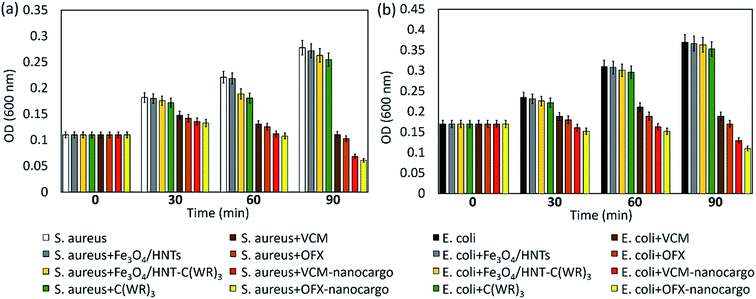 |
| Fig. 8 Bacteriostatic effects of VCM@Fe3O4/HNT–C(WR)3 and OFX@Fe3O4/HNT–C(WR)3 nanocargoes on (a) S. aureus and (b) E. coli strains. Fe3O4/HNTs, Fe3O4/HNT–C(WR)3, and individual C(WR)3 peptide sequence, VCM and OFX have been experimented as the controls. All samples have been prepared and subjected to the cells in the same concentration of 50 μg mL−1 (in DMEM). Ultrasonication in a bath with 50 kHz frequency and 100 W L−1 power density (2 minutes, at room temperature) was performed on the heterogeneous samples to make fine dispersions. The error bars show relative error (%) for three samples of each condition (n = 3). | |
3.3.4.2 Zone of inhibition and colony counter experiments. To perform more investigations on the bacteriostatic properties of the prepared VCM@Fe3O4/HNT–C(WR)3 and OFX@Fe3O4/HNT–C(WR)3 nanocargoes, the zones of growth inhibition (ZOIs) in the cultivation Petri dish (containing nutrient agar) have been diametrically evaluated. For this purpose, S. aureus and E. coli cells were incubated in the presence of 10 mg of the powder samples of the products and the controls, for 24 hours. Fig. 9 illustrates the provided images from dishes after the completion of the incubation time. As is observed, for S. aurous (images a–c), VCM@Fe3O4/HNT–C(WR)3 (VCM-nanocargo) and OFX@Fe3O4/HNT–C(WR)3 (OFX-nanocargo) have created inhibition zones with (3.7 ± 0.2) cm and (3.9 ± 0.2) cm diameters, respectively. Whereas, the same dosage of the individual VCM and OFX have formed zones with (3.2 ± 0.2) cm diameter. These meaningful differences in the antimicrobial activity between the prepared nanocargoes (with only ca. 11 wt% drug content) and the individual drug compounds, well corroborate enhancement of antimicrobial properties through incorporation of the drugs inside the nano-carrier and conjugation to the C(WR)3 peptide sequence. As well, this is seen that the controls such as HNTs, Fe3O4/HNTs, Fe3O4/HNT–C(WR)3, and C(WR)3 peptide sequence have solely shown no considerable antimicrobial effects on both cell lines. The same result is observed for the E. coli cells (images d–f), with this difference that the growth inhibition has been a bit reduced totally for all samples. It may origin from higher resistance of Gram-negative bacteria against membrane penetration.80 To have a quantitative assessment on bacteria cell killing by the prepared nanocargoes, disk counter experiment was performed via subjection of VCM@Fe3O4/HNT–C(WR)3 particles to S. aureus cells. For this purpose, bacteria cells were cultivated in LB broth medium for 24 hours at 37 °C. Then, the opacity of the solution reached to 0.4, and a suspension of VCM@Fe3O4/HNT–C(WR)3 in DMEM (5 mg mL−1) was prepared using an ultrasound bath for well dispersion. The population of the bacteria cells in the dishes was counted over 24 hours. The obtained results have revealed that great antibacterial treatment is executed by the prepared nanocargoes, as ca. (96.6 ± 14.3)% cell death was occurred in the dishes (Fig. S5, in ESI section†). Table 3 concisely reports the obtained results from the ZOI experiments on the samples.
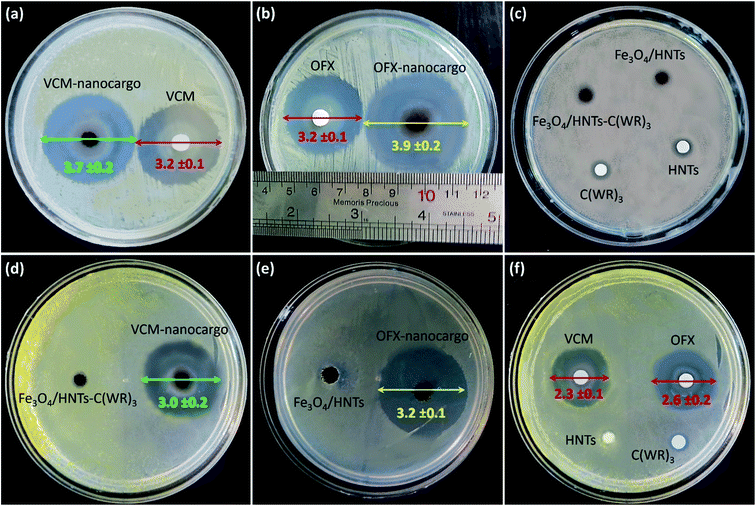 |
| Fig. 9 Digital images of the inhibition zones created by VCM@Fe3O4/HNT–C(WR)3 and OFX@Fe3O4/HNT–C(WR)3 nanocargoes, in comparison with the controls including VCM, OFX, HNTs, Fe3O4/HNTs, Fe3O4/HNT–C(WR)3, and C(WR)3 peptide sequence, over S. aureus (a–c) and E. coli (d–f) cells. Incubation was carried out at 37 °C and humidity of 95%, for 24 hours. | |
Table 3 The obtained results from ZOI experiments on VCM@Fe3O4/HNT–C(WR)3 and OFX@Fe3O4/HNT–C(WR)3 nanocargoes in comparison with the controls
Sample |
Zone diameter on S. aureus (cm) |
Zone diameter on E. coli (cm) |
VCM |
3.2 ± 0.1 |
2.3 ± 0.1 |
OFX |
3.2 ± 0.1 |
2.6 ± 0.2 |
C(WR)3 |
Partial |
Partial |
HNTs |
Partial |
Partial |
Fe3O4/HNTs |
Partial |
Partial |
Fe3O4/HNT–C(WR)3 |
Partial |
Partial |
VCM@Fe3O4/HNT–C(WR)3 |
3.7 ± 0.2 |
3.0 ± 0.2 |
OFX@Fe3O4/HNT–C(WR)3 |
3.9 ± 0.2 |
3.2 ± 0.1 |
3.3.4.3 Minimum inhibitory and bactericidal concentrations. As another comparative method, minimum inhibitory concentration (MIC) and minimum bactericidal concentration (MBC) values were evaluated for the samples of VCM@Fe3O4/HNT–C(WR)3 and OFX@Fe3O4/HNT–C(WR)3 nanocargoes, and the obtained results were compared with the controls. MIC and MBC are defined based on the concentration of an antimicrobial agent that inhibits the growth (for bacteriostatic agents) or kills all bacteria cells (for bactericidal agents).81 According to the standard procedure, the bacteria cells (S. aureus and E. coli) were cultivated in agar LB broth medium at 37 °C, for 24 hours (108 CFU mL−1). A series of diluted samples of VCM@Fe3O4/HNT–C(WR)3 and OFX@Fe3O4/HNT–C(WR)3 suspensions with a geometric sequence of concentration (2, 4, 8, 16, 32, 64, 128, and 526 μg mL−1) have been experimented in 2.0 mL of Mueller–Hinton broth (M–H broth) liquid medium followed by fresh bacterial suspension with a turbidity of 0.5. Disk counter method was used for evaluation of the growth inhibition or bactericidal effects. Table 4 summarizes the obtained results from MIC/MBC experiments. As is observed, similar MIC/MBC values have been obtained for the prepared nanocargoes and the individual VCM and OFX. The point is that only 10 wt% and 12 wt% of the nanocargoes belong to the VCM and OFX, while the same values as the pure drugs are obtained. In the case of E. coli cell line, this is seen that both MIC and MBC have been reduced for VCM@Fe3O4/HNT–C(WR)3 than the individual VCM, meaning that the carried VCM overcomes the bacterial resistance via incorporation into the tubes and conjugation to the C(WR)3 peptide sequence.
Table 4 Obtained results from MIC/MBC experiments on VCM@Fe3O4/HNT–C(WR)3 and OFX@Fe3O4/HNT–C(WR)3 nanocargoes, over S. aureus and E. coli cells
Sample |
MIC (μg mL−1) |
MBC (μg mL−1) |
S. aureus |
VCM |
8 |
64 |
OFX |
2 |
2 |
VCM@Fe3O4/HNT–C(WR)3 |
8 |
64 |
OFX@Fe3O4/HNT–C(WR)3 |
2 |
2 |
E. coli |
VCM |
32 |
128 |
OFX |
2 |
4 |
VCM@Fe3O4/HNT–C(WR)3 |
16 |
64 |
OFX@Fe3O4/HNT–C(WR)3 |
2 |
4 |
3.3.4.4 Physiological stability experiments. Since aggregation of the particles may negatively affect the internalization and drug release processes, it is of high importance to investigate aggregation states of the magnetic nanocargoes in a simulated physiological environment. For this purpose, a circulation system was prepared and VCM@Fe3O4/HNT–C(WR)3 nanocargoes were experimented in human serum albumin (HSA, 25%) solution.13 Accordingly, circulation of the particles (0.4 mg mL−1) was performed for 15, 30, and 60 minutes in HSA at 37 °C, and ultimately aggregation states were investigated by DLS analysis. As shown in the obtained DLS spectra (Fig. S6–S8, in the ESI section†), the average particle size has increased from ca. 513 nm to 1355 nm during a 60 min circulation, meaning that the efficiency of the prepared nanocargoes may decrease over the time due to the particle aggregation. This point clearly highlights the importance of the magnetic direction of the particles in the body's internal environment, which expedites the drug delivery process. It should be also noticed that electrostatic repulsion between the positive-charged guanidine groups onto the surfaces may assist dispersion of the particles and slow down the aggregation process.To investigate structural stability and degradation state of the designed drug carrier in the simulated physiological conditions, nano-carrier particles were experimented at 37 °C. For this purpose, four identical samples of Fe3O4/HNTs dispersion (10 mg mL−1) in HSA medium were stirred for 12, 24, 48, 72, and 120 hours. After completion of each time, the particles were magnetically separated from the mixture and well rinsed with deionized water and ethanol, dried, and weighted. Also, the supernatant was evaluated for tracing any metal ions (Si, Al, and Fe) released from degraded Fe3O4/HNTs. For this aim, inductively coupled plasma (ICP) analysis was employed. To have statistical evaluation, the whole assessment was repeated for three times. As reported in Table 5, half-life of the Fe3O4/HNTs in the physiological conditions has been estimated to be ca. 72 hours, which is an appropriate time in the pharmacodynamics studies.82
Table 5 The weight values of recollected Fe3O4/HNTs, and corresponding concentrations measured by ICP analysis in the supernatants after circulation for 120 hours, at 37 °C (pharmacodynamics)
Entry |
Time of experiment (h) |
Weighta (mg) |
Rel. errorb (%) |
Fec (ppm) |
Si (ppm) |
Al (ppm) |
100 mg of Fe3O4/HNTs was dispersed in 10.0 mL of HAS. Relative errors have been calculated for three samples for each condition (n = 3). Concentrations of the leached elements in the media, obtained by ICP analysis. Estimated half-life. |
1 |
0 |
100 |
— |
— |
— |
— |
2 |
12 |
97.2 |
5.3 |
Trace |
Trace |
Trace |
3 |
24 |
91.9 |
6.6 |
1.08 |
0.72 |
0.36 |
4 |
48 |
68.3 |
8.6 |
4.32 |
3.10 |
1.37 |
5 |
72d |
52.5 |
11.5 |
6.36 |
4.65 |
2.02 |
6 |
120 |
34.3 |
17.6 |
8.74 |
6.36 |
2.81 |
4. Conclusion
Incorporation of different types of medications in the delivery systems has shown to be a great method for the enhancement of therapeutic effects. In this report, a novel designed drug delivery system constructed of the natural HNTs, Fe3O4 NPs, and a conjugated peptide chain including cysteine, arginine, and tryptophan (named as C(WR)3), has been presented and suggested for targeted delivery of VCM and OFX antibiotics. The designed CPP sequence containing arginine–tryptophan blocks (synthesized on the CTC solid resin) conjugated to the particles, has enhanced cell adhesion and subsequent internalization into the living cells, confirmed by confocal microscopy. The drug release studies have shown that above 90% of the encapsulated drug in the tubes is released in the acidic conditions. This property corroborates major release of drugs in acidic environment of the cells, after the internalization process. The drug content of the prepared VCM@Fe3O4/HNT–C(WR)3 and OFX@Fe3O4/HNT–C(WR)3 nanocargoes has been evaluated to be 10 wt% and 12 wt%, respectively. As the main brilliant point, it has been observed that almost similar bacteriostatic effects have been observed for the prepared nanocargoes (VCM@Fe3O4/HNT–C(WR)3 and OFX@Fe3O4/HNT–C(WR)3) and the individual VCM and OFX, in the same dosage. The antimicrobial experiments have revealed that the growth rate is highly inhibited through subjection of the nanocargoes to the Gram-positive and -negative bacteria strains, during a short time. In comparison with the neat VCM and OFX with the same dosage as the nanocargoes, the significant enhancement of the antimicrobial effects has been corroborated. Since, the designed nanocargoes are suggested for the clinical use in the real-life, the cytotoxic effects on 3T3 fibroblast as a eukaryotic strain has been investigated, as well. The results demonstrated that the human normal cells are not affected by the subjected nanocargoes. Moreover, the half-life of the designed nanocargoes has been estimated around 72 hours in the simulated physiological environment, which is an appropriate time for biodegradation. However, biodistribution and aggregation states of the particles in the body's internal environment should be further investigated via animal testing, because they may significantly affect the cellular uptake and drug release processes. Also, the release strategy could be controlled through coating the designed nanocargoes with different types of materials like polymers. For example, to have a delayed release in drug delivery to intestine, some polymeric structures responsive to alkaline conditions can be used for the coating of the whole cargo. Overall, via performing these studies on the presented samples, we can get closer to the real-life utility in antimicrobial treatment.
Conflicts of interest
The authors declare no conflict of interest.
Acknowledgements
The authors gratefully acknowledge the partial support from the Research Council of the Baqiyatallah University of Medical Sciences.
References
- A. Maleki, R. Taheri-Ledari, R. Eivazzadeh-Keihan, M. de la Guardia and A. Mokhtarzadeh, Preparation of carbon-14 labeled 2-(2-mercaptoacetamido)-3-phenylpropanoic acid as metallo-beta-lactamases inhibitor (MBLI), for coadministration with beta-lactam antibiotics, Curr. Org. Synth., 2019, 16, 765–771 CrossRef CAS PubMed.
- J. D. Chang, E. E. Foster, A. G. Wallace and S. J. Kim, Peptidoglycan O-acetylation increases in response to vancomycin treatment in vancomycin-resistant Enterococcus faecalis, Sci. Rep., 2017, 7(1), 1–8 CrossRef PubMed.
- N. Pandey and M. Cascella, Beta lactam antibiotics, StatPearls, 2021 Search PubMed.
- V. M. Chauhan, H. Zhang, P. A. Dalby and J. W. Aylott, Advancements in the co-formulation of biologic therapeutics, J. Controlled Release, 2021, 327, 397–405 CrossRef PubMed.
- M. H. Baghersad, S. Jamshidi, A. Habibi and A. Salimi, Synthesis, Characterization, and In Vitro Evaluation of Super Paramagnetic Nanoparticles Grafted with PAMPS for Controlled Delivery of Cationic Drugs, ChemistrySelect, 2019, 4, 810–815 CrossRef CAS.
- R. Taheri-Ledari, A. Maleki, E. Zolfaghari, M. Radmanesh, H. Rabbani, A. Salimi and R. Fazel, High-performance sono/nano-catalytic system: Fe3O4@ Pd/CaCO3-DTT core/shell nanostructures, a suitable alternative for traditional reducing agents for antibodies, Ultrason. Sonochem., 2020, 61, 104824 CrossRef CAS PubMed.
- S. Parvaz, R. Taheri-Ledari, M. S. Esmaeili, M. Rabbani and A. Maleki, A brief survey on the advanced brain drug administration by nanoscale carriers: with a particular focus on AChE reactivators, Life Sci., 2020, 240, 117099 CrossRef CAS PubMed.
- J. Seifi, V. Goodarzi, F. R. Wurm, S. Shojaei, M. Jafari-Nodoushan, N. Najmoddin, H. A. Khonakdar, M. H. Baghersad and L. Uzun, Developing antibacterial superhydrophobic coatings based on polydimethylsiloxane/silver phosphate nanocomposites: assessment of surface morphology, roughness and chemistry, Prog. Org. Coat., 2020, 149, 105944 CrossRef.
- R. Eivazzadeh-Keihan, K. K. Chenab, R. Taheri-Ledari, J. Mosafer, S. M. Hashemi, A. Mokhtarzadeh, A. Maleki and M. R. Hamblin, Recent advances in the application of mesoporous silica-based nanomaterials for bone tissue engineering, Mater. Sci. Eng., C, 2020, 107, 110267 CrossRef CAS PubMed.
- A. J. Huh and Y. J. Kwon, “Nanoantibiotics”: a new paradigm for treating infectious diseases using nanomaterials in the antibiotics resistant era, J. Controlled Release, 2011, 156(2), 128–145 CrossRef CAS PubMed.
- L. B. Capeletti, L. F. de Oliveira, K. D. A. Goncalves, J. F. A. de Oliveira, A. Saito, J. Kobarg, J. H. Z. D. Santos and M. B. Cardoso, Tailored silica–antibiotic nanoparticles: overcoming bacterial resistance with low cytotoxicity, Langmuir, 2014, 30(25), 7456–7464 CrossRef CAS PubMed.
- N. Elahi, M. Kamali and M. H. Baghersad, Recent biomedical applications of gold nanoparticles: a review, Talanta, 2018, 184, 537–556 CrossRef CAS PubMed.
- R. Taheri-Ledari, W. Zhang, M. Radmanesh, S. S. Mirmohammadi, A. Maleki, N. Cathcart and V. Kitaev, Multi-stimuli nanocomposite therapeutic: docetaxel targeted delivery and synergies in treatment of human breast cancer tumor, Small, 2020, 16(41), 2002733 CrossRef CAS PubMed.
- Z. Jin, K. Wu, J. Hou, K. Yu, Y. Shen and S. Guo, A PTX/nitinol stent combination with temperature-responsive phase-change 1-hexadecanol for magnetocaloric drug delivery: magnetocaloric drug release and esophagus tissue penetration, Biomaterials, 2018, 153, 49–58 CrossRef CAS PubMed.
- M. Fizir, P. Dramou, N. S. Dahiru, W. Ruya, T. Huang and H. He, Halloysite nanotubes in analytical sciences and in drug delivery: a review, Microchim. Acta, 2018, 185(8), 1–33 CrossRef CAS PubMed.
- S. Satish, M. Tharmavaram and D. Rawtani, Halloysite nanotubes as a nature's boon for biomedical applications, Nanobiomedicine, 2019, 6 DOI:10.1177/1849543519863625.
- M. M. Al-Zu'bi and A. S. Mohan, Modelling of implantable drug delivery system in tumor microenvironment using molecular communication paradigm, IEEE Access, 2019, 7, 141929–141940 Search PubMed.
- S. Sharif, G. Abbas, M. Hanif, A. Bernkop-Schnürch, A. Jalil and M. Yaqoob, Mucoadhesive micro-composites: chitosan coated halloysite nanotubes for sustained drug delivery, Colloids Surf., B, 2019, 184, 110527 CrossRef CAS PubMed.
- Z. Vuluga, M. C. Corobea, C. Elizetxea, M. Ordonez, M. Ghiurea, V. Raditoiu, C. A. Nicolae, D. Florea, M. Iorga, R. Somoghi and B. Trica, Morphological and tribological properties of PMMA/halloysite nanocomposites, Polymers, 2018, 10(8), 816 CrossRef PubMed.
- M. Tharmavaram, G. Pandey and D. Rawtani, Surface modified halloysite nanotubes: a flexible interface for biological, environmental and catalytic applications, Adv. Colloid Interface Sci., 2018, 261, 82–101 CrossRef CAS PubMed.
- R. Taheri-Ledari and A. Maleki, Magnetic hybrid nanocatalysts, Magnetic Nanoparticle-Based Hybrid Materials, Woodhead Publishing, 2021, pp. 619–636 Search PubMed.
- R. Taheri-Ledari and A. Maleki, Magnetic nanocatalysts utilized in the synthesis of aromatic pharmaceutical ingredients, New J. Chem., 2021, 45(9), 4135–4146 RSC.
- A. Maleki, R. Taheri-Ledari, R. Ghalavand and R. Firouzi-Haji, Palladium-decorated o-phenylenediamine-functionalized Fe3O4/SiO2 magnetic nanoparticles: a promising solid-state catalytic system used for Suzuki–Miyaura coupling reactions, J. Phys. Chem. Solids, 2020, 136, 109200 CrossRef CAS.
- R. Taheri-Ledari, J. Rahimi, A. Maleki and A. E. Shalan, Ultrasound-assisted diversion of nitrobenzene derivatives to their aniline equivalents through a heterogeneous magnetic Ag/Fe3O4-IT nanocomposite catalyst, New J. Chem., 2020, 44(45), 19827–19835 RSC.
- R. Taheri-Ledari, M. S. Esmaeili, Z. Varzi, R. Eivazzadeh-Keihan, A. Maleki and A. E. Shalan, Facile route to synthesize Fe3O4@ acacia–SO3H nanocomposite as a heterogeneous magnetic system for catalytic applications, RSC Adv., 2020, 10(66), 40055–40067 RSC.
- M. Pura, A. Wibowo, H. Ardy and B. S. Purwasasmita, Fabrication of Fe3O4 nanoparticles coated with dimethylaminobenzyl-chitosan, AIP Conf. Proc., 2020, 2262, 030005 CrossRef CAS.
- N. Elahi, M. H. Baghersad and M. Kamali, Precise, direct, and rapid detection of Shigella Spa gene by a novel unmodified AuNPs-based optical genosensing system, J. Microbiol. Methods, 2019, 162, 42–49 CrossRef CAS PubMed.
- P. Nasiripur, M. Zangiabadi and M. H. Baghersad, Visible light photocatalytic degradation of methyl parathion as chemical warfare agents simulant via GO-Fe3O4/Bi2MoO6 nanocomposite, J. Mol. Struct., 2021, 1243, 130875 CrossRef CAS.
- R. Taheri-Ledari, S. S. Mirmohammadi, K. Valadi, A. Maleki and A. E. Shalan, Convenient conversion of hazardous nitrobenzene derivatives to aniline analogues by Ag nanoparticles, stabilized on a naturally magnetic pumice/chitosan substrate, RSC Adv., 2020, 10(71), 43670–43681 RSC.
- R. Taheri-Ledari, M. Saeidirad, F. S. Qazi, A. Fazeli, A. Maleki and A. E. Shalan, Highly porous copper-supported magnetic nanocatalysts: made of volcanic pumice textured by cellulose and applied for the reduction of nitrobenzene derivatives, RSC Adv., 2021, 11(41), 25284–25295 RSC.
- R. Taheri-Ledari, K. Valadi, S. Gharibi and A. Maleki, Synergistic photocatalytic effect between green LED light and Fe3O4/ZnO-modified natural pumice: a novel cleaner product for degradation of methylene blue, Mater. Res. Bull., 2020, 130, 110946 CrossRef CAS.
- R. Taheri-Ledari, S. M. Hashemi and A. Maleki, High-performance sono/nano-catalytic system: CTSN/Fe3O 4–Cu nanocomposite, a promising heterogeneous catalyst for the synthesis of N-arylimidazoles, RSC Adv., 2019, 9(69), 40348–40356 RSC.
- M. K. Manshadi, M. Saadat, M. Mohammadi, M. Shamsi, M. Dejam, R. Kamali and A. Sanati-Nezhad, Delivery of magnetic micro/nanoparticles and magnetic-based drug/cargo into arterial flow for targeted therapy, Drug Delivery, 2018, 25(1), 1963–1973 CrossRef CAS PubMed.
- Z. Varzi, M. S. Esmaeili, R. Taheri-Ledari and A. Maleki, Facile synthesis of imidazoles by an efficient and eco-friendly heterogeneous catalytic system constructed of Fe3O4 and Cu2O nanoparticles, and guarana as a natural basis, Inorg. Chem. Commun., 2021, 125, 108465 CrossRef CAS.
- Z. Hajizadeh, K. Valadi, R. Taheri-Ledari and A. Maleki, Convenient Cr (VI) removal from aqueous samples: executed by a promising clay-based catalytic system, magnetized by Fe3O4 nanoparticles and functionalized with humic acid, ChemistrySelect, 2020, 5(8), 2441–2448 CrossRef CAS.
- A. Maleki, M. Niksefat, J. Rahimi and R. Taheri-Ledari, Multicomponent synthesis of pyrano[2,3-d]pyrimidine derivatives via a direct one-pot strategy executed by novel designed copperated Fe3O4@polyvinyl alcohol magnetic nanoparticles, Mater. Today Chem., 2019, 13, 110–120 CrossRef CAS.
- R. Eyvazzadeh-Keihan, N. Bahrami, R. Taheri-Ledari and A. Maleki, Highly facilitated synthesis of phenyl(tetramethyl)acridinedione pharmaceuticals by a magnetized nanoscale catalytic system, constructed of GO, Fe3O4 and creatine, Diamond Relat. Mater., 2020, 102, 107661 CrossRef CAS.
- R. Taheri-Ledari, J. Rahimi and A. Maleki, Synergistic catalytic effect between ultrasound waves and pyrimidine-2,4-diamine-functionalized magnetic nanoparticles: applied for synthesis of 1,4-dihydropyridine pharmaceutical derivatives, Ultrason. Sonochem., 2019, 59, 104737 CrossRef PubMed.
- R. Eivazzadeh-Keihan, R. Taheri-Ledari, N. Khosropour, S. Dalvand, A. Maleki, S. M. Mousavi-Khoshdel and H. Sohrabi, Fe3O4/GO@melamine-ZnO nanocomposite: a promising versatile tool for organic catalysis and electrical capacitance, Colloids Surf., A, 2020, 587, 124335 CrossRef CAS.
- J. Rahimi, R. Taheri-Ledari and A. Maleki, Cellulose-Supported Sulfonated Magnetic Nanoparticles: Utilized for One-pot Synthesis of α-Iminonitrile Derivatives, Curr. Org. Synth., 2020, 17(4), 288–294 CrossRef CAS PubMed.
- R. Taheri-Ledari and A. Maleki, Antimicrobial therapeutic enhancement of levofloxacin via conjugation to a cell-penetrating peptide: an efficient sonochemical catalytic process, J. Pept. Sci., 2020, 26(10), e3277 CrossRef CAS PubMed.
- V. Soltaninejad, M. R. Ahghari, R. Taheri-Ledari and A. Maleki, Bifunctional PVA/ZnO/AgI/Chlorophyll Nanocomposite Film: Enhanced Photocatalytic Activity for Degradation of Pollutants and Antimicrobial Property under Visible-Light Irradiation, Langmuir, 2021, 37(15), 4700–4713 CrossRef CAS PubMed.
- K. R. B. Singh, P. Sridevi and R. Pratap Singh, Potential applications of peptide nucleic acid in biomedical domain, Eng. Rep., 2020, 2(9), e12238 CAS.
- R. P. Singh, B. K. Oh and J. W. Choi, Application of peptide nucleic acid towards development of nanobiosensor arrays, Bioelectrochemistry, 2010, 79(2), 153–161 CrossRef CAS PubMed.
- S. Subramaniam, P. Joyce, N. Thomas and C. A. Prestidge, Bioinspired drug delivery strategies for repurposing conventional antibiotics against intracellular infections, Adv. Drug Delivery Rev., 2021, 177, 113948 CrossRef CAS PubMed.
- U. Piotrowska, M. Sobczak and E. Oledzka, Current state of a dual behaviour of antimicrobial peptides—therapeutic agents and promising delivery vectors, Chem. Biol. Drug Des., 2017, 90(6), 1079–1093 CrossRef CAS PubMed.
- V. K. Gupta, A. Fakhri, S. Agarwal, E. Ahmadi and P. A. Nejad, Synthesis and characterization of MnO2/NiO nanocomposites for photocatalysis of tetracycline antibiotic and modification with guanidine for carriers of caffeic acid phenethyl ester-an anticancer drug, J. Photochem. Photobiol., B, 2017, 174, 235–242 CrossRef CAS PubMed.
- B. Casciaro, F. Cappiello, M. Cacciafesta and M. L. Mangoni, Promising approaches to optimize the biological properties of the antimicrobial peptide esculentin-1a (1–21) NH2: amino acids substitution and conjugation to nanoparticles, Front. Chem., 2017, 5, 26 CrossRef PubMed.
- Á. Martin-Serrano, R. Gómez, P. Ortega and F. J. de la Mata, Nanosystems as vehicles for the delivery of antimicrobial peptides (AMPs), Pharmaceutics, 2019, 11(9), 448 CrossRef PubMed.
- H. Lee, S. I. Lim, S. H. Shin, Y. Lim, J. W. Koh and S. Yang, Conjugation of cell-penetrating peptides to antimicrobial peptides enhances antibacterial activity, ACS Omega, 2019, 4(13), 15694–15701 CrossRef CAS PubMed.
- R. Taheri-Ledari, W. Zhang, M. Radmanesh, N. Cathcart, A. Maleki and V. Kitaev, Plasmonic photothermal release of docetaxel by gold nanoparticles incorporated onto halloysite nanotubes with conjugated 2D8-E3 antibodies for selective cancer therapy, J. Nanobiotechnol., 2021, 19(1), 1–21 CrossRef PubMed.
- A. Maleki, R. Taheri-Ledari and R. Ghalavand, Design and Fabrication of a Magnetite-based Polymer-supported Hybrid Nanocomposite: A Promising Heterogeneous Catalytic System Utilized in Known Palladium-assisted Coupling Reactions, Comb. Chem. High Throughput Screening, 2020, 23(2), 119–125 CrossRef CAS PubMed.
- M. S. Esmaeili, Z. Varzi, R. Taheri-Ledari and A. Maleki, Preparation and study of the catalytic application in the synthesis of xanthenedione pharmaceuticals of a hybrid nano-system based on copper, zinc and iron nanoparticles, Res. Chem. Intermed., 2021, 47, 973–996 CrossRef CAS.
- A. Maleki, R. Taheri-Ledari, J. Rahimi, M. Soroushnejad and Z. Hajizadeh, Facile peptide bond formation: effective interplay between isothiazolone rings and silanol groups at silver/iron oxide nanocomposite surfaces, ACS Omega, 2021, 4(6), 10629–10639 CrossRef PubMed.
- W. Zhang, R. Taheri-Ledari, Z. Hajizadeh, E. Zolfaghari, M. R. Ahghari, A. Maleki, M. R. Hamblin and Y. Tian, Enhanced activity of vancomycin by encapsulation in hybrid magnetic nanoparticles conjugated to a cell-penetrating peptide, Nanoscale, 2020, 12(6), 3855–3870 RSC.
- M. V. Sidorova, A. S. Molokoedov, A. A. Az'muko, E. V. Kudryavtseva, E. Krause, M. V. Ovchinnikov and Z. D. Bespalova, The Use of Hydrogen Peroxide for Closing Disulfide Bridges in Peptides, Russ. J. Bioorg. Chem., 2004, 30, 101–110 CrossRef CAS PubMed.
- M. Rouhi, M. Babamoradi, Z. Hajizadeh, A. Maleki and S. T. Maleki, Design and performance of polypyrrole/halloysite nanotubes/Fe3O4/Ag/Co nanocomposite for photocatalytic degradation of methylene blue under visible light irradiation, Optik, 2020, 212, 164721 CrossRef CAS.
- M. Massaro, S. Riela, G. Cavallaro, C. G. Colletti, S. Milioto, R. Noto, F. Parisi and G. Lazzara, Palladium supported on Halloysite-triazolium salts as catalyst for ligand free Suzuki cross-coupling in water under microwave irradiation, J. Mol. Catal. A: Chem., 2015, 408, 12–19 CrossRef CAS.
- M. Massaro, R. Noto and S. Riela, Past, present and future perspectives on halloysite clay minerals, Molecules, 2020, 25(20), 4863 CrossRef CAS PubMed.
- P. Yuan, D. Tan and F. Annabi-Bergaya, Properties and applications of halloysite nanotubes: recent research advances and future prospects, Appl. Clay Sci., 2015, 112, 75–93 CrossRef.
- J. Rahimi, R. Taheri-Ledari, M. Niksefat and A. Maleki, Enhanced reduction of nitrobenzene derivatives: effective strategy executed by Fe3O4/PVA-10% Ag as a versatile hybrid nanocatalyst, Catal. Commun., 2020, 134, 105850 CrossRef CAS.
- R. Taheri-Ledari, J. Rahimi and A. Maleki, Method screening for conjugation of the small molecules onto the vinyl-coated Fe3O4/silica nanoparticles: highlighting the efficiency of ultrasonication, Mater. Res. Express, 2020, 7(1), 015067 CrossRef CAS.
- J. González-Rivera, A. Spepi, C. Ferrari, I. Longo, J. T. Rodriguez, E. Fantechi, C. Innocenti, F. Pineider, M. A. Vera-Ramírez, M. R. Tiné and C. Duce, Structural, textural and thermal characterization of a confined nanoreactor with phosphorylated catalytic sites grafted onto a halloysite nanotube lumen, Appl. Clay Sci., 2020, 196, 105752 CrossRef.
- S. Balapangu, E. Nyankson, B. O. Asimeng, R. Asiamah, P. K. Arthur and E. K. Tiburu, Capturing Dioclea Reflexa Seed Bioactives on Halloysite Nanotubes and pH Dependent Release of Cargo against Breast (MCF-7) Cancers In Vitro, Separations, 2021, 8(3), 26 CrossRef CAS.
- K. Fidecka, J. Giacoboni, P. Picconi, R. Vago and E. Licandro, Quantification of amino groups on halloysite surfaces using the Fmoc-method, RSC Adv., 2020, 10(24), 13944–13948 RSC.
- S. S. Soltani, R. Taheri-Ledari, S. M. F. Farnia, A. Maleki and A. Foroumadi, Synthesis and characterization of a supported Pd complex on volcanic pumice laminates textured by cellulose for facilitating Suzuki–Miyaura cross-coupling reactions, RSC Adv., 2020, 10, 23359–23371 RSC.
- A. Maleki, R. Taheri-Ledari and M. Soroushnejad, Surface functionalization of magnetic nanoparticles via palladium-catalyzed Diels-Alder approach, ChemistrySelect, 2018, 3(46), 13057–13062 CrossRef CAS.
- P. Singh, K. R. B. Singh, J. Singh, S. Narayan Das and R. P. Singh, Tunable electrochemistry and efficient antibacterial activity of plant-mediated copper oxide nanoparticles synthesized by Annona squamosa seed extract for agricultural utility, RSC Adv., 2021, 11, 18050–18060 RSC.
- J. Singh, K. R. B. Singh, M. Kumar, R. Verma, R. Verma, P. Malik, S. Srivastava, R. P. Singh and D. Kumar, Melt-quenched vanadium pentoxide-stabilized chitosan nanohybrids for efficient hydrazine detection, Mater. Adv., 2021, 2, 6665–6675 RSC.
- S. A. Afolalu, O. D. Samuel and O. M. Ikumapayi, Development and characterization of nano-flux welding powder from calcined coconut shell ash admixture with FeO particles, J. Mater. Res. Technol., 2020, 9(4), 9232–9241 CrossRef CAS.
- M. Maruthupandy, G. Rajivgandhi, T. Muneeswaran, M. Anand and F. Quero, Highly efficient antibacterial activity of graphene/chitosan/magnetite nanocomposites against ESBL-producing Pseudomonas aeruginosa and Klebsiella pneumoniae, Colloids Surf., B, 2021, 202, 111690 CrossRef CAS PubMed.
- F. Taktak and Y. Öğen, Preparation and characterization of novel silk fibroin/2-(N,N-dimethylamino)ethyl methacrylate based composite hydrogels with enhanced mechanical properties for controlled release of cefixime, J. Macromol. Sci., Part A: Pure Appl.Chem., 2017, 54, 458–464 CrossRef CAS.
- M. Karimi, M. Eslami, P. Sahandi-Zangabad, F. Mirab, N. Farajisafiloo, Z. Shafaei, D. Ghosh, M. Bozorgomid, F. Dashkhaneh and M. R. Hamblin, pH-Sensitive stimulus-responsive nanocarriers for targeted delivery of therapeutic agents, Wiley Interdiscip. Rev.: Nanomed. Nanobiotechnol., 2016, 8(5), 696–716 CAS.
- A. C. Santos, C. Ferreira, F. Veiga, A. J. Ribeiro, A. Panchal, Y. Lvov and A. Agarwal, Halloysite clay nanotubes for life sciences applications: from drug encapsulation to bioscaffold, Adv. Colloid Interface Sci., 2018, 257, 58–70 CrossRef CAS PubMed.
- A. B. Grossman, D. J. Burgin and K. C. Rice, Quantification of staphylococcus aureus biofilm formation by crystal violet and confocal microscopy. Humana, New York, Methods in Molecular Biology: Staphylococcus aureus, 2021, 2341, 69–78 Search PubMed.
- F. He, Z. Y. Ye, L. D. Zhao, B. C. Yin and B. C. Ye, Probing exosome internalization pathways through confocal microscopy imaging, Chem. Commun., 2019, 55, 14015–14018 RSC.
- L. A. Bernardez and L. R. P. de Andrade Lima, Improved method for enumerating sulfate-reducing bacteria using optical density, MethodsX, 2015, 2, 249–255 CrossRef CAS PubMed.
- P. F. Xu, Z. H. Liu, Y. H. Duan, Q. Sun, D. Wang, X. F. Zeng and J. X. Wang, Microfluidic controllable synthesis of monodispersed sulfur nanoparticles with enhanced antibacterial activities, Chem. Eng. Sci., 2020, 398, 125293 CrossRef CAS.
- T. Baygar, N. Sarac, A. Ugur and I. RanaKaraca, Antimicrobial characteristics and biocompatibility of the surgical sutures coated with biosynthesized silver nanoparticles, Bioorg. Chem., 2019, 86, 254–258 CrossRef CAS PubMed.
- S. Vasso, J. N. Barreto and P. K. Tosh, Emerging issues in Gram-negative bacterial resistance: an update for the practicing clinician, Mayo Clin. Proc., 2015, 90, 395–403 CrossRef PubMed.
- K. S. Ong, Y. L. Cheow and S. M. Lee, The role of reactive oxygen species in the antimicrobial activity of pyochelin, J. Adv. Res., 2017, 8(4), 393–398 CrossRef CAS PubMed.
- M. Hanif, F. Jabbar, S. Sharif, G. Abbas, A. Farooq and M. Aziz, Halloysite nanotubes as a new drug-delivery system: a review, Clay Miner., 2016, 51(3), 469–477 CrossRef CAS.
Footnote |
† Electronic supplementary information (ESI) available: The tables listing the used materials and equipment in the presented work. Moreover, DLS curves, EDX spectra, and the photos of disk counter experiments on the products have been given in this section. The LC-MS results of the synthesized peptide sequence have been reported, as well. See DOI: 10.1039/d1ra07821f |
|
This journal is © The Royal Society of Chemistry 2021 |
Click here to see how this site uses Cookies. View our privacy policy here.