DOI:
10.1039/D1SC03428F
(Edge Article)
Chem. Sci., 2021,
12, 11730-11734
Hollow and highly diastereoselective face-rotating polyhedra constructed through rationally engineered facial units†
Received
24th June 2021
, Accepted 19th July 2021
First published on 23rd July 2021
Abstract
Molecular face-rotating polyhedra (FRP) exhibit complex stereochemistry, rendering it challenging to manipulate their assembly in a stereoselective manner. In our previous work, stereocontrolled FRP were gained at the cost of losing the confined inner space, which hampers their host–guest interactions and potential applications. Through a rational design approach, herein we demonstrate the successful construction of hollow FRP with high diastereoselectivity. Whereas the [4 + 4] imine condensation of meta-formyl substituted C3h-symmetric TAT-m and C3-symmetric Tri-NH2 led to the formation of all feasible FRP-12 diastereoisomers; the para-substituted constitutional isomer, TAT-p, exclusively assembled into a pair of homo-directional enantiomeric FRP-13-CCCC/AAAA with a cavity size larger than 600 Å3. Detailed structural characterizations and theoretical investigations revealed the thermodynamic landscape of FRP assembly can be effectively shaped by modulating the van der Waals repulsive forces among the facial building blocks. Our work provided a novel strategy towards stereospecific assembly of pure organic cages, opening up new opportunities for further applications of these chiral materials.
Introduction
Rationally designed discrete molecular cages have drawn extensive attention in recent years on account of their wide applications in gas storage,1 molecular recognition,2 separation,1b,3 catalysis,4 and containment of reactive materials.5 By fine-tuning the shape, angle or rigidity associated with the molecular building blocks,6 the geometry and size of the confined space inside the resulting cages can be precisely engineered for specific functions. Introducing handedness into molecular cages further lends a new dimension to asymmetric catalysis7 and chiral resolution.8 Nevertheless, manipulating the outcome of cage syntheses, i.e. diastereoselectivity, is a challenging task as a result of the minute differences between various diastereoisomers on the thermodynamic landscape. In literature, successful chirality control over coordination-driven assembly can be achieved by regulating the chiral communications among ligands.9 For instance, by modulating the steric repulsions among ligand edges, Nitschke et al. reported the syntheses of a series of metal–organic cages with different degrees of diastereoselectivity.10 On the other hand, introducing chiral guests may also enable the chirality transfer to the cage hosts, templating the formation of specific diastereoisomers.11 In both cases, however, the intervention of extra components, e.g. metal salts and chiral small molecules, is required. As far as pure organic molecular cages are concerned, examples of stereochemical control through interfacial interactions remain rare.
Recently, our group reported a variety of novel chiral face-rotating polyhedra (FRP)12 consisting of prochiral 2D facial building blocks, such as truxene (Tr)13 and triazatruxene (TAT).14 The senses of anticlockwise (A) or clockwise (C) rotations arise when these planar building blocks lose their σh mirror symmetry upon FRP formation, generating a series of diastereoisomeric cages in the one pot reaction (Fig. S4†). The distribution of the populations of different FRP stereoisomers, instead of being statistically governed, can be biased to favor specific species when interfacial non-covalent interactions are present. For instance, the assembly of Tr-based FRP-2 solely produces the homo-directional AAAA cage as a result of the van der Waals repulsion among the interior n-butyl side chains (Fig. 1a).13a Similarly, the π–π interaction between the TAT units in FRP-9-AA exclusively leads to the formation of a homo-directional molecular prism (Fig. 1b).14 Their high diastereoselectivity, nonetheless, was gained at the cost of losing the cavity space of FRP, impeding potential applications in host–guest encapsulation. Herein, we demonstrate the successful construction of a series of [4 + 4] tetrahedral FRP with large inner cavities. In addition, by rationally designing the interfacial interactions between the facial building blocks, these FRP could be tailor-made in a highly diastereroselective fashion (Fig. 1c and S11†).
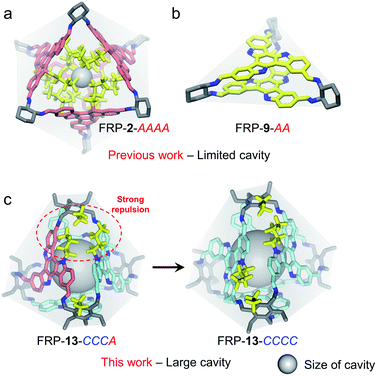 |
| Fig. 1 The van der Waals repulsion (a) and π–π interaction (b) between facial units lead to homo-directional FRP at the cost of inner cavity. (c) The location of facial interaction was moved out of FRP to maintain the cavity size and the strong repulsive force prevented the generation of hetero-directional FRP (CCCA/CAAA or CCAA), resulting in homo-directional FRP-13-CCCC/AAAA with high diastereoselectivity (the A and C patterns was distinguished by different colour. Part of hydrogen atoms and alkyl chains were omitted for clarity). | |
Results and discussion
Catalyzed by trifluoroacetic acid (0.075 eq.), the meta-formyl substituted TAT-m (1 eq.) and 1,3,5-triaminomethyl-2,4,6-triethylbenzene (Tri-NH2, 1 eq.) were co-assembled into FRP-12 in toluene through imine condensation reaction15 (Fig. 2a). High-resolution mass spectrum (HRMS) of FRP-12 showed a mass-to-charge ratio of 1586.9859, corresponding to [4 + 4] molecular cages composed of four pieces of each building blocks (calculated m/z = 1586.9868 for [M + 2H]2+, Fig. S25a†). In theory, five diastereoisomers could stem from different face-rotating patterns on the [4 + 4] tetrahedral FRP-12, i.e., homo-directional AAAA/CCCC, and hetero-directional CAAA/CCCA and CCAA (Fig. 2a and S11†). This is in perfect agreement with the fact that five distinct fractions of FRP-12 were resolved by employing high-performance liquid chromatography (HPLC) with a chiral stationary phase, respectively eluting at 14.3, 17.7, 21.4, 27.3 and 31.0 min. Preliminary assignments of these fractions can be made based on the averaged symmetry reflected in their 1H-NMR spectra.10,13a With only a single set of imine proton signals observed, the 1st/5th fractions collected were designated as the FRP-12-AAAA/CCCC isomers, which possess the highest T-symmetry. Similarly, the 2nd/4th and the 3rd fractions could be identified as the C3-symmetric FRP-12-CAAA/CCCA and S4-symmetric FRP-12-CCAA isomers, respectively (Fig. 2d and S4b†). The absolute configurations of FRP-12 were further determined based on the comparisons between the experimental and (ZINDO/S)-calculated CD spectra. By relating the magnitudes of the Cotton effects to the facial patterns (Fig. 2c and S28†),14 these five fractions were assigned as FRP-12-AAAA, CAAA, CCAA, CCCA and CCCC in the order of their elution time.
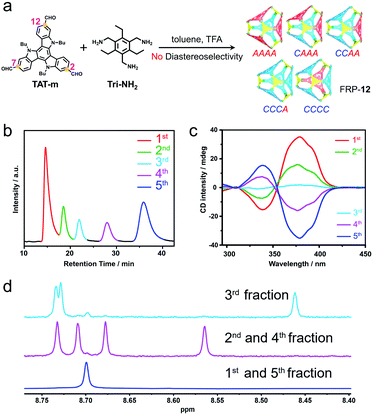 |
| Fig. 2 (a) Non-diastereoselective construction of FRP-12. (b) Chiral HPLC analysis showed that FRP-12 contain five components in 32 : 13 : 10 : 13 : 32 ratios. Detection wavelength: 335 nm. Further characterization confirmed that the configurations of these five fractions of FRP-12 are AAAA, CAAA, CCAA, CCCA and CCCC in the order of elution, respectively. (c) CD spectra of all fractions of FRP-12 recorded in toluene. (d) Partial 1H NMR spectra of FRP-12. The T-(1st and 5th fractions), C3-(2nd and 4th fraction) and S4-(3rd fraction) symmetric polyhedra exhibited one, four and three imine proton peaks, respectively (from bottom to top). | |
The assembly of FRP-12 gave rise to a complicated mixture of stereoisomeric molecular cages, whose non-trivial chiral separation inevitably impeded their further developments in both research and practical applications. In our previous work, it was shown that employing different TAT constitutional isomers as building blocks of FRP led to totally distinct geometry and diastereoselectivity.14 Inspired by the significant impact of molecular design on the FRP assembly processes, we produced the in silico structures of FRP-12 (Fig. S32†), as well as a new series of FRP-13 involving para-formyl substituted TAT-p facial units instead of TAT-m. The calculations of the relative energy levels of different diastereoisomers in both series of FRP were performed on Vienna Ab initio Simulation Package (VASP), using gradient corrected (PBE) density functional theory in vacuum with D3-BJ dispersion correction. In the case of FRP-12, the hetero-directional CCAA and CCCA/CAAA isomers were found to be 6–7 kcal mol−1 slightly less stable than their homo-directional CCCC/AAAA analogues (Fig. 3a, left). In contrast, the energy gaps between the most stable FRP-13-CCCC/AAAA enantiomeric pair and the hetero-directional isomers, namely FRP-13-CCCA/CAAA and CCAA, were predicted to be wider at 12–13 kcal mol−1 (Fig. 3a, right).
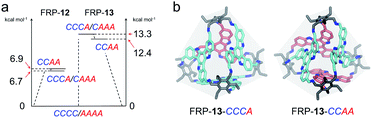 |
| Fig. 3 (a) Computationally optimized structures of FRP-12 and FRP-13 series show distinct energy profiles. The hetero-directional FRP-12-CCAA and CCCA/CAAA were found to be 6.9 and 6.7 kcal mol−1 less stable than the of homo-directional FRP-12-CCCC/AAAA analogues, respectively. On the other hand, the energy gaps between homo- and hetero-directional FRP-13 were estimated to be larger at 12.4 and 13.3 kcal mol−1, respectively. (b) The optimized structures of FRP-13-CCCA (left) and CCAA (right) (hydrogen atoms and the n-alkyl chains were omitted for clarity). | |
FRP-13in vitro were assembled from the para-formyl substituted TAT-p facial building blocks and the Tri-NH2 vertexes with a recipe similar to that of FRP-12. All structural characterizations were also carried out by following the routine. The [4 + 4] molecular topology was confirmed by HRMS (Fig. 1c and S25b†). The high symmetry of FRP-13, as revealed by the simple pattern featured in the 1H NMR spectra, corroborates the T-symmetric homo-directional CCCC/AAAA isomers (Fig. S20–S24†). The chiral HPLC analysis also showed coherent results that FRP-13 could be successfully resolved into two fractions showing antipodal CD spectra (Fig. 4a and b). The 2nd fraction exhibited a negative Cotton effect at circa 350 nm, corresponding to the calculated FRP-13-CCCC (Fig. 4b and S31†), and the 1st peak was then assigned as FRP-13-AAAA accordingly.
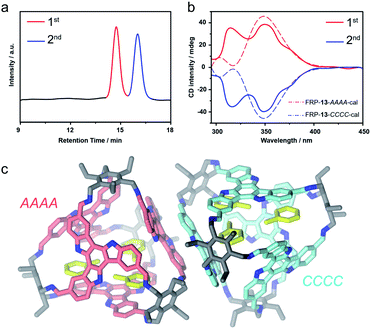 |
| Fig. 4 (a) Chiral HPLC analysis revealed that FRP-13 are consisted of two components in 1 : 1 ratio. Detection wavelength: 335 nm. Further characterizations confirmed that the directionality of the first and second fractions of FRP-13 are AAAA and CCCC, respectively. (b) Experimental and calculated (dash line) CD spectra of FRP-13 recorded in toluene. (c) Crystal structures of FRP-13-AAAA and CCCC. The toluene molecules inside the FRP are emphasized in yellow (hydrogen atoms and the n-alkyl chains were omitted for clarity). | |
In addition, single crystals of FRP-13 were cured by slowly diffusing methanol into a toluene solution. Unambiguous structural elucidation was enabled by X-ray crystallography, revealing a pair of enantiomeric FRP-13-CCCC/AAAA tetrahedral cages were co-crystalized in R
c space group (Fig. 4c). Interestingly, although FRP-13 shows a time-averaged T-symmetry in solution, in the crystal structure one of the TAT facial units shifts closer to the centroid of the cage, making the solid-state conformation of FRP-13C3-symmetric, presumably as a result of more efficient packing (Fig. S37 and S38†). Moreover, three toluene solvent molecules can be found inside of the cavity, indicating the potential for host–guest interactions. The cavity volume of FRP-13-CCCC/AAAA is estimated to be 772 Å3 by promolecular density (the cavity volume can also be calculated to be 609 Å3 according to the radius of its inscribed sphere, see in Fig. S39 and S40†). In agreement with the theoretically calculated energy levels, the experimental results of NMR, HPLC, CD and single crystal structures unanimously suggested that para-substituted TAT-p underwent highly diastereoselective assembly process with Tri-NH2 to form a pair of homo-directional enantiomeric cages out of five possible diastereoisomers.
Further structural analysis showed that the two types of facial building blocks, TAT-m and TAT-p, when assembled with Tri-NH2, cause a ∼22° difference in the facial rotational angle in the resulting FRP (Fig. S36†). This minor structural variation, coupled with the multifaceted face-rotating patterns, leads to distinct spatial arrangements of their n-butyl chains. For both FRP-12 and 13-CCCC/AAAA, the bulky n-butyl groups between their TAT faces are placed far off from each other and show little steric repulsion (Fig. S33a and c†), which is in accordance with the fact that experimentally the homo-directional stereoisomers are the most stable species obtained.
Similarly, relative weak steric interactions are expected for the hetero-directional FRP-12-CCCA/AAAC or CCAA, as their n-butyl side chains are positioned away from the Tri-NH2 vertexes in the molecular models (Fig. 5a and S33d†). The non-covalent interactions among the building blocks, which could be visualized with the aid of reduced density gradient (RDG) analysis,16 confirmed that only low-density and low-gradient regions exist between the hetero-directional TAT-m faces (Fig. 5a, right). On the other hand, in hetero-directional FRP-13-CCCA/AAAC and CCAA isomers, the alkyl chains between the facial units are located much closer to the ethyl groups of Tri-NH2 vertexes (Fig. 5b and S33b†). The strong repulsive interactions between the hetero-directional TAT-p units of FRP-13-CCCA can be clearly manifested by the RDG isosurface (Fig. 5b, right). The crowding of the imine bond protons and Tri-NH2 units, as identified by RDG analysis (Fig. S34 and S35†), also makes the formations of the hetero-directional FRP-13 highly unfavored, leaving the FRP-13-CCCC/AAAA as the sole thermodynamic products.
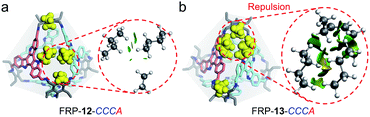 |
| Fig. 5 Computationally optimized structures of FRP-12-CCCA (a) and FRP-13-CCCA (b). The alkyl groups between hetero-directional TAT units are highlighted in yellow. The RDG analysis indicates that the repulsive interactions between hetero-directional TAT units in FRP-13-CCCA (right) are much stronger than that in FRP-12-CCCA (left) (hydrogen atoms on the FRP backbone were omitted for clarity). | |
Conclusions
In conclusion, we have successfully constructed two novel series of hollow [4 + 4] face-rotating polyhedral, FRP-12 and 13, with various degree of diastereoselectivity and large cavity. Structural characterizations and theoretical calculations indicated that the effective interfacial interactions between the TAT building blocks are essential to the outcome of the cage assembly processes. Whereas the condensation of meta-substituted TAT-m facial building blocks with Tri-NH2 vertexes formed a mixture of five FRP-12 stereoisomers, moving the formyl group on the facial units to the para-positions completely changed the picture. The minor structural tweaking resulted in ∼22° rotations of all facial units in FRP-13, bringing out clashing alkyl–alkyl interactions between TAT-p units, as well as the unfavored gauche conformations of the imine linkages, in the FRP with hetero-directional configurations. On account of the large energy gaps between the hetero- and homo-directional diastereoisomers, FRP-13 were obtained as a pair of CCCC/AAAA enantiomers exclusively. The highly diastereo-selective assembly of FRP-13 provides an example of stereo-controlled construction of pure organic cages by rational molecular design. This work clearly revealed the mechanism for the generation of highly diastereoselective FRP with large inner voids, opening up future opportunities for the constructions of more diverse optically-pure FRP and the further research in chiral resolution, asymmetric catalysis and bio-recognition applications.
Data availability
All associated computational and experimental details are provided in the ESI.†
Author contributions
X.-Y. Cao, X.-C. Wang, X. Tang and Andrew C.-H. Sue conceived, initiated and developed this work. X. Tang synthesized the face-rotating polyhedra and participated in all the data analysis. K. Tan and Z.-H. Li performed the theoretical calculation and RDG analysis. H.-L. Liu and S.-L. Zhang carried out the chiral HPLC and CD analysis. Z.-H. Li and W.-B. Gao performed the MS and NMR characterizations. X. Dong and W.-B. Gao characterized the structure of single crystal. X. Tang and Andrew C.-H. Sue drafted the manuscript with contributions from K. Tan, X.-C. Wang, H. Qu, L.-L. Yang, Z.-Q. Tian and X.-Y. Cao. All authors discussed the results and commented on the manuscript.
Conflicts of interest
There are no conflicts to declare.
Acknowledgements
We thank Pei Zhang, Yu Wang, Zhiwei Lin, Liubin Feng, Hongxun Fang, Changqing Rao, Si Chen and Xujing Lin for helpful discussion and assistance in experiments. This work was supported by the National Natural Science Foundation of China (NSFC) (No. 21971216, 21722304, 91427304, 21573181 and 91227111), the Top-Notch Young Talents Program of China and the Fundamental Research Funds for the Central Universities of China (No. 20720160050).
References
-
(a) T. Hasell, J. A. Armstrong, K. E. Jelfs, F. H. Tay, K. M. Thomas, S. G. Kazarian and A. I. Cooper, Chem. Commun., 2013, 49, 9410–9412 RSC;
(b) L. J. Chen, P. S. Reiss, S. Y. Chong, D. Holden, K. E. Jelfs, T. Hasell, M. A. Little, A. Kewley, M. E. Briggs, A. Stephenson, K. M. Thomas, J. A. Armstrong, J. Bell, J. Busto, R. Noel, J. Liu, D. M. Strachan, P. K. Thallapally and A. I. Cooper, Nat. Mater., 2014, 13, 954–960 CrossRef CAS;
(c) G. Zhang, O. Presly, F. White, I. M. Oppel and M. Mastalerz, Angew. Chem., Int. Ed., 2014, 53, 1516–1520 CrossRef CAS PubMed.
-
(a) C. X. Zhang, Q. Wang, H. Long and W. Zhang, J. Am. Chem. Soc., 2011, 133, 20995–21001 CrossRef CAS PubMed;
(b) C. X. Zhang, H. Long and W. Zhang, Chem. Commun., 2012, 48, 6172–6174 RSC;
(c) L. Zhao, Y. L. Chu, C. He and C. Y. Duan, Chem. Commun., 2014, 50, 3467–3469 RSC;
(d) W. M. Bloch, Y. Abe, J. J. Holstein, C. M. Wandtke, B. Dittrich and G. H. Clever, J. Am. Chem. Soc., 2016, 138, 13750–13755 CrossRef CAS;
(e) M. M. Zhang, M. L. Saha, M. Wang, Z. X. Zhou, B. Song, C. J. Lu, X. Z. Yan, X. P. Li, F. H. Huang, S. C. Yin and P. J. Stang, J. Am. Chem. Soc., 2017, 139, 5067–5074 CrossRef CAS;
(f) W. Y. Zhang, D. Yang, J. Zhao, L. K. Hou, J. L. Sessler, X. J. Yang and B. Wu, J. Am. Chem. Soc., 2018, 140, 5248–5256 CrossRef CAS PubMed;
(g) R. J. Li, J. J. Holstein, W. G. Hiller, J. Andréasson and G. H. Clever, J. Am. Chem. Soc., 2019, 141, 2097–2103 CrossRef CAS;
(h) L. Zhang, Y. Jin, G.-H. Tao, Y. Gong, Y. Hu, L. He and W. Zhang, Angew. Chem., Int. Ed., 2020, 59, 20846–20851 CrossRef CAS PubMed.
- T. Mitra, K. E. Jelfs, M. Schmidtmann, A. Ahmed, S. Y. Chong, D. J. Adams and A. I. Cooper, Nat. Chem., 2013, 5, 276–281 CrossRef CAS.
-
(a) M. Yoshizawa, M. Tamura and M. Fujita, Science, 2006, 312, 251–254 CrossRef CAS;
(b) M. D. Pluth, R. G. Bergman and K. N. Raymond, Science, 2007, 316, 85–88 CrossRef CAS PubMed;
(c) C. J. Hastings, D. Fiedler, R. G. Bergman and K. N. Raymond, J. Am. Chem. Soc., 2008, 130, 10977–10983 CrossRef CAS PubMed;
(d) W. Cullen, M. C. Misuraca, C. A. Hunter, N. H. Williams and M. D. Ward, Nat. Chem., 2016, 8, 231–236 CrossRef CAS PubMed;
(e) L. Qiu, R. McCaffrey, Y. Jin, Y. Gong, Y. Hu, H. Sun, W. Park and W. Zhang, Chem. Sci., 2018, 9, 676–680 RSC;
(f) N. Sun, C. Wang, H. Wang, L. Yang, P. Jin, W. Zhang and J. Jiang, Angew. Chem., Int. Ed., 2019, 58, 18011–18016 CrossRef CAS.
-
(a) P. Mal, B. Breiner, K. Rissanen and J. R. Nitschke, Science, 2009, 324, 1697–1699 CrossRef CAS;
(b) T. Y. Jiao, L. Chen, D. Yang, X. Li, G. C. Wu, P. M. Zeng, A. K. Zhou, Q. Yin, Y. J. Pan, B. Wu, X. Hong, X. Q. Kong, V. M. Lynch, J. L. Sessler and H. Li, Angew. Chem., Int. Ed., 2017, 56, 14545–14550 CrossRef CAS PubMed;
(c) D. Yang, J. Zhao, L. Yu, X. Lin, W. Zhang, H. Ma, A. Gogoll, Z. Zhang, Y. Wang, X. J. Yang and B. Wu, J. Am. Chem. Soc., 2017, 139, 5946–5951 CrossRef CAS.
-
(a) M. Fujita, S. Nagao and K. Ogura, J. Am. Chem. Soc., 1995, 117, 1649–1650 CrossRef CAS PubMed;
(b) M. Mastalerz, Chem. Commun., 2008, 4756–4758 RSC;
(c) T. Hasell, X. F. Wu, J. T. Jones, J. Bacsa, A. Steiner, T. Mitra, A. Trewin, D. J. Adams and A. I. Cooper, Nat. Chem., 2010, 2, 750–755 CrossRef CAS;
(d) Q. F. Sun, J. Iwasa, D. Ogawa, Y. Ishido, S. Sato, T. Ozeki, Y. Sei, K. Yamaguchi and M. Fujita, Science, 2010, 328, 1144–1147 CrossRef CAS;
(e) Y. Z. Liu, C. H. Hu, A. Comotti and M. D. Ward, Science, 2011, 333, 436–440 CrossRef CAS;
(f) H. Li, H. C. Zhang, A. D. Lammer, M. Wang, X. P. Li, V. M. Lynch and J. L. Sessler, Nat. Chem., 2015, 7, 1003–1008 CrossRef CAS;
(g) S. Zarra, D. M. Wood, D. A. Roberts and J. R. Nitschke, Chem. Soc. Rev., 2015, 44, 419–432 RSC;
(h) T. R. Cook and P. J. Stang, Chem. Rev., 2015, 115, 7001–7045 CrossRef CAS PubMed;
(i) D. Fujita, Y. Ueda, S. Sato, N. Mizuno, T. Kumasaka and M. Fujita, Nature, 2016, 540, 563–566 CrossRef CAS PubMed;
(j) S. Chakraborty, W. Hong, K. J. Endres, T. Z. Xie, L. Wojtas, C. N. Moorefield, C. Wesdemiotis and G. R. Newkome, J. Am. Chem. Soc., 2017, 139, 3012–3020 CrossRef CAS;
(k) B. Chen, J. J. Holstein, S. Horiuchi, W. G. Hiller and G. H. Clever, J. Am. Chem. Soc., 2019, 141, 8907–8913 CrossRef CAS PubMed;
(l) S. Huang, Z. Lei, Y. Jin and W. Zhang, Chem. Sci., 2021, 12, 9591–9606 RSC.
-
(a) C. Zhao, Q. F. Sun, W. M. Hart-Cooper, A. G. DiPasquale, F. D. Toste, R. G. Bergman and K. N. Raymond, J. Am. Chem. Soc., 2013, 135, 18802–18805 CrossRef CAS;
(b) J. Guo, Y. W. Xu, K. Li, L. M. Xiao, S. Chen, K. Wu, X. D. Chen, Y. Z. Fan, J. M. Liu and C. Y. Su, Angew. Chem., Int. Ed., 2017, 56, 3852–3856 CrossRef CAS;
(c) J. J. Jiao, C. X. Tan, Z. J. Li, Y. Liu, X. Han and Y. Cui, J. Am. Chem. Soc., 2018, 140, 2251–2259 CrossRef CAS PubMed;
(d) C. X. Tan, J. J. Jiao, Z. J. Li, Y. Liu, X. Han and Y. Cui, Angew. Chem., Int. Ed., 2018, 57, 2085–2090 CrossRef CAS;
(e) C. X. Tan, D. D. Chu, X. H. Tang, Y. Liu, W. M. Xuan and Y. Cui, Chem.–Eur. J., 2019, 25, 662–672 CrossRef CAS PubMed;
(f) Y. Wang, Y. B. Sun, P. C. Shi, M. M. Sartin, X. J. Lin, P. Zhang, H. X. Fang, P. X. Peng, Z. Q. Tian and X. Y. Cao, Chem. Sci., 2019, 10, 8076–8082 RSC;
(g) P. M. Punt, M. D. Langenberg, O. Altan and G. H. Clever, J. Am. Chem. Soc., 2021, 143, 3555–3561 CrossRef CAS PubMed.
-
(a) W. M. Xuan, M. N. Zhang, Y. Liu, Z. J. Chen and Y. Cui, J. Am. Chem. Soc., 2012, 134, 6904–6907 CrossRef CAS PubMed;
(b) K. Wu, K. Li, Y. J. Hou, M. Pan, L. Y. Zhang, L. Chen and C. Y. Su, Nat. Commun., 2016, 7, 10487 CrossRef CAS PubMed;
(c) Y. J. Hou, K. Wu, Z. W. Wei, K. Li, Y. L. Lu, C. Y. Zhu, J. S. Wang, M. Pan, J. J. Jiang, G. Q. Li and C. Y. Su, J. Am. Chem. Soc., 2018, 140, 18183–18191 CrossRef CAS.
-
(a) N. Ousaka, J. K. Clegg and J. R. Nitschke, Angew. Chem., Int. Ed., 2012, 51, 1464–1468 CrossRef CAS;
(b) M. C. Young, L. R. Holloway, A. M. Johnson and R. J. Hooley, Angew. Chem., Int. Ed., 2014, 53, 9832–9836 CrossRef CAS;
(c) L. L. Yan, C. H. Tan, G. L. Zhang, L. P. Zhou, J. C. Bunzli and Q. F. Sun, J. Am. Chem. Soc., 2015, 137, 8550–8555 CrossRef CAS;
(d) A. M. Castilla, M. A. Miller, J. R. Nitschke and M. M. Smulders, Angew. Chem., Int. Ed., 2016, 55, 10616–10620 CrossRef CAS PubMed;
(e) Y. Y. Zhou, H. F. Li, T. Y. Zhu, T. Gao and P. F. Yan, J. Am. Chem. Soc., 2019, 141, 19634–19643 CrossRef CAS;
(f) P. Howlader, E. Zangrando and P. S. Mukherjee, J. Am. Chem. Soc., 2020, 142, 9070–9078 CrossRef CAS PubMed;
(g) Q. X. Shi, X. H. Zhou, W. Yuan, X. S. Su, A. Neniskis, X. Wei, L. Taujenis, G. Snarskis, J. S. Ward, K. Rissanen, J. de Mendoza and E. Orentas, J. Am. Chem. Soc., 2020, 142, 3658–3670 CrossRef CAS.
- W. J. Meng, J. K. Clegg, J. D. Thoburn and J. R. Nitschke, J. Am. Chem. Soc., 2011, 133, 13652–13660 CrossRef CAS PubMed.
-
(a) C. M. Hong, D. M. Kaphan, R. G. Bergman, K. N. Raymond and F. D. Toste, J. Am. Chem. Soc., 2017, 139, 8013–8021 CrossRef CAS PubMed;
(b) D. W. Zhang, T. K. Ronson, J. L. Greenfield, T. Brotin, P. Berthault, E. Leonce, J. L. Zhu, L. Xu and J. R. Nitschke, J. Am. Chem. Soc., 2019, 141, 8339–8345 CAS;
(c) C. Bravin, G. Mason, G. Licini and C. Zonta, J. Am. Chem. Soc., 2019, 141, 11963–11969 CrossRef CAS;
(d) P. Howlader, S. Mondal, S. Ahmed and P. S. Mukherjee, J. Am. Chem. Soc., 2020, 142, 20968–20972 CrossRef CAS;
(e) L. Cheng, K. Liu, Y. J. Duan, H. H. Duan, Y. W. Li, M. Gao and L. P. Cao, CCS Chem., 2020, 2749–2751 CrossRef;
(f) B. Y. Li, B. Zheng, W. Y. Zhang, D. Zhang, X. J. Yang and B. Wu, J. Am. Chem. Soc., 2020, 142, 6304–6311 CrossRef CAS PubMed.
-
(a)
R. B. Fuller, A. L. Loeb and E. J. Applewhtie, Synergetics: Explorations in the Geometry of Thinking, Macmillan Pub. Co., 1975 Search PubMed;
(b) Y. Wang, H. X. Fang, W. Zhang, Y. B. Zhuang, Z. Q. Tian and X. Y. Cao, Chem. Commun., 2017, 53, 8956–8959 RSC;
(c) H. Qu, Z. Y. Huang, X. Dong, X. C. Wang, X. Tang, Z. H. Li, W. B. Gao, H. L. Liu, R. S. Huang, Z. J. Zhao, H. Zhang, L. L. Yang, Z. Q. Tian and X. Y. Cao, J. Am. Chem. Soc., 2020, 142, 16223–16228 CrossRef CAS.
-
(a) X. C. Wang, Y. Wang, H. Y. Yang, H. X. Fang, R. Chen, Y. B. Sun, N. F. Zheng, K. Tan, X. Lu, Z. Q. Tian and X. Y. Cao, Nat. Commun., 2016, 7, 12469 CrossRef;
(b) Y. Wang, H. X. Fang, I. Tranca, H. Qu, X. C. Wang, A. J. Markvoort, Z. Q. Tian and X. Y. Cao, Nat. Commun., 2018, 9, 488 CrossRef PubMed.
- P. Zhang, X. C. Wang, W. Xuan, P. X. Peng, Z. H. Li, R. Q. Lu, S. Wu, Z. Q. Tian and X. Y. Cao, Chem. Commun., 2018, 54, 4685–4688 RSC.
-
(a) M. E. Belowich and J. F. Stoddart, Chem. Soc. Rev., 2012, 41, 2003–2024 RSC;
(b) Y. H. Jin, Q. Wang, P. Taynton and W. Zhang, Acc. Chem. Res., 2014, 47, 1575–1586 CrossRef CAS PubMed.
- E. R. Johnson, S. Keinan, P. Mori-Sanchez, J. Contreras-Garcia, A. J. Cohen and W. T. Yang, J. Am. Chem. Soc., 2010, 132, 6498–6506 CrossRef CAS.
Footnote |
† Electronic supplementary information (ESI) available. CCDC 2053589. For ESI and crystallographic data in CIF or other electronic format see DOI: 10.1039/d1sc03428f |
|
This journal is © The Royal Society of Chemistry 2021 |
Click here to see how this site uses Cookies. View our privacy policy here.