DOI:
10.1039/D1SC02918E
(Edge Article)
Chem. Sci., 2021,
12, 13283-13291
Aggregation-induced delayed fluorescence luminogens: the innovation of purely organic emitters for aqueous electrochemiluminescence†
Received
30th May 2021
, Accepted 5th September 2021
First published on 7th September 2021
Abstract
Due to overcoming the limitation of aggregation caused quenching (ACQ) of solid-state emitters, aggregation-induced emission (AIE) organic luminogens have become a promising candidate in aqueous electrochemiluminescence (ECL). However, restricted by the physical nature of fluorescence, current organic AIE luminogen-based ECL (AIECL) faces the bottleneck of low ECL efficiency. Here, we propose to construct de novo aqueous ECL based on aggregation-induced delayed fluorescence (AIDF) luminogens, called AIDF-ECL. Compared with the previous organic AIE luminogens, purely organic AIDF luminogens integrate the superiorities of both AIE and the utilization of dark triplets via thermal-activated spin up-conversion properties, thereby possessing the capability of close-to-unity exciton utilization for ECL. The results show that the ECL characteristics using AIDF luminogens are directly related to their AIDF properties. Compared with an AIECL control sample based on a tetraphenylethylene AIE moiety, the ECL efficiency of our AIDF-ECL model system is improved by 5.4 times, confirming the excellent effectiveness of this innovative strategy.
Introduction
Electrochemiluminescence (ECL),1–5 also termed as electrogenerated chemiluminescence, is essentially related to excited state generation via electrochemical reaction in the vicinity of an electrode. In addition to fascinating ECL light-emitting devices6,7 and weavable fibre applications,8 ECL is well recognized in analytical chemistry, life and environmental sciences due to its multiple advantages as compared to chemiluminescence (CL) or photoluminescence (PL) methods, such as ultralow background signal, high sensitivity, multi-dimensional electrochemical regulation capability, etc. Very recently, aggregation-induced emission (AIE), a concept launched by Ben Zhong Tang and co-workers,9,10 has been introduced into the ECL field as a new-generation ECL emitter.11–19 Impressively, by using the AIE luminogens, the ubiquitous aggregation caused quenching (ACQ) issue originating from notorious π–π interactions and/or molecular-motion-induced energy dissipation has been well solved via the AIE effect.10 For AIE, the water-insolubility of organic molecules is not a drawback, but actually becomes a premise and framework for constructing self-aggregated powder, film or crystalline solid-state emitters featuring much-enhanced PL quantum efficiency (ΦPL). Coincidentally, this property is extremely satisfactory for the development of a qualified aqueous ECL emitter, which is the essential demand of the whole ECL technique towards various kinds of applications.2,20
Starting from the pioneer report on a platinum(II) complex in 2017 by De Cola's group,11 a variety of aggregation-induced electrochemiluminescence (AIECL) emitters have emerged, such as metallic complexes,18,21,22 metal clusters,23 AIE organic molecules,12,14–16,24 carbon dots17 or polymer dots.13,25 Meanwhile, ECL bioscience sensing studies using these emitters have also been developed, e.g. a chloramphenicol sensor,26 methyltransferase assay,27 microRNA assay28etc. However, a main challenge of the AIECL motif is the low ECL efficiency. At present, the main strategy is to improve the solid-state ΦPL of AIE emitters either by chemistry or physics methods.13–16,25 However, under electrochemical excitation, the basic charge-to-photon conversion processes that determine the ECL efficiency (ΦECL) of the AIECL system have rarely been considered.23 It was noticed that, among the AIECL emitters, the reported metallic complex or cluster AIECL samples generally outperformed the others in terms of ΦECL.11,22,23 With the assistance of the heavy-atom effect from Au, Ru or Ir metals, these AIECL systems correspond to phosphorescence in photophysics. Under electrochemical excitation, all of the generated excited states (R*) (under spin statistics,29 the R* ratios between the lowest singlets (S1) and triplets (T1) in nature are close to 25%/75%) were radiatively decayed via a phosphorescent route. By contrast, without exception, the ΦECL behaviours of all of the purely organic AIECL emitters12–16,25,30 were limited by their fluorescence properties. Despite the high ΦPL achieved in the aggregated state, it was only the singlet excited states (1R*) (∼25% in total) that were harvested for ECL via the fluorescent route under electrochemical excitation, while the dominant triplet excited states (3R*) (∼75% in total) were wasted via a nonradiative decay channel. Previously, Ishimatsu et al. reported efficient ECL of donor–acceptor molecules with thermally activated delayed fluorescence (TADF) properties.31 Owing to the small S1/T1 energy gap (ΔEST) and high reverse intersystem crossing (RISC) efficiency (ΦRISC), the process of spin up-conversion from the T1 to S1 ensured the utilization of triplets generated by electrochemical excitation. In dichloromethane (DCM) medium, such TADF-type ECL renders a high ΦECL at about 50%, which was comparable to its ΦPL in DCM solution. Accordingly, it is reliable to construct efficient ECL of purely organic systems to break the rules of spin statistics. Unfortunately, owing to the water-insolubility and ACQ effects of these TADF emitters,31,32 such a mechanism is invalid in an aqueous medium. For aqueous ECL using purely organic luminophores, organic luminophores simultaneous possessing AIE and spin up-conversion properties are an ideal candidate. However, there have been no such reports so far.
Herein, we report that aggregation-induced delayed fluorescence (AIDF) luminogens33,34 have great potential to develop efficient and stable aqueous ECL. We called this new system AIDF-ECL as this phenomenon is intrinsically based on the AIDF properties of this kind of luminogen. Considering its combination of the merits of AIE and TADF features, AIDF-ECL will be an ideal system to build aqueous ECL using purely organic luminophores. Surprisingly, a lot of efficient AIDF luminogens with different colours have been successfully explored for organic light-emitting diodes (OLEDs),33–36 while their superiorities of AIDF properties in an aqueous medium suitable for aqueous ECL have not been considered. To our best knowledge, this work is the first attempt. As a proof of concept, we selected one AIDF luminogen and presented the direct correlation between its AIDF property and its ECL behaviours. One tetraphenylethylene (TPE)-moiety-containing AIE luminogen without AIDF properties was used as a control sample, which represents all ever-reported purely organic AIECL systems12–16,22,24,30 that possess traditional fluorescence properties. Importantly, with a similar ΦPL, the AIDF luminogen displayed an obvious enhancement in ΦECL with respect to that of the control AIE luminogen sample. Our results provide important clues for the further development and application of this new class of aqueous ECL luminophore.
Experimental section
Materials
All the chemicals and reagents were purchased from commercial sources and used as received without further purification. Ultrapure fresh water was obtained from a water purification system (>18.2 MΩ). Potassium chloride (KCl), potassium hydrogen phosphate (KH2PO4) and dipotassium phosphate (K2HPO4) were purchased from Sinopharm Chemical Reagent Co. Ltd (Shanghai, China). Acetonitrile (ACN, anhydrous, 99.8%) and tetrahydrofuran (THF, ≥99.9%) were purchased from Sigma-Aldrich Co. Ltd (Shanghai, China). Tripropylamine (TPrA, ≥98%) was purchased from Alfa Co. Ltd. Dichloromethane (DCM, 99.9%) was purchased from Innochem Co. Ltd (Beijing, China). Tetra-n-butylammonium hexafluorophosphate (TBAPF6, 98%) was purchased from Acros Co. Ltd. The AIDF-type mCP-BP-PXZ (C49H31N3O2) molecule and the AIE-type TPE-TAPBI (C67H56N2) molecule were synthesized according to our previously reported paper.36,37 All the aqueous ECL measurements used phosphate buffered solution (PBS, pH = 7.44) containing 0.1 M KCl, 0.1 M K2HPO4 and 0.1 M KH2PO4 as the electrolyte.
General characterization
For the photophysical characterization, an Edinburgh fluorescence spectrometer (FLS1000) was used, in which transient PL and steady-state PL measurements were triggered by a picosecond pulsed LED (EPLED-365) and Xe2 xenon lamp, respectively. For direct measurement of the absolute PL quantum efficiency (PLQY) of solutions or films, integrating sphere testing accessories were coupled, which were integrated with the whole FLS1000 system and controlled by the same software. Various kinds of photophysical parameters (e.g. shown in Tables S1–S3†) were calculated according to the previous established theory.36,38,39 Redox electrochemistry experiments were conducted on a CHI 660B electrochemistry workstation (CH Instruments Inc). ECL measurements were carried out on a Xi'an Remex MPI-EII ECL system. Transmission Electron Microscopy (TEM) measurements were obtained with a JEOL JEM-2100F system operated at 200 kV.
The preparation of AIDF or AIE luminogen solutions
At first, we prepared the mCP-BP-PXZ or TPE-TAPBI solutions (5 mM in concentration) in THF as the mother solution for subsequent blending or dilution. The next step is to add additional THF and/or ultrapure fresh water into these mother solutions to fabricate mCP-BP-PXZ (0.1 mM) or TPE-TAPBI (0.1 mM) luminogen solutions in a THF/H2O blend solvent with different water factions as required, i.e. fw: 0–95 vol%. To prevent agglomeration or heterogeneity, the as-prepared luminogen solutions were thoroughly shaken in general, and then used for the subsequent characterization or experiments (e.g. modifying the GCE electrode for CV and ECL test).
The glassy carbon electrode (GCE) modification method
Prior to usage, the GCE (4 mm in diameter) was routinely polished by using 0.3 μm and 0.05 μm alumina slurry, respectively, which was followed by successive sonication in water, ethanol and ultrapure fresh water in sequence. Then, the GCE electrode was thoroughly rinsed with ultrapure water and dried using high-purity N2 flow. Finally, the bare GCE was dip-coated into the mCP-BP-PXZ (20 μL, 0.1 mM) or TPE-TAPBI (20 μL, 0.1 mM) luminogen solutions in THF/H2O mixtures with different water fractions, and dried by baking it with an infrared lamp in ambient conditions to obtain the AIDF-luminogen- or AIE-luminogen-modified GCE samples.
CV and ECL measurements
The CV experiments were tested on a CHI 660B electrochemistry workstation (CH Instruments Inc). Generally, a three-electrode system (a Pt wire as a counter electrode, a Ag wire or Ag/AgCl as a reference electrode, and a GCE or AIDF-luminogen-modified GCE or AIE-luminogen-modified GCE with a diameter of 4 mm as a working electrode) was used, which was calibrated by using a ferrocene (Fc)/ferrocenium (Fc+) couple. CVs of mCP-BP-PXZ or TPE-TAPBI in solution (1 mM) were tested in waterless dichloromethane (DCM) (for the anode scan) or acetonitrile (ACN) (for the cathode scan) with 0.1 M TBAPF6 as a supporting electrolyte under a nitrogen-filled glovebox environment and scan rate of 0.1 V s−1.
Electrochemiluminescence (ECL) was tested on a MPI-EII electrochemiluminescence (Remex Electronic Instrument Lt. Co., Xi'an, China) detection system. In all aqueous ECL measurement experiments, a three-electrode system (Pt wire as a counter electrode, Ag/AgCl (saturation KCl solution) as a reference electrode, and an AIDF-luminogen-modified or AIE-luminogen-modified GCE with a diameter of 4 mm as a working electrode) was used, and the photomultiplier tube (PMT) voltage of the ECL system was set at 850 V. In detail, the luminogen modified GCE was immersed in PBS solution containing 0.1 M KCl, 0.1 M K2HPO4 and 0.1 M KH2PO4 as the electrolyte for ECL tests. Co-reactant ECL studies were conducted using the same procedures except for additionally adding 40 mM TPrA into the PBS solution. The relative ECL efficiency of the mCP-BP-PXZ luminogen modified GCE/TPrA was calculated using the TPE-TAPBI luminogen modified GCE/TPrA as the internal standard. In detail, the relative ECL efficiencies were calculated using the following equation:30,40
in which,
Φ0ECL is the ECL efficiency of the TPE-TAPBI modified GCE (the pre-aggregation solution conditions: 0.1 mM,
fw = 95%) in PBS solution with 40 mM TPrA, which was taken as 100% as the internal reference.
I,
i and
x are ECL intensity, current value, and the targeted emitter,
i.e. the mCP-BP-PXZ modified GCE (the pre-aggregation solution conditions: 0.1 mM,
fw = 95%) in PBS solution with 40 mM TPrA, respectively.
Results and discussion
Photophysical properties
Organic ECL emitters, except for some peculiar cases, e.g. triplet–triplet annihilation (TTA)-type,29 can be divided into three kinds according to their excited state decay dynamics (Scheme 1A), i.e. fluorescent type (FL-ECL),41 AIE-ECL (i.e. AIECL)14–16 and AIDF-ECL (mentioned herein). Similar to electroluminescent (EL) excitation conditions,42–44 the produced yield of S1/T1 excited states under electrochemical excitation is about 25%/75% under spin statistics.29,31 Despite the merit of the AIE effect of restraining various non-radiative (NR) routes (see the comparison between FL-ECL and AIE-ECL in Scheme 1A), the ever-explored organic AIE-ECL emitters belong to fluorescence in photophysics. Under electrochemical excitation, the dominantly generated triplet excited states (75% in total) cannot be harvested for ECL, which is limited by a distinct high ΔEST level (>0.5–1.0 eV) and thus inefficient RISC process. For the AIDF luminogens in the aggregated state, a distinctly reduced ΔEST (<0.1–0.3 eV) and the favorable AIE effect are coupled to facilitate spin up-conversion from those triplets to singlets via RISC, and then radiative decay via a delayed fluorescent (DF) route. Accordingly, the harvesting of triplets is realized through the delayed fluorescence (DF)-ECL channel (Scheme 1A, bottom). In practice, a qualified AIDF molecule, namely mCP-BP-PXZ,36 was applied in this work (see Scheme 1C for its chemical structure) to construct this novel AIDF-ECL system. When dissolved in the good solvent tetrahydrofuran (THF), its PL emission is extremely weak. After adding poor solvent water at a high content, self-aggregation occurs via the AIDF effect (see dashed box shown in Scheme 1B and also the followed discussion for its physics reason), along with sharply enhanced PL emission (Scheme 1B). For the control AIE-ECL system, the fluorescent-type AIE molecule, e.g. TPE-TAPBI (Scheme 1C for its chemical structure),37 was used in this work. Noticeably, the desired DF-ECL process is absent for the AIE-ECL sample (Scheme 1A, middle). The intense DF via a fast and efficient RISC process is the peculiar photophysical basis of the AIDF-ECL strategy to be discussed.
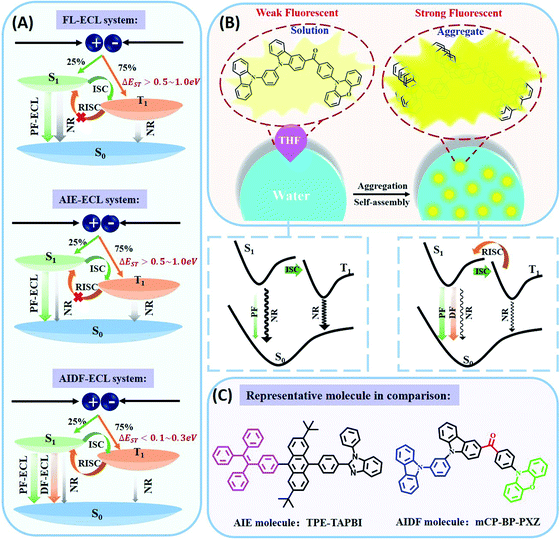 |
| Scheme 1 (A) Schematic illustration of the fluorescent-type ECL (FL-ECL), aggregation-induced emission-type ECL (AIE-ECL) and aggregation-induced delayed fluorescence (AIDF)-type ECL (AIDF-ECL) and their mechanisms, in which S1, T1, S0, PF-ECL, DF-ECL, IS, RISC and NR represent the lowest singlet state, the lowest triplet state, the ground singlet state, prompt fluorescent ECL, delayed fluorescent ECL, intersystem crossing, reverse intersystem crossing, and nonradiative deactivation, respectively. (B) Preparation of the AIDF aggregated luminogens by self-assembly in a mixed solvent. Inside the dashed box are the schematic diagrams of photophysical transitions in the molecular state in THF solution or the aggregated solid state in a THF/H2O mixed solvent. (C) Chemical structures of AIE (left) and AIDF (right) molecules used in this report. | |
To begin with, we introduce the peculiar difference in AIE phenomena with or without AIDF properties. Steady-state PL (Fig. 1A and B) and transient PL (Fig. 1C and D) studies were performed on these two molecules in THF/water mixtures with different water contents (fw: 0–95 vol%). On increasing the fw value, the PL peak intensity is monotonically enhanced, e.g. increasing by 71.8-fold for mCP-BP-PXZ (fw: 0% vs. 95%, Fig. 1A and its inset picture) and 8.03-fold for TPE-TAPBI (fw: 0% vs. 90%, Fig. 1B and its inset picture). Apparently, there is no significant difference between the steady-state PL spectrum features and the visual discernible image characteristics, i.e. the PL peak, full width at half maximum (FWHM), and colours. In this sense, both of them are AIE-type luminogens. In the highly aggregated state (e.g. larger than 80% for fw), physical constraint of the molecular motion action largely lowers the nonradiative decay path for S1 to S0 transition, which contributes to the PL enhancement as observed. In particular, a distinct difference does exist in their transient PL behaviours, which is directly measured in air conditions. For mCP-BP-PXZ with the highest fw (i.e. 95% vs. 0%), except for a significantly enhanced prompt fluorescent (PF) quantum efficiency (ΦPF) (42.1% vs. 5.1%), a distinctly enhanced delayed fluorescent (DF) emission component was observed, corresponding to a sharp increase in DF quantum efficiency (ΦDF) from near zero to 20.1% and a largely increased DF exciton lifetime (τd) to 702 ns (Fig. 1C, S1 and Table S1†). These results indicated that, with the assistance of the AIE effect, both PF originating from singlets and DF originating from triplets are feasible for mCP-BP-PXZ, which stands for a typical PL transient behaviour of AIDF luminogens.34,36,45 As for the reasons, the enhancement in the RISC process plays the dominant role in the DF route of the mCP-BP-PXZ luminogen (dashed box shown in Scheme 1B), which is due to the unquenched S1 (as the NR decay rate from S1 to S0 is largely reduced now), the small ΔEST (0.024 eV) and the enhanced spin–orbit coupling (SOC) in the aggregated condition (confirmed by theoretical calculation).36 By contrast, irrespective of any aggregated condition for TPE-TAPBI, owing to the distinct large ΔEST (>0.3 eV), there is merely a PF contribution with ns-range exciton lifetimes (0.7 ns–2.7 ns) and no DF emission signals can be observed (Fig. 1D, S2 and Table S2†). For this kind of AIE luminogen, dark triplets cannot be harvested for radiative decay. In this respect, the photophysical characteristics of TPE-TAPBI stand for all ever-reported AIE-type organic ECL luminophores,13–16,23–25,30 in which the harvesting issues of triplets are not considered. Due to its chemical structure, the electron cloud distribution of the highest occupied molecular orbital (HOMO) and the lowest unoccupied molecular orbital (LUMO) is highly overlapped, rendering a distinct high ΔEST and thus the blocked RISC process for DF. For mCP-BP-PXZ and its analogous, the HOMO and LUMO distribution is deliberately separated to a large extent, e.g. by introducing a large torsion angle with phenoxazine (PXZ) for the development of mCP-BP-PXZ.36 As a result, both high ΦPL and TADF merits are simultaneously realized for the aggregated state of the AIDF luminogens (Fig. S3 and Table S1†). Thanks to these AIDF merits, non-doped OLEDs using mCP-BP-PXZ achieved an extremely high performance, e.g. maximum external quantum efficiency (EQE) value at 22.1%.36 In parallel, non-doped TPE-TAPBI-based OLEDs merely achieved a peak EQE of 5.73%.37 Under EL excitation with spin statistics restriction, the generation ratio of singlets/triplets is also ∼25%/75%. The EQE ratio of them is about 4
:
1, which implies the significance of collection of triplets for AIDF-type OLEDs.
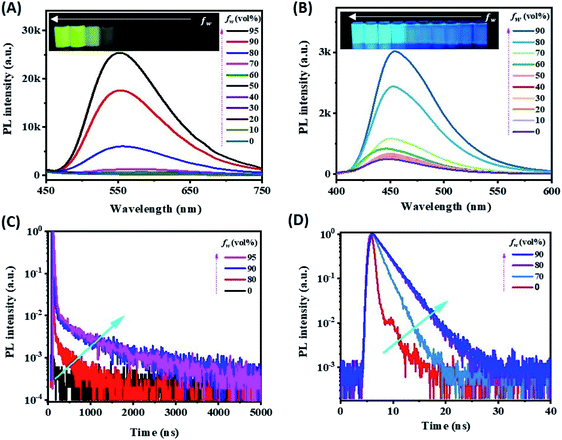 |
| Fig. 1 PL spectra of the (A) mCP-BP-PXZ and (B) TPE-TAPBI molecule in THF/water mixtures with different water fractions (fw). Inset: the corresponding photographs of the luminogens in THF/water mixtures under UV irradiation (λ = 365 nm). The transient PL decay curves of the (C) mCP-BP-PXZ molecule with different fw (excitation wavelength: 363 nm, and detection wavelength: 553 nm, in air) at 300 K, and the (D) TPE-TAPBI molecule with different fw (excitation wavelength: 363 nm, and detection wavelength: 454 nm, in air) at 300 K. | |
Electrochemistry and electrochemiluminescence
For electrochemical study, glassy carbon electrodes (GCEs) were modified with such AIDF molecules by dip-coating in those solutions and then drying at ambient conditions (see Experimental section for the details). Their photographs under UV irradiation (λ = 365 nm) (Fig. S4†) are analogous to the inset pictures shown in Fig. 1A. Similar to the solution condition (Fig. 1A), the mCP-BP-PXZ modified GCE is the brightest when the fw is 95%. Accordingly, it is speculated that pre-aggregation occurs in the corresponding solution and directly determines the photophysical behaviour of the as-fabricated dip-coated AIDF luminogen-modified GCE. As measured by transmission electron microscopy (TEM) (Fig. S5†), the corresponding mCP-BP-PXZ dip-coated film (fw: 95%) has the morphology of nanoaggregates, in which disk-shaped aggregates with diameters of about 50–100 nm are fused together. Under N2 protection, its transient PL behaviours are comparable to the values measured in the solution state, i.e. τp/τd: 19/877 ns (Fig. S6, S7 and Table S3†), well confirming the AIDF property for the dip-coated film. As compared to the N2-protected film sample, it is observed that the DF emission of the mCP-BP-PXZ film is lowered in air (Fig. S6†), which is due to the triplet quenching of oxygen.46 However, the lowered degree was significantly less than that of previous TADF emitters.31 The exact reason is still under study. As a possible reason, firstly, the fast RISC rate (2.1 × 106 s−1) in such aggregated state may play an important role, which guarantees the DF route. In addition, it may be attributed to the unique AIE properties of the mCP-BP-PXZ luminogen, in which the triplet quenching by oxygen could be inhibited by molecular conformational factors, such as the restricted molecular motion. As measured, the resultant mCP-BP-PXZ dip-coated film in air possesses the τp/τd of 17 ns/453 ns and ΦPL of 28.3%, in which ΦPF and ΦDF are 15.6% and 12.7%, respectively (Table S3†).
First, we tried an annihilation ECL test for the mCP-BP-PXZ-modified GCE sample. Unfortunately, no ECL was observed for it. The reason is probably limited by the stability of free radicals generated in the annihilation test conditions. It has been reported that in the ECL mode, the stable intermediate states formed by the co-reactant assist ECL luminophores to generate excitons, and then produce significantly enhanced ECL.47 Herein, considering its satisfactory electrooxidation behaviour (i.e. a reversible and stable anodic half-wave current–potential cyclic voltammetry (CV) curve, Fig. S9†), we attempted to conduct oxidative-reduction ECL using conventional tripropylamine (TPrA)48,49 as the co-reactant, under the conditions of a potential window of 0 V–1.3 V (vs., Ag/AgCl), scan rate of 0.5 V s−1, 0.1 M PBS containing 0.1 M KCl and 40 mM TPrA, and pH 7.44. As shown in Fig. 2A (taking fw = 95% as an example), the current starts to rise rapidly from about 0.74 V, while the ECL signal does not start to rise until after 0.97 V, and then reaches the peak value at 1.28 V (Fig. 2B). For the bare GCE containing TPrA alone in the electrolytic cell, extremely weak ECL is produced (Fig. S10†). For the AIDF-luminogen-modified GCE, ECL was also not observed in the absence of TPrA (not shown here). Therefore, the corresponding emission mechanism should be a TPrA-involved co-reactant ECL mode.15,25 In detail, during the electrooxidation process, the AIDF luminogens (AIDF gen) are first oxidized to generate cationic radicals on the GCE electrode (AIDF gen˙+). On further increasing the oxidation potential, TPrA molecules are oxidized to produce a sufficient amount of strong reductive intermediates, i.e. TPrA˙, so as to collide with the AIDF gen˙+ to generate excitons on mCP-BP-PXZ (AIDF gen*), and then produce ECL (Fig. 3A). As for the ECL spectrum (inset graph in Fig. 2C), the emission peak (596 nm) displayed a 53 nm redshift with respect to its PL spectrum (543 nm). The exact reason is still unclear. To understand it, we noticed that a similar distinct redshift phenomenon was also observed for the AIECL of carboranyl carbazole nanoaggregates in the aggregated state.16 As suggested, in view of the morphology-related ECL spectra, the surface state transition may be involved, which is analogous to the well-known redshift phenomena typically occurring in semiconductor nanomaterial ECL emitters.50
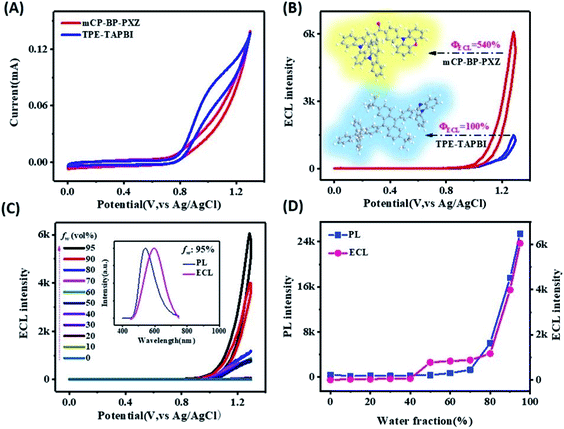 |
| Fig. 2 (A) Anodic cyclic voltammograms and (B) the oxidative-reduction ECL responses of the AIDF molecule (red line, fw: 95%) and AIE molecule (blue line, fw: 95%). (C) The oxidative-reduction ECL responses of the AIDF luminogen-modified GCE under the conditions of a potential window ranging from 0 V to 1.3 V (vs. Ag/AgCl), scan rate of 0.5 V s−1, 0.1 M PBS containing 0.1 M KCl and 40 mM TPrA, and pH 7.44. PMT: 850 V. Inset: PL and ECL spectrum of the AIDF luminogen in the solid state (fw: 95%). (D) PL and ECL trends of the AIDF luminogen with different fw. | |
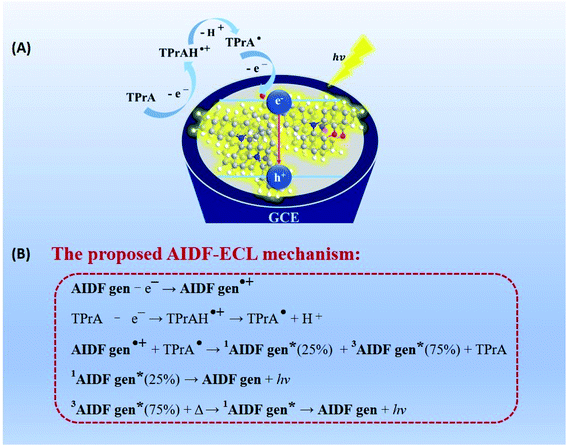 |
| Fig. 3 (A) The schematic subprocesses of oxidative-reduction ECL of the AIDF-luminogen-modified GCE/TPrA couple and (B) the proposed ECL mechanisms, in which 1AIDF gen* and 3AIDF gen* represent singlet and triplet excited states that were electrochemically generated on AIDF luminogens, respectively. | |
At the same time, we observed that the ECL intensity increased with the increase of water content fw (0–95%) of the AIDF luminogens that were dispersed for pre-aggregation. The reason can be mainly attributed to two aspects as we observed: (i) the oxidation current was gradually increased on increasing the fw (Fig. S10†). Therefore, under the same potential, it is likely that more free AIDF radical cations (AIDF gen˙+) are generated, which could collide with the reductive intermediates of TPrA (TPrA˙) to generate more excitons (AIDF gen*, either singlets (25% in total) or triplets (75% in total) in excitonic nature), and then radiative decay via ECL. On increasing the water fraction (fw) of the mCP-BP-PXZ solution, intermolecular arrangement of AIDF luminogens in the solid state might be enhanced, which would promote the charge transport properties of the mCP-BP-PXZ-modified GCE working electrode. (ii) The exciton radiative decay efficiency of the mCP-BP-PXZ-modified GCE electrode increases with the increase of fw. As shown in Fig. 2C and D, the variation trend of the ECL intensity is basically consistent with that of the PL intensity of the corresponding mCP-BP-PXZ luminogens. Since the AIDF effect of mCP-BP-PXZ luminogens is monotonically enhanced on increasing the fw (Fig. 1A and C), the achieved ECL intensity–potential behaviours of the AIDF sample shown in Fig. 2C are reasonable, which is an intrinsic characteristic of the AIDF-ECL scaffold.
As for evaluating of ECL efficiency, it is difficult to make direct comparisons between our solid-state AIDF luminogen-modified GCE/TPrA and the standard liquid-phase Ru(bpy)32+/TPrA reference, since they have different concentrations of ECL emitters, and different dynamic factors, such as radical/ion diffusion and migration rate. To confirm the effectiveness of the AIDF property on the ECL efficiency of AIE-type luminogens, a TPE-TAPBI-modified GCE was used as the control condition (Fig. S11†), since it was confirmed to be an AIE luminogen without AIDF properties (mentioned above). As shown in Fig. 2A and B, we compared the behaviours of CV and co-reactant ECL for the mCP-BP-PXZ and TPE-TAPBI luminogens in the same preparation conditions. Using a routinely relative ECL efficiency calculating method (see Experimental section)30,40 and taking the ECL efficiency of the TPE-TAPBI-modified GCE/TPrA sample as a reference (set as 100%), the relative ECL efficiency of our AIDF-ECL sample is calculated as 540%. The electrochemical currents of the two systems are basically comparable (Fig. 2A and Table S5†). The content of co-reactant TPrA is also the same (40 mM), so its effect on the electrochemical current is also similar. Therefore, the number of cationic radicals and intermediates of TPrA produced by such oxidative-reduction ECL mode should be comparable. The difference in relative ECL efficiency thus mainly comes from the difference in ECL intensity. Although the ΦPL values of the two samples are similar (28.3% and 34.0%, measured in air) (Table S6†), the ECL efficiency of the AIDF-ECL sample is greatly improved considering that AIDF samples can effectively utilize triplet excitons generated by electrochemical excitation. Possible ECL mechanisms of AIDF luminogens are thus schematically listed in Fig. 3B. On the basis of the AIE framework, it is shown that AIDF-ECL has a significant superiority for aqueous ECL with much higher efficiency. Under electrochemical excitation, it not only inhibits ACQ in an aqueous medium via self-aggregation, but the AIDF property means that it has the intrinsic capability to fully utilize singlet and triplet excitons for radiative decay during the ECL test.
Finally, we also briefly evaluated the stability of AIDF luminogens for aqueous ECL test conditions. Through 50 cycles of stability tests, we found that under good AIDF pre-aggregation conditions (e.g. fw: 95%–80%), the ECL intensity of mCP-BP-PXZ luminogens is basically not changed (Fig. S12 and S13†). Also, at a wide concentration range of TPrA from 80 mM to 200 mM for real sensing applications, the ECL stability is also excellent (Fig. S14†). This indicates that the ECL decay dynamic behaviours of AIDF luminogens are stable under aqueous electrochemical conditions. AIDF luminogens, e.g. mCP-BP-PXZ used herein, were qualified as a potential candidate for efficient, reliable and reversible ECL.
Conclusions
In conclusion, an innovation within the AIECL topic is described. Compared with all of the developed AIECL organic luminophores, the model molecule that we studied has unique AIDF photophysical characteristics, namely the synergism of AIE characteristics and triplet-to-singlet spin up-conversion by thermal activation. Using one conventional AIECL model emitter (i.e. TPE-TAPBI) as a control, the ECL efficiency is improved by 5.4 times by using one AIDF-ECL model emitter (i.e. mCP-BP-PXZ), while both of them have similar PL efficiency (ΦPL: 34.0% vs. 28.3%). This demonstrates the great potential of AIDF organic luminogens in achieving much higher aqueous ECL efficiency as compared to the earlier suggested AIE organic luminogen analogues. However, ECL study in aqueous media using AIDF luminogens is still in its infancy. The next step will be to focus on further disclosing the related mechanisms in-depth, so as to accelerate its development in the fascinating ECL field.
Data availability
Supporting data for this article have been uploaded as part of the ESI† material.
Author contributions
B.-H. Zhang and L. Niu conceived the idea. B.-H. Zhang designed the experiments and wrote the manuscript. Z.-J. Zhao and B.-Z. Tang designed the synthesis of the AIE materials. H.-J. Liu and B. Chen synthesized the AIE materials. Y. Kong performed the corresponding photophysical, electrochemistry and electrochemiluminescence experiments. B.-L. Zhao, Y.-L. Luo, L.-J. Chen, Y.-W. Zhang and D.-X. Han assisted with the data collection and analysis. B.-H. Zhang, Z.-J. Zhao and Y. Kong assisted with the manuscript revision.
Conflicts of interest
There are no conflicts to declare.
Acknowledgements
This work was supported by the foundations from the Research & Development Projects in Key Areas of Guangdong Province, China (2019B010933001), the National Natural Science Foundation of China (51773195), the Natural Science Foundation of Guangdong Province (2019B030301003, 2021A1515010510, 2021B1515020048) and Guangzhou Science and Technology Plan Project (202102010409, 202102010390, 202102010487). We highly appreciate Miss S.X. Song for her professional CV test and data analysis during the revision stage of this work.
Notes and references
- M. M. Richter, Chem. Rev., 2004, 104, 3003–3036 CrossRef CAS PubMed.
- W. J. Miao, Chem. Rev., 2008, 108, 2506–2553 CrossRef CAS PubMed.
- A. Fiorani, G. Valenti, M. Iurlo, M. Marcaccio and F. Paolucci, Curr. Opin. Electrochem., 2018, 8, 31–38 CrossRef CAS.
- Y. Q. Chen, S. Quan, F. X. Du, B. H. Lou, J. P. Li and G. B. Xu, Chin. J. Anal. Chem., 2021, 49, 849–857 Search PubMed.
- X. Ma, W. Gao, F. Du, F. Yuan, J. Yu, Y. Guan, N. Sojic and G. Xu, Acc. Chem. Res., 2021, 54, 2936–2945 CrossRef CAS PubMed.
- S. Lee, H. J. Lee, Y. Ji, K. H. Lee and K. Hong, Adv. Mater., 2021, 33, 2005456 CrossRef CAS PubMed.
- K. Schlingman, Y. Chen, R. S. Carmichael and T. B. Carmichael, Adv. Mater., 2021, 33, 2006863 CrossRef CAS PubMed.
- Z. T. Zhang, K. P. Guo, Y. M. Li, X. Y. Li, G. Z. Guan, H. P. Li, Y. F. Luo, F. Y. Zhao, Q. Zhang, B. Wei, Q. B. Pei and H. S. Peng, Nat. Photonics, 2015, 9, 233–238 CrossRef CAS.
- J. D. Luo, Z. L. Xie, J. W. Y. Lam, L. Cheng, H. Y. Chen, C. F. Qiu, H. S. Kwok, X. W. Zhan, Y. Q. Liu, D. B. Zhu and B. Z. Tang, Chem. Commun., 2001, 1740–1741 RSC.
- J. Mei, N. L. C. Leung, R. T. K. Kwok, J. W. Y. Lam and B. Z. Tang, Chem. Rev., 2015, 115, 11718–11940 CrossRef CAS PubMed.
- S. Carrara, A. Aliprandi, C. F. Hogan and L. De Cola, J. Am. Chem. Soc., 2017, 139, 14605–14610 CrossRef CAS PubMed.
- H. W. Liu, L. F. Wang, H. F. Gao, H. L. Qi, Q. Gao and C. X. Zhang, ACS Appl. Mater. Interfaces, 2017, 9, 44324–44331 CrossRef CAS PubMed.
- Z. Wang, Y. Feng, N. Wang, Y. Cheng, Y. Quan and H. Ju, J. Phys. Chem. Lett., 2018, 9, 5296–5302 CrossRef CAS PubMed.
- Z. Han, Z. Yang, H. Sun, Y. Xu, X. Ma, D. Shan, J. Chen, S. Huo, Z. Zhang, P. Du and X. Lu, Angew. Chem., Int. Ed., 2019, 58, 5915–5919 CrossRef CAS PubMed.
- J. L. Liu, J. Q. Zhang, Z. L. Tang, Y. Zhuo, Y. Q. Chai and R. Yuan, Chem. Sci., 2019, 10, 4497–4501 RSC.
- X. Wei, M.-J. Zhu, Z. Cheng, M. Lee, H. Yan, C. Lu and J.-J. Xu, Angew. Chem., Int. Ed., 2019, 58, 3162–3166 CrossRef CAS PubMed.
- J. R. Adsetts, S. Hoesterey, C. Gao, D. A. Love and Z. Ding, Langmuir, 2020, 36, 14432–14442 CrossRef CAS PubMed.
- L. P. Lu, L. L. Zhang, W. J. Miao, X. Y. Wang and G. S. Guo, Anal. Chem., 2020, 92, 9613–9619 CrossRef CAS PubMed.
- Z. Wang, J. Pan, Q. Li, Y. Zhou, S. Yang, J.-J. Xu and D. Hua, Adv. Funct. Mater., 2020, 30, 2000220 CrossRef CAS.
- W. Lv, H. Ye, Z. Yuan, X. Liu, X. Chen and W. Yang, TrAC, Trends Anal. Chem., 2020, 123, 115767 CrossRef CAS.
- S. Carrara, B. Stringer, A. Shokouhi, P. Ramkissoon, J. Agugiaro, D. J. D. Wilson, P. J. Barnard and C. F. Hogan, ACS Appl. Mater. Interfaces, 2018, 10, 37251–37257 CrossRef CAS PubMed.
- H. Gao, N. Zhang, Y. Li, W. Zhao, Y. W. Quan, Y. X. Cheng, H. Y. Chen and J. J. Xu, Sci. China: Chem., 2020, 63, 715–721 CrossRef CAS.
- H. P. Peng, Z. N. Huang, H. H. Deng, W. H. Wu, K. Y. Huang, Z. L. Li, W. Chen and J. W. Liu, Angew. Chem., Int. Ed., 2020, 59, 9982–9985 CrossRef CAS PubMed.
- Y. Zhang, Y. Zhao, Z. Han, R. Zhang, P. Du, Y. Wu and X. Lu, Angew. Chem., Int. Ed., 2020, 59, 23261–23267 CrossRef CAS PubMed.
- S. Y. Ji, W. Zhao, H. Gao, J. B. Pan, C. H. Xu, Y. W. Quan, J. J. Xu and H. Y. Chen, iScience, 2020, 23, 100774 CrossRef CAS PubMed.
- S. Li, X. Ma, C. Pang, M. Wang, G. Yin, Z. Xu, J. Li and J. Luo, Biosens. Bioelectron., 2021, 176, 112944 CrossRef CAS PubMed.
- L. Cui, M.-H. Zhao, C.-C. Li, Q. Wang, X. Luo and C.-Y. Zhang, Anal. Chem., 2021, 93, 2974–2981 CrossRef CAS PubMed.
- J. L. Liu, Y. Zhuo, Y. Q. Chai and R. Yuan, Chem. Commun., 2019, 55, 9959–9962 RSC.
-
A. J. Bard, Electrogenerated Chemiluminescence, Marcel Dekker, Inc., 2004 Search PubMed.
- L. Cui, S. Yu, W. Gao, X. Zhang, S. Deng and C.-Y. Zhang, ACS Appl. Mater. Interfaces, 2020, 12, 7966–7973 CrossRef CAS PubMed.
- R. Ishimatsu, S. Matsunami, T. Kasahara, J. Mizuno, T. Edura, C. Adachi, K. Nakano and T. Imato, Angew. Chem., Int. Ed., 2014, 53, 6993–6996 CrossRef CAS PubMed.
- P. Huang, B. Zhang, Q. Hu, B. Zhao, Y. Zhu, Y. Zhang, Y. Kong, Z. Zeng, Y. Bao, W. Wang, Y. Cheng and L. Niu, ChemPhysChem, 2021, 22, 726–732 CrossRef CAS PubMed.
- J. J. Guo, Z. J. Zhao and B. Z. Tang, Adv. Opt. Mater., 2018, 6, 1800264 CrossRef.
- R. Furue, T. Nishimoto, I. S. Park, J. Lee and T. Yasuda, Angew. Chem., Int. Ed., 2016, 55, 7171–7175 CrossRef CAS PubMed.
- H. J. Kim, M. Godumala, S. K. Kim, J. Yoon, C. Y. Kim, H. Park, J. H. Kwon, M. J. Cho and D. H. Choi, Adv. Opt. Mater., 2020, 8, 1902175 CrossRef CAS.
- H. J. Liu, J. J. Zeng, J. J. Guo, H. Nie, Z. J. Zhao and B. Z. Tang, Angew. Chem., Int. Ed., 2018, 57, 9290–9294 CrossRef CAS PubMed.
- B. Chen, B. Q. Liu, J. J. Zeng, H. Nie, Y. Xiong, J. H. Zou, H. L. Ning, Z. M. Wang, Z. J. Zhao and B. Z. Tang, Adv. Funct. Mater., 2018, 28, 1803369 CrossRef.
- Y. Tao, K. Yuan, T. Chen, P. Xu, H. Li, R. Chen, C. Zheng, L. Zhang and W. Huang, Adv. Mater., 2014, 26, 7931–7958 CrossRef CAS PubMed.
- X. Lin, Y. Zhu, B. Zhang, X. Zhao, B. Yao, Y. Cheng, Z. Li, Y. Qu and Z. Xie, ACS Appl. Mater. Interfaces, 2018, 10, 47–52 CrossRef CAS PubMed.
- F. Wang, J. Lin, T. Zhao, D. Hu, T. Wu and Y. Liu, J. Am. Chem. Soc., 2016, 138, 7718–7724 CrossRef CAS PubMed.
- H. Qi, C. Zhang, Z. Huang, L. Wang, W. Wang and A. J. Bard, J. Am. Chem. Soc., 2016, 138, 1947–1954 CrossRef CAS PubMed.
- S. Wang, H. Zhang, B. Zhang, Z. Xie and W.-Y. Wong, Mater. Sci. Eng., R, 2020, 140, 100547 CrossRef.
- M. A. Baldo, D. F. O'Brien, M. E. Thompson and S. R. Forrest, Phys. Rev. B, 1999, 60, 14422–14428 CrossRef CAS.
- D. F. O. B. M. A. Baldo, Y. You, A. Shoustikov, M. E. T. S. Sibley and S. R. Forrest, Nature, 1998, 395, 151 CrossRef.
- J. Huang, H. Nie, J. Zeng, Z. Zhuang, S. Gan, Y. Cai, J. Guo, S.-J. Su, Z. Zhao and B. Z. Tang, Angew. Chem., Int. Ed., 2017, 56, 12971–12976 CrossRef CAS PubMed.
- R. Ishimatsu, Y. Kirino, C. Adachi, K. Nakano and T. Imato, Chem. Lett., 2016, 45, 1183–1185 CrossRef CAS.
- A. Zanut, A. Fiorani, S. Canola, T. Saito, N. Ziebart, S. Rapino, S. Rebeccani, A. Barbon, T. Irie, H. P. Josel, F. Negri, M. Marcaccio, M. Windfuhr, K. Imai, G. Valenti and F. Paolucci, Nat. Commun., 2020, 11, 2668 CrossRef CAS PubMed.
- Q. Li, J. Y. Zheng, Y. L. Yan, Y. S. Zhao and J. N. Yao, Adv. Mater., 2012, 24, 4745–4749 CrossRef CAS PubMed.
- L. Wu, J. S. Wang, L. Y. Feng, J. S. Ren, W. L. Wei and X. G. Qu, Adv. Mater., 2012, 24, 2447–2452 CrossRef CAS PubMed.
- P. Wu, X. Hou, J.-J. Xu and H.-Y. Chen, Chem. Rev., 2014, 114, 11027–11059 CrossRef CAS PubMed.
Footnote |
† Electronic supplementary information (ESI) available: Additional photophysics, electrochemical and electrochemiluminescence data and the correlated analysis. See DOI: 10.1039/d1sc02918e |
|
This journal is © The Royal Society of Chemistry 2021 |
Click here to see how this site uses Cookies. View our privacy policy here.