DOI:
10.1039/D0SC06112C
(Edge Article)
Chem. Sci., 2021,
12, 3050-3054
A reduced-dimensional polar hybrid perovskite for self-powered broad-spectrum photodetection†
Received
6th November 2020
, Accepted 2nd January 2021
First published on 4th January 2021
Abstract
Polar hybrid perovskites have been explored for self-powered photodetection benefitting from prominent transport of photo-induced carriers and the bulk photovoltaic effect (BPVE). However, these self-powered photodetection ranges are relatively narrow depending on their intrinsic wide bandgaps (>2.08 eV), and the realization of broad-spectrum self-powered photodetection is still a difficult task. Herein, we successfully obtained a polar multilayered perovskite, (I-BA)2(MA)2Pb3I10 (IMP, MA+ = methylammonium and I-BA+ = 4-iodobutylammonium), via rational dimension reduction of CH3NH3PbI3. It features the narrowest bandgap of 1.71 eV in a BPV material. As a consequence, the integration of narrow bandgap and BPVE causes the self-powered photodetection to extend to 724 nm for IMP, and a repeatable photovoltaic current reaching 1.0 μA cm−2 is acquired with a high “on/off” ratio of ∼103 and photodetectivity (∼109 Jones) at zero bias. This innovative research provides a foothold for adjusting the physical properties of hybrid perovskites and will expand their potential for self-powered broad-spectrum detection.
Introduction
Self-powered photoelectric detection is of great significance to next-generation miniature and cost-effective photoelectric devices.1–8 Self-powered photodetection based on the bulk photovoltaic effect (BPVE) of polar materials has attracted intense attention recently.9,10 Compared to the traditional self-powered photodetectors constructed by p–n junctions or Schottky barriers,11,12 BPVE induced self-powered photodetection eliminates the complicated interface engineering and fabrication process.13,14 Notably, self-powered visible-blind ultraviolet photodetection depending on the BPVE has been realized in BaTiO3, (K0.5Na0.5)–(Mn0.005Nb0.995)O3, and La-doped Pb(Zr,Ti)O3.15–17 However, these inorganic oxides suffer from wide bandgaps (>2.7 eV) and low concentrations of photo-induced carriers, which limit their potential detection range. In this context, it is very intriguing and still challenging to acquire broad-spectrum candidates with the BPVE for high-performance self-powered photodetection.
Currently, three-dimensional (3D) CH3NH3PbI3 perovskite displaying broad-band absorption and high carrier mobility has shown great promise for solar cell and photodetection applications.18–20 But its high structural symmetry leads to the lack of BPVE. Owing to the fact that there is no built-in electric field to facilitate carrier separation and transport, it can only work well with an applied external power source or based on a p–i–n structure.21–23 Thus, the rational introduction of symmetry breaking to achieve the BPVE in hybrid perovskites is essential.24,25 Benefitting from the structural compatibility and tunability of hybrid perovskites, Xiong's group has designed low-symmetry polar two-dimensional (2D) hybrid perovskites, such as (4,4-difluorocyclohexylammonium)2PbI4 and [R- and S-1-(4-chlorophenyl)ethylammonium]2PbI4,26,27 by employing large organic cations. They feature interesting ferroelectricity with intrinsic BPVEs. Furthermore, the BPVE was successfully achieved in chiral-polar layered lead-iodide perovskites (S/R-MPA)2(MA)Pb2I7 designed via introducing chiral organic cations.28 However, they still possess relatively wide bandgaps (>2.08 eV). It is well known that the inorganic layer is one of the determinants of the bandgap of a hybrid perovskite,29–33 and thus a multilayered I-based perovskite with a polar structure is promising to acquire a narrow bandgap toward broad-spectrum self-powered photodetection. In addition, the increase of the inorganic layer is expected to benefit the transport of photo-induced carriers, which results in high performance photodetection.34–36
Here, we present a new Ruddlesden–Popper 2D hybrid perovskite, (I-BA)2(MA)2Pb3I10 (IMP, MA+ = methylammonium, and I-BA+ = 4-iodobutylammonium), which is fabricated by the dimension reduction of the 3D prototype hybrid perovskite CH3NH3PbI3. It is noteworthy that IMP exhibits a wide absorption extending to 724 nm, corresponding to a narrow optical bandgap of ∼1.71 eV, close to the 1.50 eV of CH3NH3PbI3.37 Moreover, the polar structure enables the BPVE in IMP. Taking advantage of these above distinct performances, self-powered broad-spectrum photodetection with a large zero-bias photocurrent of 1.0 μA cm−2 was obtained with a high “on/off” ratio of ∼103 and photodetectivity (∼109 Jones) at zero bias. To the best of our knowledge, IMP features the narrowest bandgap in BPV materials for promising self-powered photodetection.
Results and discussion
Structure description
Crystals of IMP were acquired from concentrated HI solution through a temperature cooling process. The measured PXRD patterns of the IMP are similar to those of the simulated results, which indicates that the synthesized IMP is a pure phase. Crystals exposed to the ambient atmosphere after a month present identical PXRD patterns to those of the original sample, revealing that IMP is environmentally stable (Fig. S1†). Single crystal X-ray diffraction reveals that IMP crystallizes in the monoclinic system with a polar space group of Pc at room temperature (Table S1†). The observed second harmonic generation further confirms the polar structure (Fig. S2†). As shown in Fig. 1a, IMP adopts a Ruddlesden–Popper 2D trilayered architecture with [Pb3I10]∞ inorganic sheets. The organic MA+ cations reside in the cavities formed by the corner-sharing PbI6 octahedra, and I-BA+ is the “spacer” that is confined in the interlayer space of the inorganic sheets. Finally, the organic I-BA+ cations link with the infinite trilayer via N–H⋯I hydrogen bonds to form the 3D network (Fig. S3†). It's worth noting that both confined MA+ and I-BA+ cations are ordered at room temperature in IMP, and are different to MA+ cations in the typical 3D perovskites that are highly disordered and random in the cavities. Besides, the configuration of PbI6 octahedra is distorted, inferred from the inhomogeneous Pb–I bond lengths (3.0490–3.3334 Å) and I–Pb–I bond angles (86.12–95.19°), as shown in Tables S2 and S3.† As a result, the combination of ordered organic cations and distorted PbI6 octahedra leads to the polar structure for IMP. The molecular dipole moment and spontaneous polarization were calculated by using the point electric charge model.38,39 The spontaneous polarization of IMP is estimated to be 1.51 μC cm−2 along the a-axis and 3.62 μC cm−2 along the c-axis, respectively (ESI†).
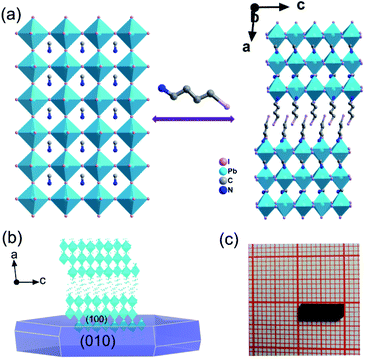 |
| Fig. 1 (a) Trimming three-dimensional CH3NH3PbI3 to design a 2D structure of IMP viewed along the b-axis. (b) Typical growth morphology for crystals of IMP. (c) Solution-grown bulk crystal of IMP with dimensions of 9.0 × 3.0 × 1.5 mm3. | |
Semiconducting performance
The above trilayered perovskite is expected to present striking photoelectric performance benefitting from the transport of photo-excited carriers in the inorganic layer.40,41 Large single crystals with sizes up to 9 × 3 × 1.5 mm3 can be obtained (Fig. 1c), and the crystal morphology is consistent with the simulated result (Fig. 1b). To evaluate the potential of the IMP single crystal for photodetection, its optical and semiconducting properties were studied. Firstly, solid-state ultraviolet-visible (UV-Vis) diffuse reflectance spectroscopy was performed at room temperature. As shown in Fig. 2a, the UV-Vis absorption spectrum of IMP displays a broad absorption cutoff at 724 nm, corresponding to a narrow bandgap of 1.71 eV, which is obviously smaller than those of (S/R-MPA)2(MA)Pb2I7 (2.08 eV) and (4,4-difluorocyclohexylammnium)2PbI4 (2.38 eV), demonstrating its potential in broad-spectrum self-powered photodetection. Meanwhile, the electronic structure of IMP was studied via first-principles density functional theory (DFT). As shown in Fig. 2b, both the conduction band minimum and the valence band maximum are localized at the B point, indicating a direct bandgap feature of IMP. The calculated bandgap value of 1.63 eV is slightly smaller than the experimental value (1.71 eV), due to the limitation of the DFT methods.42 The partial density of states of IMP was further analyzed, and it is found that the Pb-6p orbit offers the conduction band minimum while the inorganic framework I-5p orbit dominates the valence band maximum (Fig. S4†). Hence, it is proposed that the inorganic framework determines the optical bandgap and energy structure of IMP, consistent with the highest occupied molecular orbital (HOMO) and lowest unoccupied molecular orbital (LUMO). As revealed in Fig. 2c, electrons in the highest occupied molecular orbital (HOMO) are almost around the I atom, while those in the LUMO mainly disperse around the Pb atom. Besides, temperature-dependent conductivity of IMP further confirms its typical semiconducting characteristic (Fig. S5†). Finally, the charge transport properties of IMP were investigated based on the space charge limited current method (Fig. 2d).43,44 The trap density (ntraps) was calculated to be 5.21 × 1010 cm−3 according to ntraps = 2εε0VTFL/ed2. Such value is much smaller than those of traditional inorganic semiconductors (CdTe: 1011 to 1013 cm−3,45 Si: 1013 to 1014 cm−3,46etc.), and is comparable to that of reported high-quality MAPbI3 (ntraps = 3.3 × 1010 cm−3),47 demonstrating its potential for high-performance photoresponse.
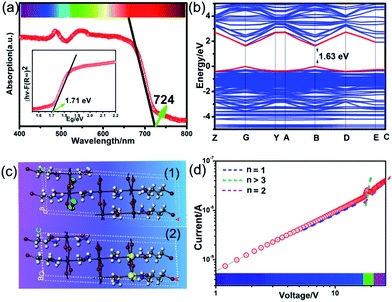 |
| Fig. 2 (a) UV-Vis absorption spectrum for IMP. Inset: calculated bandgap of IMP. (b) Theoretically calculated band structure of IMP. (c) The calculated charge density isosurfaces for HOMO (1) and LUMO (2) orbitals. (d) Logarithmic I–V characteristics of IMP based on the space charge limited current method. | |
Photovoltaic performance and photodetection
The voltage dependent current (I–V curve) was measured on high-quality single crystals of IMP. The electrodes were attached to the [001] direction of the single crystal, paralleling the polarization orientation of the c-axis. Strikingly, the BPVE was clearly observed under irradiation at 637 nm (Fig. 3a). Moreover, the photocurrent density increases directly with the increase of incident light intensity, originating from the enhanced photo-induced carriers. Under an incident light intensity of 50.6 mW cm−2, an open-circuit photovoltage of ∼0.15 V is acquired (Fig. 3b), and a spontaneous short-circuit photocurrent reaching up to 1.0 μA cm−2 is generated (Fig. 3c). This short-circuit photocurrent is higher than that of reported active self-powered photodetectors, such as BiFeO3 (0.4 μA cm−2)48 and (Pb,La)(Zr,Ti)O3 (∼4.0 nA cm−2).17 Furthermore, the time-dependent photocurrent response of the single-crystal photodetector shows no obvious attenuation after multiple cycles, indicating that the BPV in IMP is stable under illumination to realize high-performance self-powered photodetection. Taking advantage of the low trap density in the high-quality single crystal, the IMP device exhibits extremely low dark current (∼4 × 10−12 A), leading to a high “on/off” ratio of ∼103 under illumination (50.6 mW cm−2), and the detectivity is estimated to be 1.25 × 109 Jones by formula (1). |  | (1) |
where Jph is the photocurrent, Jd is the dark current, Llight is the incident light intensity, and q is the elementary charge. The time-resolved photoresponse of IMP was studied at zero bias. As shown in Fig. 3d, the rise time and fall time were found to be 165 μs and 220 μs (Fig. 3d), which are shorter than those of other reported self-powered photodetectors, such as 0.2 s for EA4Pb3Cl10 and 0.25 s for ZnS,49,50 demonstrating the great potential of IMP for future high-speed self-powered detection. In addition, considering the broad-spectrum absorption of IMP, the photoelectric performances under 405 nm, 520 nm and 700 nm were further investigated. The BPVEs were also observed with notable short-circuit photocurrent (Fig. S6 and S7†). These results indicate that IMP is highly promising in broad-spectrum self-powered photodetection.
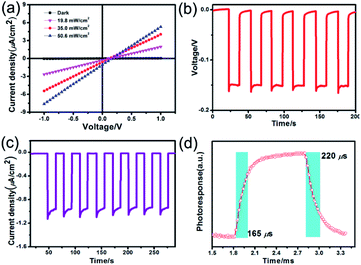 |
| Fig. 3 (a) Current–voltage characteristics along polarization directions of IMP with different incident power (with a 637 nm laser). (b) Reproducible bulk photovoltaic on/off switching. (c) Reproducible photocurrent on/off switching at zero bias. (d) The rise and fall of the photocurrent at zero bias. | |
Photovoltaic mechanism
To reveal the relationship between BPVEs and polar structure, variable-temperature structures of IMP were analysed. It is found that IMP crystallizes in a centrosymmetric space group of C2/m at 385 K, indicating a structural phase transition accompanying the symmetry breaking during temperature increase (Fig. 4a and b). Variable-temperature PXRDs of the sample were further performed to confirm the reversible phase transition. As shown in Fig. S8,† some PXRD patterns change obviously accompanying the structural phase transition after heating to 395 K, which recover after cooling to room temperature. The presence of a pair of thermal peaks at temperatures of 356 K and 364 K during differential scanning calorimetry measurements further confirms the phase transition (Fig. 4c). Most importantly, the temperature-dependent photovoltage shows a gradual decline with increasing temperature, and disappears completely above 364 K, revealing that the BPVEs in IMP depend on the spontaneous polarization in the structure at room temperature (Fig. 4d). At 385 K, the organic cations become disordered and the inorganic layers exhibit a highly symmetric configuration, which endow IMP with a centrosymmetric space group without spontaneous polarization. In order to further confirm that the BPVEs originate from spontaneous polarization, the BPVEs along the a-axis and b-axis were also studied (Fig. S9†). As expected, an obvious BPVE was observed along the a-axis, while no signal appeared along the b-axis with a gliding plane.
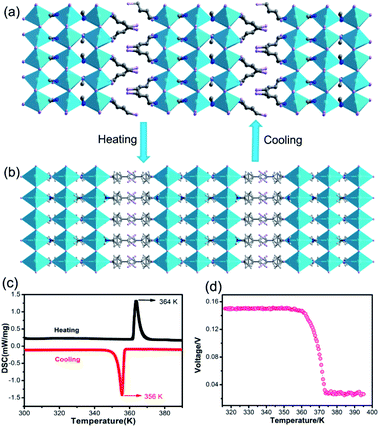 |
| Fig. 4 (a) Structure of IMP at 290 K. (b) Structure of IMP at 385 K. (c) DSC curves in heating and cooling runs for IMP. (d) Temperature-dependent photovoltage of IMP. | |
Conclusions
In summary, we developed a polar trilayered hybrid perovskite via dimension reduction of 3D MAPbI3. This I-based trilayered hybrid perovskite enables a broad spectrum absorption extending to 724 nm, corresponding to a narrow optical bandgap of ∼1.71 eV. Strikingly, benefiting from the intrinsic polar feature, BPVE was acquired with an open-circuit voltage of 0.15 V and a short-circuit current density of 1.0 μA cm−2 under 637 nm illumination. Moreover, the high-quality crystal device of IMP exhibits a high “on/off” ratio of ∼103 and photodetectivity (∼109 Jones) at zero bias. These features make IMP a promising candidate for the self-powered broad-spectrum detection. This work provides a foothold for developing high-performance self-powered photodetectors in emerging application fields.
Author contributions
D. Li prepared the samples and wrote the manuscript. W. T. Wu and S. G. Han carried out the structure characterization. X. T. Liu and D. Li analyzed the photoelectric properties. Y. Peng, X. Q. Li, M. C. Hong and J. H. Luo provided suggestions for the project. L. N. Li designed and directed this project. All the authors discussed and commented on the manuscript.
Conflicts of interest
There are no conflicts to declare.
Acknowledgements
This work was supported by the National Natural Science Foundation of China (21971238, 21833010, 21525104, 21875251, 21975258, 61975207 and 21921001), the Key Research Program of Frontier Sciences of the Chinese Academy of Sciences (ZDBS-LY-SLH024), the NSF of Fujian Province (2018H0047), the Strategic Priority Research Program of the Chinese Academy of Sciences (XDB20010200) and Youth Innovation Promotion of CAS.
Notes and references
- Y. Zhang, X. Xu and X. Fang, InfoMat, 2019, 1, 542–551 CrossRef CAS.
- L. Peng, L. Hu and X. Fang, Adv. Funct. Mater., 2014, 24, 2591–2610 CrossRef CAS.
- S. Park, S. W. Heo, W. Lee, D. Inoue, Z. Jiang, K. Yu, H. Jinno, D. Hashizume, M. Sekino, T. Yokota, K. Fukuda, K. Tajima and T. Someya, Nature, 2018, 561, 516–521 CrossRef CAS.
- S. Xu, Y. Qin, C. Xu, Y. Wei, R. Yang and Z. L. Wang, Nat. Nanotechnol., 2010, 5, 366–373 CrossRef CAS.
- D. K. Singh, R. Pant, A. M. Chowdhury, B. Roul, K. K. Nanda and S. B. Krupanidhi, ACS Appl. Electron. Mater., 2020, 2, 944–953 CrossRef CAS.
- D. Wu, J. Guo, J. Du, C. Xia, L. Zeng, Y. Tian, Z. Shi, Y. Tian, X. J. Li, Y. H. Tsang and J. Jie, ACS Nano, 2019, 13, 9907–9917 CrossRef CAS.
- M. Dai, H. Chen, F. Wang, M. Long, H. Shang, Y. Hu, W. Li, C. Ge, J. Zhang, T. Zhai, Y. Fu and P. Hu, ACS Nano, 2020, 14, 9098–9106 CrossRef CAS.
- L. H. Zeng, Q. M. Chen, Z. X. Zhang, D. Wu, H. Yuan, Y. Y. Li, W. Qarony, S. P. Lau, L. B. Luo and Y. H. Tsang, Adv Sci., 2019, 6, 1901134 CrossRef CAS.
- X. Liu, S. Wang, P. Long, L. Li, Y. Peng, Z. Xu, S. Han, Z. Sun, M. Hong and J. Luo, Angew. Chem., Int. Ed., 2019, 58, 14504–14508 CrossRef CAS.
- Y. Peng, X. Liu, Z. Sun, C. Ji, L. Li, Z. Wu, S. Wang, Y. Yao, M. Hong and J. Luo, Angew. Chem., Int. Ed., 2020, 59, 3933–3937 CrossRef CAS.
- M. Peng, Y. Liu, A. Yu, Y. Zhang, C. Liu, J. Liu, W. Wu, K. Zhang, X. Shi, J. Kou, J. Zhai and Z. L. Wang, ACS Nano, 2016, 10, 1572–1579 CrossRef CAS.
- Y. Bie, Z. Liao, H. Zhang, G. Li, Y. Ye, Y. Zhou, J. Xu, Z. Qin, L. Dai and D. Yu, Adv. Mater., 2011, 23, 649–653 CrossRef CAS.
- Q. Wang, C. Zhou and Y. Chai, Nanoscale, 2020, 12, 8109–8118 RSC.
- F. Cao, W. Tian, L. Meng, M. Wang and L. Li, Adv. Funct. Mater., 2019, 29, 1808415 CrossRef.
- J.-k. Li, C. Ge, K.-j. Jin, J.-y. Du, J.-t. Yang, H.-b. Lu and G.-z. Yang, Appl. Phys. Lett., 2017, 110, 142901 CrossRef.
- J. Park, S. S. Won, C. W. Ahn, I. W. Kim and G. L. Brennecka, J. Am. Chem. Soc., 2013, 96, 146–150 CAS.
- J. Zhang, X. Su, M. Shen, Z. Shen, L. Zhang, X. Zhang, W. Cheng, M. Cao and G. Zou, Sci. Rep., 2013, 3, 2109 CrossRef.
- D. P. McMeekin, G. Sadoughi, W. Rehman, G. E. Eperon, M. Saliba, M. T. Hoerantner, A. Haghighirad, N. Sakai, L. Korte, B. Rech, M. B. Johnston, L. M. Herz and H. J. Snaith, Science, 2016, 351, 151–155 CrossRef CAS.
- N. J. Jeon, H. Na, E. H. Jung, T.-Y. Yang, Y. G. Lee, G. Kim, H.-W. Shin, S. I. Seok, J. Lee and J. Seo, Nat. Energy, 2018, 3, 682–690 CrossRef CAS.
- Y. Fang, Q. Dong, Y. Shao, Y. Yuan and J. Huang, Nat. Photonics, 2015, 9, 679–686 CrossRef CAS.
- X. Zheng, H. Lei, G. Yang, W. Ke, Z. Chen, C. Chen, J. Ma, Q. Guo, F. Yao, Q. Zhang, H. Xu and G. Fang, Nano Energy, 2017, 38, 1–11 CrossRef CAS.
- Y. Zhang, Y. Liu, Z. Yang and S. Liu, J. Energy Chem., 2018, 27, 722–727 CrossRef.
- F. Bai, J. Qi, F. Li, Y. Fang, W. Han, H. Wu and Y. Zhang, Adv. Mater. Interfaces, 2018, 5, 1701275 CrossRef.
- C. Ji, D. Dey, Y. Peng, X. Liu, L. Li and J. Luo, Angew. Chem., Int. Ed., 2020, 59, 18933–18937 CrossRef CAS.
- P.-F. Li, Y.-Y. Tang, W.-Q. Liao, H.-Y. Ye, Y. Zhang, D.-W. Fu, Y.-M. You and R.-G. Xiong, NPG Asia Mater., 2017, 9, e342 CrossRef CAS.
- T. T. Sha, Y. A. Xiong, Q. Pan, X. G. Chen, X. J. Song, J. Yao, S. R. Miao, Z. Y. Jing, Z. J. Feng, Y. M. You and R. G. Xiong, Adv. Mater., 2019, 31, e1901843 CrossRef.
- C. K. Yang, W. N. Chen, Y. T. Ding, J. Wang, Y. Rao, W. Q. Liao, Y. Y. Tang, P. F. Li, Z. X. Wang and R. G. Xiong, Adv. Mater., 2019, 31, e1808088 CrossRef.
- P. J. Huang, K. Taniguchi and H. Miyasaka, J. Am. Chem. Soc., 2019, 141, 14520–14523 CrossRef CAS.
- S. Han, P. Wang, J. Zhang, X. Liu, Z. Sun, X. Huang, L. Li, C. Ji, W. Zhang, B. Teng, W. Hu, M. Hong and J. Luo, Laser Photonics Rev., 2018, 12, 1800060 CrossRef.
- H. Y. Zhang, Z. Wei, P. F. Li, Y. Y. Tang, W. Q. Liao, H. Y. Ye, H. Cai and R. G. Xiong, Angew. Chem., Int. Ed., 2018, 57, 526–530 CrossRef CAS.
- W. Q. Liao, Y. Zhang, C. L. Hu, J. G. Mao, H. Y. Ye, P. F. Li, S. D. Huang and R. G. Xiong, Nat. Commun., 2015, 6, 7338 CrossRef.
- S. Han, X. Liu, Y. Liu, Z. Xu, Y. Li, M. Hong, J. Luo and Z. Sun, J. Am. Chem. Soc., 2019, 141, 12470–12474 CrossRef CAS.
- I. Spanopoulos, I. Hadar, W. Ke, Q. Tu, M. Chen, H. Tsai, Y. He, G. Shekhawat, V. P. Dravid, M. R. Wasielewski, A. D. Mohite, C. C. Stoumpos and M. G. Kanatzidis, J. Am. Chem. Soc., 2019, 141, 5518–5534 CrossRef CAS.
- A. K. Jena, A. Kulkarni and T. Miyasaka, Chem. Rev., 2019, 119, 3036–3103 CrossRef CAS.
- H. Tsai, W. Nie, J. C. Blancon, C. C. Stoumpos, R. Asadpour, B. Harutyunyan, A. J. Neukirch, R. Verduzco, J. J. Crochet, S. Tretiak, L. Pedesseau, J. Even, M. A. Alam, G. Gupta, J. Lou, P. M. Ajayan, M. J. Bedzyk and M. G. Kanatzidis, Nature, 2016, 536, 312–316 CrossRef CAS.
- X. Li, W. Ke, B. Traore, P. Guo, I. Hadar, M. Kepenekian, J. Even, C. Katan, C. C. Stoumpos, R. D. Schaller and M. G. Kanatzidis, J. Am. Chem. Soc., 2019, 141, 12880–12890 CrossRef CAS.
- W.-J. Yin, T. Shi and Y. Yan, Appl. Phys. Lett., 2014, 104, 063903 CrossRef.
- S. Wang, X. Liu, L. Li, C. Ji, Z. Sun, Z. Wu, M. Hong and J. Luo, J. Am. Chem. Soc., 2019, 141, 7693–7697 CrossRef CAS.
- L. Li, X. Liu, C. He, S. Wang, C. Ji, X. Zhang, Z. Sun, S. Zhao, M. Hong and J. Luo, J. Am. Chem. Soc., 2020, 142, 1159–1163 CrossRef CAS.
- C. Chen, L. Gao, W. Gao, C. Ge, X. Du, Z. Li, Y. Yang, G. Niu and J. Tang, Nat. Commun., 2019, 10, 1927 CrossRef.
- J. C. Blancon, H. Tsai, W. Nie, C. C. Stoumpos, L. Pedesseau, C. Katan, M. Kepenekian, C. M. M. Soe, K. Appavoo, M. Y. Sfeir, S. Tretiak, P. M. Ajayan, M. G. Kanatzidis, J. Even, J. J. Crochet and A. D. Mohite, Science, 2017, 355, 1288–1291 CrossRef CAS.
- L. Mao, P. Guo, M. Kepenekian, I. Spanopoulos, Y. He, C. Katan, J. Even, R. D. Schaller, R. Seshadri, C. C. Stoumpos and M. G. Kanatzidis, J. Am. Chem. Soc., 2020, 142, 8342–8351 CrossRef CAS.
- G. González, J. Appl. Phys., 2015, 117, 084306 CrossRef.
- I. Muzikante and E. A. Silinsh, Acta Phys. Pol., A, 1995, 88, 389–399 CrossRef CAS.
- A. Balcioglu, R. K. Ahrenkiel and F. Hasoon, J. Appl. Phys., 2000, 88, 7175–7178 CrossRef CAS.
- I. Capan, V. Borjanović and B. Pivac, Sol. Energy Mater. Sol. Cells, 2007, 91, 931–937 CrossRef CAS.
- D. Shi, V. Adinolfi, R. Comin, M. Yuan, E. Alarousu, A. Buin, Y. Chen, S. Hoogland, A. Rothenberger, K. Katsiev, Y. Losovyj, X. Zhang, P. A. Dowben, O. F. Mohammed, E. H. Sargent and O. M. Bakr, Science, 2015, 347, 519–522 CrossRef CAS.
- P. Poosanaas, A. Dogan, S. Thakoor and K. Uchino, J. Appl. Phys., 1998, 84, 1508–1512 CrossRef CAS.
- S. Wang, L. Li, W. Weng, C. Ji, X. Liu, Z. Sun, W. Lin, M. Hong and J. Luo, J. Am. Chem. Soc., 2020, 142, 55–59 CrossRef CAS.
- D. Li, S. Hao, G. Xing, Y. Li, X. Li, L. Fan and S. Yang, J. Am. Chem. Soc., 2019, 141, 3480–3488 CrossRef CAS.
Footnote |
† Electronic supplementary information (ESI) available. CCDC 2033297 and 2033299. For ESI and crystallographic data in CIF or other electronic format see DOI: 10.1039/d0sc06112c |
|
This journal is © The Royal Society of Chemistry 2021 |
Click here to see how this site uses Cookies. View our privacy policy here.