DOI:
10.1039/D1SC03714E
(Edge Article)
Chem. Sci., 2021,
12, 12391-12399
Tuning of bandgaps and emission properties of light-emitting diode materials through homogeneous alloying in molecular crystals†
Received
8th July 2021
, Accepted 14th August 2021
First published on 16th August 2021
Abstract
Alloy formation is ubiquitous in inorganic materials science, and it strongly depends on the similarity between the alloyed atoms. Since molecules have widely different shapes, sizes and bonding properties, it is highly challenging to make alloyed molecular crystals. Here we report the generation of homogenous molecular alloys of organic light emitting diode materials that leads to tuning in their bandgaps and fluorescence emission. Tris(8-hydroxyquinolinato)aluminium (Alq3) and its Ga, In and Cr analogues (Gaq3, Inq3, and Crq3) form homogeneous mixed crystal phases thereby resulting in binary, ternary and even quaternary molecular alloys. The MxM′(1−x)q3 alloy crystals are investigated using X-ray diffraction, energy dispersive X-ray spectroscopy and Raman spectroscopy on single crystal samples, and photoluminescence properties are measured on the exact same single crystal specimens. The different series of alloys exhibit distinct trends in their optical bandgaps compared with their parent crystals. In the AlxGa(1−x)q3 alloys the emission wavelengths lie in between those of the parent crystals, while the AlxIn(1−x)q3 and GaxIn(1−x)q3 alloys have red shifts. Intriguingly, efficient fluorescence quenching is observed for the MxCr(1−x)q3 alloys (M = Al, Ga) revealing the effect of paramagnetic molecular doping, and corroborating the molecular scale phase homogeneity.
Introduction
Alloy formation represents one of the cornerstones in inorganic materials science. Ever since the bronze age, humankind has relied on numerous materials that owe their unique properties to the continuous mixing of similar atoms or ions in the crystalline state thereby forming solids with random disorder. In sharp contrast the formation of alloys between molecular species is highly challenging since molecules are unique in size, shape and properties. Crystal engineering approaches directed towards multi-component crystals are largely based on cocrystal design utilizing supramolecular synthons that non-covalently bind different component molecules in crystals.1 While cocrystals are limited to the fixed stoichiometries of molecular components, organic alloys are characterized by their continuous stoichiometries in crystals. Since the possibilities of different molecular components occupying similar crystal lattices are limited, the reports of molecular alloy crystals are relatively rare.2–10 Moreover, studies that explore tuning of physical properties of molecular materials by alloy formation are even rarer – despite the promising prospects of alloys in bandgap engineering of organic semiconductors and molecular photovoltaic crystals.11,12 Although mixed solid state phases formed by melt-cooling of different molecular components, or by doping in polymer matrices, are sometimes referred to as alloy phases, these composite phases need to be clearly distinguished from molecular alloy crystals, which are strictly characterized by homogenous mixing of molecular components in their crystal lattices. Studies of metal organic frameworks and organic–inorganic hybrid perovskite structures that show composition variation with respect to ionic species in the crystal lattices have been reported with tuning in the optical/electrical properties, but they are not strictly classified as organic alloys.13,14 A well-known class of organic alloy crystals are those formed by donor–acceptor charge transfer complexes. Matzger et al. and Pei et al. explored the variation in bandgaps of molecular solid solutions, but continuous or systematic trends in bandgaps could not be observed in these alloys.15,16 Recently, we demonstrated systematic tuning in the optical band gaps in molecular alloys formed by a series of diphenyl dichalcogenides.17 In the context of photophysical properties of molecular alloys, Fu et al. demonstrated the tuning of photoluminescence emission and optical waveguiding performances of charge transfer alloy crystals formed by perylene and 9,10-dicyano-anthracene (DCA)1−x(Pe)x.18 Although the composition range of the alloy components in these examples were limited to <10%, this report points to the prospects of achieving desirable tuning of photophysical properties if high composition ranges could be achieved in molecular alloys. In this article, we report the crystalline molecular alloys formed by organic light-emitting diode (OLED) materials Mq3, where M = Al, Ga, In, and Cr and q = 8-hydroxyquinoline ligand. Tris(8-hydroxyquinoline)aluminum or Alq3 is widely used as the light emitting layer material in OLED devices.19,20 Light emission is generated in such emissive layers by electron–hole recombination. Hence, it is logical to assume that the tuning of bandgaps (such as the trend reported by us in dichalcogenide molecular alloys17) may also result in the tuning of emission properties of OLED components such as Alq3. Tuning of emission properties in Mq3 compounds are primarily attempted by means of chemical derivatives of the ligand molecule by adding functional groups to 8-hydroxyquinoline ligand.21,22 However, such methods result in significant changes from the original crystal structure of Mq3 in packing features, intermolecular interactions and thereby in opto-electronic properties. Recently, Han et al. reported alloy crystal forms of Mq3 methanol solvates and Mq3 nano/microrods obtained through a solution pathway.23 However, the structural characterization of the mixed crystals were limited to the solvate crystal Al0.5Ga0.5q3 MeOH, the crystal structure of which was quite different from that of the pure Alq3 or Gaq3. To our knowledge there is no systematic structure–property relation study on the multicomponent molecular alloy forms of Mq3 series of crystalline OLED materials. Here we report the binary, tertiary and quaternary molecular alloys of Alq3, Gaq3, Inq3, and Crq3 that form homogenously mixed crystal phases. A detailed structural study correlating the crystal structures, composition and domain structures with the trends in optical band gaps and fluorescence emission properties observed for the alloy crystals is described here employing single crystal X-ray diffraction (SCXRD), powder XRD, energy dispersive X-ray (EDX), Raman and fluorescence spectroscopy. Since Mq3 systems exhibit multiple crystal polymorphs and two different stereoisomers (see Fig. 1a), the effect of such structural preferences in the alloy formation and emission properties are investigated. In addition, we report an hitherto unknown phenomenon of fluorescence quenching in molecular alloys (in the alloy crystals formed by Crq3) revealing the effect of paramagnetic molecular doping/impurity on the emission properties of these OLED materials.
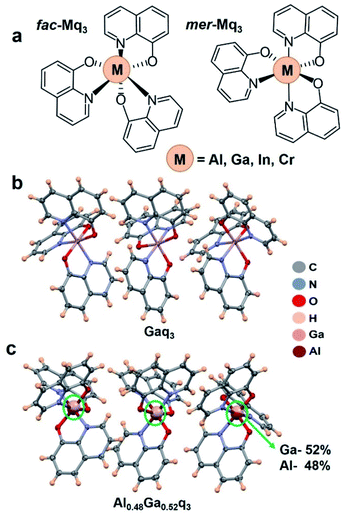 |
| Fig. 1 (a) The two possible stereoisomers, namely meridional (mer) and facial (fac), of Mq3 molecules discussed in this study, where M = Al, Ga, In, and Cr and q = 8-hydroxyquinoline ligand. Asymmetric units of (b) the new polymorph of Gaq3 and (c) the binary alloy Al0.48Ga0.52q3 shown as thermal ellipsoid plots at the 50% probability level (metal atoms of the alloy structure have been highlighted as enlarged balls). | |
Results and discussion
Polymorphs and stereoisomers of Mq3 compounds and the generation of their alloy crystals
In this study, we employed a two-zone sublimation–condensation method to obtain crystals of the Mq3 compounds. The Mq3 series of compounds exhibit two stereoisomers; a meridional (mer) isomer with C1 symmetry and facial (fac) isomer with C3 symmetry, as shown in Fig. 1a. They are known to crystallize in five different crystal forms – resulting from a combination of polymorphs and stereoisomers – referred to as α, β, γ, δ, and ε. While α, β, and ε forms are adopted by the mer isomer, the γ and δ forms are associated with the fac stereoisomer.24,25 The central metal atom M is in +3 oxidation state in all the Mq3 compounds discussed here. The preferences for the stereoisomers are known to be different in the series of Mq3 compounds, depending upon the size and covalent radii of the central metal atom. The preference for fac or mer isomer is a result of the tradeoff between two opposite factors26 (i) the structure destabilizing steric effect between the ligands which is higher in fac form and increases with decreasing covalent radii, (ii) the stabilizing effect from stronger metal–ligand orbital interactions and bonding, which is predominant in the fac isomer. It is also known that the In–N and In–O bonds are more covalent as compared to other M–O and M–N bonds in this series.20 Hence, for Alq3, Gaq3, and Crq3 the mer isomer is found to be more stable, whereas for Inq3 the fac isomer is preferred (owing to the higher covalent radius of In). The crystal forms obtained in this study are in accordance with this trend in stability of isomers. For Alq3, Gaq3 and Crq3 the crystal forms obtained by two-zone sublimation–condensation method were determined to be mer-ε form, whereas the Inq3 crystals obtained were found to be predominantly fac-δ form. The crystals showed acicular morphology (Fig. S1†), and their crystal structures were confirmed by single crystal XRD experiments at 100 K, except for Inq3 for which the poor quality of the single crystals allowed only the determination of unit cell parameters. Table S2 in the ESI† shows the crystallographic cell parameters for the different crystal forms discussed here. The crystal structures of mer-ε form adopted by Alq3 and Crq3 have Z = 6, with 3 molecules in the asymmetric unit (Z′ = 3) and crystallographic space group P
. The cell parameters of Alq3 and Crq3 showed similarity implying their isostructurality. Although only the β form of Gaq3 is known (CSD code: ETELAQ), in this study we discovered a new polymorph of Gaq3Z = 6 and Z′ = 3 in triclinic space group P
, which is isostructural to the ε form of Alq3 and Crq3. Fig. S2 in the ESI† shows the crystal packing diagrams of mer-Gaq3 in β and ε forms. The discovery of this mer-ε polymorph points to the propensity of Gaq3 to potentially occupy the lattice of Alq3 or Crq3 crystals.
A commonly reported class of solid solution/alloy crystals are formed between planar aromatic molecules as donor–acceptor charge transfer complexes, where two or more electron-acceptor molecules are sandwiched between planar aromatic electron donor molecules.15,18,27 Hence the individual crystal structures of the electron-acceptor molecules are not a crucial factor in the alloy formation. On the contrary, for nonplanar molecules to form alloys, isostructurality of the crystal structures of individual components is an important structural criterion.
The crystal structures of the Mq3 compounds (Alq3, Gaq3, Crq3 and Inq3) are stabilized by intermolecular C–H⋯π, C–H⋯O interactions and π⋯π stacking interactions (with a partial stacking of the aromatic rings). To obtain a more quantitative understanding of the crystal packing and interactions in these structures, we employed Hirshfeld fingerprint analysis28 which revealed percentage contribution of different types of intermolecular contacts. Hirshfeld surface analysis revealed that the isostructurality in crystal packing and unit cell parameters also manifest as the percentage contributions of C⋯C and H⋯C, and H⋯O interatomic contacts (corresponding to the proportions of C–H… π, C–H⋯O interactions and π⋯π stacking interactions) – which are very similar among the series of Mq3 crystal structures (Fig. S3 and Table S1†). These results are akin to the previously reported examples of isostructural interaction topologies in analogous crystal structures4,17 reported by us (represented as energy frameworks29), which facilitated alloy formation. Their unit cell volumes are also very similar, and show the following order: Vcell(Alq3) < Vcell(Gaq3) < Vcell(Crq3) (see ESI Table S2†).
Inspired by the isostructurality of Alq3, Gaq3 and Crq3 (as can be seen from Tables S1 and S2†), we further probed the possibility of alloy formation between these compounds. It should be noted that Han et al. recently reported mixed alloy-like crystals of Mq3 solvates and crystalline nanorods.23 The powder diffractograms of the nano/microrods obtained by the mixed phases of Alq3 and Gaq3 from chloroform solvent indicated a possible α polymorph formation, although these phases were not structurally characterized. Moreover, the composition of the alloy phases were not determined but arbitrarily assigned to the ratio of parent compounds used for crystallization. Here, firstly we set out to synthesize binary molecular alloys using the following combinations: Alq3–Gaq3, Alq3–Crq3 and Gaq3–Crq3 in different stoichiometric ratios. In order to obtain the single crystals of the binary alloys devoid of any solvent molecules (that might potentially co-crystallize and alter the crystal structure), we employed two-zone sublimation–condensation method. Crystals of Alq3–Gaq3, Alq3–Crq3 and Gaq3–Crq3 alloy phases thus obtained were of acicular morphology, very similar to their parent crystals. SCXRD analysis of the single crystals of the alloys revealed a wide range of compositions (see Tables S3–S7†). We have recently demonstrated that single crystals of molecular alloys obtained from the same crystallization experiment can show a range of composition of their components.17 Here, we find that accurate stoichiometry control in the alloy crystals is not possible for the systems in this study (using the sublimation–condensation method). Nevertheless, the compositions of the alloy single crystals from SCXRD roughly match with the stoichiometry of the compound mixtures used in their crystallization although with some exceptions (see Tables S3–S7†). The deviation of the composition from their starting stoichiometries were particularly dominant for the alloy crystals containing Crq3, where the percentage of Crq3 was generally found to be higher than the stoichiometry used in the crystallization mixture.
As opposed to Alq3, Gaq3 and Crq3, the readily obtained form of Inq3 is the fac isomer crystallizing in δ form (Z = 2). Although the mer-Inq3 is reported, it crystallizes in β form (CSD code: WADGUZ).30 Hence, the alloys of Inq3 with other Mq3 compounds were found to be quite difficult to obtain possibly due to the fact that Inq3 prefers a fac stereoisomer as opposed to the other three Mq3 compounds. We could not obtain alloy single crystals of Inq3 with Alq3, Gaq3 or Crq3 for higher composition of Inq3 in the alloy form. Since the major focus of this study is to obtain structure–property relations in the molecular alloys of this series of OLED materials at a molecular to crystal domain level, our investigations were focused mainly on single crystals. Because good quality single crystals could not be obtained for 30% and 50% alloys of Inq3 with Alq3 and Gaq3, we used powder-XRD to characterize these phases (see ESI†). Molecular alloy formation inherently leads to crystallographic disorders with respect to both positions and site occupancy values, and we tested two strategies to model the alloy crystal structures. The structural models with constraints on the positions and atomic displacement parameters (ADPs) of the central metal atoms of Mq3 were especially found to result in satisfactory convergence in the crystallographic refinements. The occupancy values of metal atoms in the binary alloy crystals were refined using an occupancy free variable (see ESI†).
It is interesting to note some structural features of Mq3 compounds that exhibit changes in the alloy crystal structures as compared to their parent crystal forms. The metal–ligand bond distances (M–O and M–N bonds) in the series of Mq3 structures and in the alloys are of particular interest. The average M–O distances for the series of Mq3 crystal structures from the SCXRD analysis are in the following order: Alq3 (1.86 Å) < Gaq3 (1.95 Å) < Crq3 (1.96 Å). Whereas the M–N bond distances shows the order of Alq3 (2.03 Å) < Crq3 (2.04 Å) < Gaq3 (2.08 Å). Interestingly, the M–O and M–N bond distances in the alloy crystal structures are found to be intermediate between the corresponding bond distances in their parent crystal structures. Fig. 2 shows the trends in M–O and M–N bond distances in Alq3–Gaq3 and Alq3–Crq3 alloy crystals, with the bond distance variations roughly corelating with the composition of the alloys (also, see Table S9†). This ‘bond averaging effect’ in the alloy crystals is likely due to the spatial averaging of the corresponding metal and ligand atom positions in the alloy crystals, rather than a realistic bond elongation/compression of the M–O and M–N bonds. However, this indicates the ability of different component Mq3 molecules to occupy very similar crystallographic positions in the alloy crystal lattice in a more or less homogenous manner.
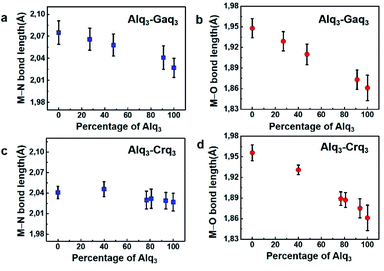 |
| Fig. 2 Variations in M–O and M–N bond distances observed in the crystal structures of (a, b) Alq3–Gaq3 and (c, d) Alq3–Crq3 alloys with respect to the corresponding bond distances in their parent crystal structures. | |
Ternary and quaternary alloy crystals of Mq3
Further, we set out to examine if ternary and quaternary molecular alloys could be obtained from this series of Mq3 compounds. By extending the same crystallization approach with 1
:
1
:
1 stoichiometric ratio of Alq3, Gaq3 and Crq3 as well as 1
:
1
:
1
:
1 mixture of Alq3, Crq3, Gaq3 and Inq3, we obtained single crystals which were further analyzed using SCXRD and EDX spectroscopy. For binary molecular alloys, the composition obtained from EDX spectra were found to be comparable to the values obtained from SCXRD. However, for ternary and quaternary alloy crystals, the crystallographic occupancy refinement of the closely positioned metal atoms into three or four linearly correlated free variable failed to attain convergence (leading to unreasonable values of occupancies, including negative occupancies). Hence, to obtain realistic crystal structure models for the three component and four component alloys, we measured EDX spectra on the same single crystals used for SCXRD data collection (Fig. 3a and b). The crystal structures of the ternary and quaternary alloys are thus refined using SCXRD data with values of metal atom occupancies fixed to the composition values obtained from the EDX spectra. To our knowledge, these structures represent the rare examples of quaternary molecular alloys (medium entropy alloys) formed by nonplanar molecules. The examples of six-component solid-solutions with three stoichiometry-tunable molecular components reported by Desiraju et al.,7,8 and the quaternary solid solutions of donor–acceptor type charge transfer complexes reported by Matzger et al. are to be noted in this context.15
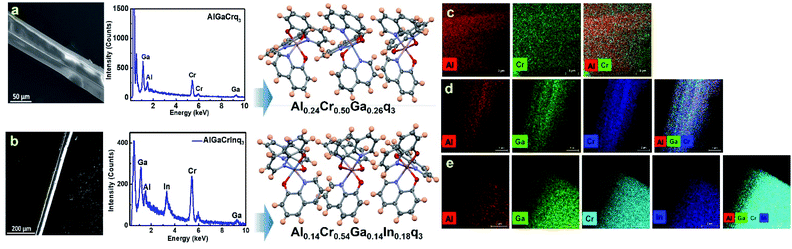 |
| Fig. 3 Scanning electron microscopic images of (a) ternary and (b) quaternary alloy single crystals along with their EDX spectra, and the corresponding single crystal X-ray structural models (thermal ellipsoid plot at 50% probability level) obtained using the composition values from the EDX spectra. EDX maps of alloy crystals showing the homogenous distribution of their components in (c) Alq3–Crq3 binary, (d) Alq3–Gaq3–Crq3 ternary and (e) Alq3–Gaq3–Inq3–Crq3 quaternary alloys. | |
Spectroscopic studies of the domain structure and homogeneity of the alloy crystals
Although SCXRD provides the structure of the alloy crystals with multiple components in the lattice, it gives only a spatially averaged picture of the molecules in the alloy crystals. Beyond molecular structure and crystallographic parameters, domain structure is an important feature in alloy crystal phases that influences their physical properties. The long-range distribution of molecular components in a homogenous or heterogenous manner can dictate the degree of mixing of electronic states corresponding to individual components in the alloy crystals. In a recent report, we investigated the molecular alloy crystals formed by diphenyl disulfide and diphenyl diselenide analogues using EDX spectroscopy revealing a homogenous distribution in the alloy phases.17 In this study, we employed EDX spectroscopy to (i) probe the domain structure of the alloy phases, and (ii) obtain their accurate compositions. We performed high-resolution mapping of the elemental composition on the single crystals of the alloys (Fig. 3c–e) as well as on the microparticles obtained by dispersing the single crystals of the alloys in ethanol (Fig. S19†). Fig. 3c–e show that the alloy crystals show homogenous distribution of molecular components within the resolution of the EDX mapping (2 nm). Since the domain structure from the EDX spectra implies the molecular level mixing of different components in the alloy crystal lattices, such a homogenous distribution can lead to new type of intermolecular interactions – different from those present in the parent crystals. For example, the nature of intermolecular interactions present in the Alq3–Crq3 alloy crystal could be different from those in Alq3 and Crq3 parent crystals. To probe any intermolecular effects such as charge transfer between the different components in the alloy crystals, we performed Raman spectroscopic studies. Fig. 4a–d shows the Raman spectra measured on the single crystals of pure Alq3, Gaq3, Crq3, Inq3 and their alloy crystals. In this study, we focused on the spectral range of 80–800 cm−1 which contain the characteristic peaks corresponding to the metal–ligand inter-actions. For pure Alq3 crystals, we observe a peak at 118 cm−1 corresponding to the Al–oxine deformation and Al–N stretching, and a significant peak is observed at 525 cm−1 corresponding to ring deformation and Al–O stretching. The peak observed at 314 cm−1 is attributed to Al–N stretching and C–C–O bending.31,32 All these peaks are characteristic features of the mer isomer of Alq3 and it confirms that the crystals obtained are in mer form. All the observed peak positions and their vibrational assignments are tabulated in Tables S10 and S11 in the ESI.† Gaq3 and Crq3 crystals also showed similar peaks with slight differences in the peak positions with the change in the central metal atom. However, the spectra obtained for pure Inq3 crystals are different from those of the Alq3, Gaq3 and Crq3. A comparison of the Raman spectra obtained for Inq3 with those reported in the literature for fac-Alq3 indicate that the crystals could be predominantly in fac form31 as it was also indicated by SCXRD measurements (since the weakly diffracting single crystals of Inq3 were too small to obtain complete diffraction data for the structural refinement, only the unit cell parameters were obtained from the SCXRD measurements). Raman spectra of the binary alloy crystals formed by Alq3, Gaq3 and Crq3 were found to be very similar to their parent crystals with no significant shifts in their characteristic peak positions.31,32 This implies the absence of any considerable charge transfer type interactions between the molecular components in the alloys. An observable effect in the Raman spectra of the alloy crystals is a relative broadening of these characteristic peaks compared to parent crystals. The fact that the characteristic peaks corresponding to the M–O and M–N stretching modes do not show any significant change in the alloy crystals (compared to the parent crystals) further substantiates our argument that the bond distance averaging in the alloy structures is the consequence of the crystallographic spatial averaging rather that a bond relaxation effect. On the other hand, alloys formed by Inq3 show a different trend from that of the pure Inq3 crystals. As discussed earlier, the pure Alq3 Raman spectrum corresponds to the mer isomeric form while that of Inq3 exhibit spectral features corresponding to fac isomer as it is the preferred isomer for Inq3. However, the Raman spectra of the alloy phases of Inq3 reveal an intriguing trend: when the composition of Alq3 is higher in Alq3–Inq3 alloy crystal, the spectra match well with that of the mer isomeric form. On the other hand, as the concentration of Alq3 decreases, the characteristic peaks of the mer isomer disappears and peaks corresponding to fac isomer emerge in the spectra (Fig. 4c). In crystals of Alq3, the peak at 525 cm−1 corresponds to mer isomer and the peaks at 535 cm−1 and 548 cm−1 correspond to fac isomer. These peaks at 535 cm−1 and 548 cm−1 in Alq3–Inq3 alloy crystals with higher composition of Inq3, such as Alq3–Inq3 (50
:
50), and Alq3–Inq3 (30
:
70) are replaced by a new peak at 525 cm−1 for alloy crystals with low composition of Inq3 (as in 90
:
10 alloy of Alq3–Inq3). This implies that the relatively less stable mer-isomer of Inq3 could be getting entrapped in the Alq3–Inq3 alloy crystal lattice, where it is a minor component.
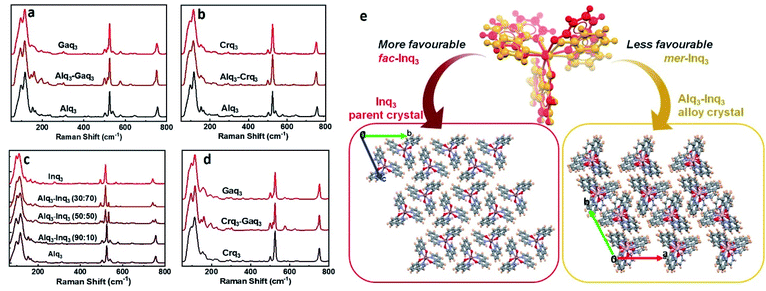 |
| Fig. 4 (a–d) Raman spectra of Alq3, Gaq3, Crq3, Inq3 and their binary alloys measured on single crystals. (e) Structural overlay of fac-Inq3 and mer-Inq3 along with the crystal packing diagrams for fac-Inq3 and the supramolecular ‘entrapment’ of mer-Inq3 in the lattice of mer-Alq3 leading to Alq3-Inq3 alloy crystals. | |
Optical bandgaps of the alloys from UV-Vis diffuse reflectance spectra
A major consequence of the mixing of different molecules that are chemical analogues in the alloy phases could be the possible mixing of their electronic states. This could manifest as changes in the energy band gaps of the alloy crystals. In a recent report, we demonstrated that alloy formation can indeed lead to tuning in optical band gaps of crystals (even in the absence of π–π stacking) in the crystal structure.17 The bandgap is a fundamental feature of a material that is linked to many of its optical and electronic properties. Especially since the Mq3 series of compounds are OLED emissive layer materials, the trends in variation of band gaps in the alloy crystals is of particular interest. Hence, we measured UV-visible diffuse reflectance spectra on the solid-state samples of pure Mq3 crystals and their binary alloys. The diffuse reflectance spectra were transformed using the Kubelka–Munk function33 and the optical bandgaps were estimated from Tauc's plots.34 The bandgaps observed for the series of pure crystals are in the following order Crq3 < Gaq3 < Alq3 < Inq3. Fig. S12† shows that Tauc's plot of Crq3 shows a distinct feature of two separate slopes. It should be noted that the Tauc plot is usually effective when there is nearly zero absorption below the band gap energy, however in case of Crq3 and its alloy crystals, there is a fair amount of absorption below the band gap energy. These absorption features usually arise from impurity or modified structure, or surface modifications. Hence a modified Tauc plot is used in these cases.35 See ESI Fig. S10–S14† for Tauc's plots of all the Mq3 crystals and their alloys. The values of the optical bandgaps for the parent crystals and their alloys are given in Table 1. Notably for Alq3–Gaq3 alloys the bandgap was found to be intermediate to those of pure Alq3 and Gaq3 similar to the trend we reported recently for diphenyl dichalcogenide alloys.17 In case of Alq3–Inq3 alloys the tuning of bandgap to intermediate values (of Alq3 and Inq3) is observed for lower composition of Alq3. Higher concentration of Alq3 (lower concentration of Inq3) in the alloy phase leads to a significant lowering of the band gap compared to the values of both pure Inq3 and Alq3. A similar trend is also observed for Gaq3–Inq3 alloy phases for which bandgap tuning (at high compositions of Inq3) and bandgap lowering (at low compositions of Inq3) were observed.
Table 1 Optical bandgaps (eV) for the crystalline Mq3 samples and their binary alloys obtained from UV-vis diffuse reflectance spectra
Alloy |
Composition ratios |
100 : 0 |
90 : 10 |
50 : 50 |
30 : 70 |
0 : 100 |
Alq3–Gaq3 |
2.65 |
2.63 |
2.62 |
2.60 |
2.59 |
Alq3–Inq3 |
2.65 |
2.61 |
2.67 |
2.66 |
2.71 |
Gaq3–Inq3 |
2.59 |
2.55 |
2.6 |
|
2.71 |
Alq3–Crq3 |
2.65 |
2.54 |
2.53 |
|
2.53 |
Gaq3–Crq3 |
2.59 |
2.53 |
2.53 |
|
2.53 |
A conjoined analysis of the features from Raman spectra alongside SCXRD structures suggest that the possible origin of this distinct trend in bandgaps is in the preferential stabilization of different stereoisomers of Inq3 with different compositions in its alloys. We find that the supramolecular entrapment of mer-Inq3 (an otherwise unfavored isomer of Inq3) as a minor component in the lattice of a mer-Alq3 or mer-Gaq3 major component could be the structural origin of this trend. This leads to intriguing effects in the emission properties of such alloy crystals as evident from the photoluminescence emission maxima described in the following section. Interestingly the bandgaps of the Alq3–Crq3 and Gaq3–Crq3 alloy crystals were found to be very close to the value of pure Crq3 crystals (2.53 eV). Such a lowering of bandgap close to that of the paramagnetic component in molecular alloy crystals is hitherto unknown.
Effect of alloy formation on the photoluminescence emission
To probe the effect of alloy formation in the emission properties of the alloy crystals, we examined the photoluminescence (PL) on the single crystals of the pure Mq3 and their binary alloys. The crystals mounted on a quartz plate were excited at a wavelength of 370 nm. The PL properties of pure Mq3 systems are well studied and their emission is understood to originate from π–π* electronic transition in the ligand (from the HOMO localized around the phenoxide ring to the LUMO localized around the pyridyl ring).36 Their emission maxima are known to vary with the central metal atom, polymorphic forms, and stereoisomers of the Mq3. It was previously reported that with the increase in the size of the metal atom, there could be an increase in the covalent nature of the metal ligand bond leading to the red shift in the emission peak value.20,37 Moreover, the mer isomeric forms showed a red shifted emission compared to the fac isomer in all the Mq3 systems.38 In addition to the stereochemical differences in the mer and fac isomers, variations in their crystal packing and intermolecular interactions might also be resulting in different relative energies for the ground and excited states – leading to shifts in their corresponding emission wavelengths. We observed the following trend in the wavelengths of emission maxima (λmax) for the series of crystals studied here (Fig. 5): Gaq3 (526 nm) > Alq3 (504 nm) > Inq3 (495 nm). Notably in the case of Crq3 no photoluminescence emission was observed. This is evident from the optical microscopic images of Crq3 single crystals (Fig. 5a) as well from the fluorescence spectra (Fig. 5d). Although the PL emission maximum of Inq3 (at 498 nm) seems to defy the trend with respect to metal atom size, it is to be noted that the crystal form of Inq3 obtained in this study are found to be fac form (the more stable stereoisomer for Inq3). The emission maximum we obtained for Inq3 matches with the previously reported PL spectra of fac-Inq3 in powder form.38 Further, we examined the emission features in the PL spectra of the binary alloy crystals. In the case of Alq3–Gaq3 alloy crystal, we observed a tuning of the emission wavelength between the parent compounds i.e., the emission maxima of the alloy crystals fall between the emission maxima of pure Alq3 and Gaq3 (Fig. 5b). With increasing concentration of Gaq3 in the alloy, the emission showed a red shift. However, in the case of Alq3–Inq3 alloy crystals, the trend was quite different. In Alq3–Inq3 alloy crystals with higher composition of Alq3, the emission peak exhibits a red shift compared to both the parent compounds, however, for lower compositions of Alq3, the peak positions are close to the parent compounds (Fig. 5c and g, Table 2). The significant red shifts in the PL emission maxima of Alq3–Inq3 alloys red-shifted from those of the parent crystal forms may be understood in terms of the preferred stereoisomer and the lattice structure of Inq3 molecules in their alloy phases. Possible explanations for the observed red shifts can be (i) charge transfer interaction between Inq3–Alq3 molecules in the crystal lattice leading to changes in the electronic energy levels, or (ii) supramolecular entrapment of the relatively less stable mer isomer of Inq3 in the crystal lattice of the alloys. The latter is consistent with our structural and spectroscopic observations on the alloys. The crystal packing features of the major component lattice of the mer-ε Alq3 prefers the incorporation of mer-Inq3 compared to fac-Inq3. Hence the possible origin of the red shifts observed in the PL emission should be the occurrence of the otherwise less stable mer-Inq3 ‘trapped’ in the alloys, which is supported by SCXRD and Raman spectral analysis as well as by the trend observed in the optical bandgaps.
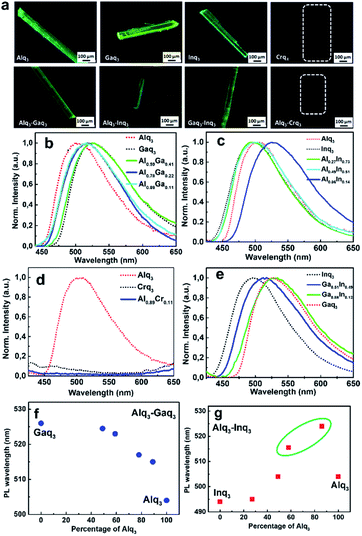 |
| Fig. 5 (a) Optical microscopic images of the single crystals of the parent compounds (top row) and some binary alloys (bottom row), under UV light (λ = 395 nm). (b–e) Photo-luminescence spectra of Alq3, Gaq3, Inq3 and Crq3 in comparison with those of the binary alloys (background corrected). The absence of PL in Crq3 and quenching of PL in Alq3–Crq3 alloy are evident from (d). The samples are excited at λ = 370 nm. (f) The tuning in λmax of the PL emission in Alq3–Gaq3 alloy crystals, and (g) the red shifts in the λmax in Alq3–Inq3 alloy crystals away from the λmax values of the parent crystals. | |
Table 2 Photoluminescence emission maxima (λmax in nm) of the binary alloy crystals
Alq3–Gaq3 |
Alq3–Inq3 |
Gaq3–Inq3 |
Composition |
Em. λmax |
Composition |
Em. λmax |
Composition |
Em. λmax |
Gaq3 pure |
526 |
Inq3 pure |
495 |
Gaq3 pure |
526 |
Al0.59Ga0.41 |
523 |
Al0.27In0.73 |
496 |
Ga0.88In0.12 |
527 |
Al0.78Ga0.22 |
517 |
Al0.49In0.51 |
502 |
Ga0.51In0.49 |
515 |
Al0.89Ga0.11 |
515 |
Al0.86In0.14 |
524 |
|
|
Alq3 pure |
504 |
Alq3 pure |
504 |
Inq3 pure |
495 |
Quenching of photoluminescence emission in the alloy crystals by the paramagnetic Crq3 molecules
As mentioned above, the crystals of Crq3 exhibit no significant PL emission (Fig. 5). However, we observed an intriguing phenomenon of fluorescence quenching caused by Crq3 molecules in its alloy crystals. The binary alloys of Crq3 with Alq3 and Gaq3 shows an effective quenching of PL emission. A similar phenomenon of quenching is also found for the ternary alloy crystals of AlxGayCr1−x−yq3 and the quaternary alloy crystals of AlxGayInzCr1−x−y−zq3. Crq3 is paramagnetic, with the central Cr3+ atom having an electronic configuration of 3d3 (t32ge0g). It is known that paramagnetic metal centers can interact with the excited states of fluorescing molecules leading to a quenching in fluorescence.39,40 Fluorescence quenching by paramagnetic metal ions, such as Cr3+, Fe3+, Co2+, Ni2+, and Cu2+ have been reported,41–43 and this phenomenon has been utilized in the detection of nitroxyl (HNO) in biological systems.40 On the other hand, fluorescence enhancement induced by Cr3+ ions upon complexation with the ligand molecules is also reported.43 It should be noted that most of this reported examples of fluorescence quenching or enhancement are associated with the complexation of paramagnetic metal ions with fluorophore molecules in solution state. The mechanism of quenching by paramagnetic metal centers are generally understood to be based on the photoinduced electron transfer (PET) from a singlet excited state of the fluorophore to paramagnetic metal center upon metal–ligand complexation. However, in the present study the fluorescence quenching is found to be originating from sheer intermolecular interactions between the Crq3 molecules and the fluorescing molecule such as Alq3 or Gaq3 in the alloy lattice. Moreover, we find that the presence of Crq3 leads to highly efficient fluorescence quenching in its molecular alloys with Alq3 and Gaq3 – not only in 1
:
1 alloys, but also in alloys with compositions as low as ∼5% of Crq3. These observations indicate a highly homogenous mixing of the molecular components in the alloy crystals, allowing effective interaction of the paramagnetic Crq3 molecules with the electronic states of the Alq3 or Gaq3 molecules in the neighborhood of the alloy lattices. These results call for in-depth experimental and theoretical investigations, as the exact origin of the quenching phenomenon caused by the intermolecular interactions in the Crq3 alloy crystals are not clear.
Conclusion
In summary, the molecular alloys formed by the Mq3 series of compounds reported in this study exhibit intriguing trends with respect to their spectral and emission properties. The detailed analysis combining SCXRD, EDX and Raman spectra reveal correlations with the observed trends in their structure, optical band gaps and photoluminescence emission properties. In addition, we have reported the rare examples of ternary and quaternary molecular alloys formed by nonplanar molecules. The highly efficient fluorescence quenching caused by Crq3 in its molecular alloy crystals reveals the effect of paramagnetic molecular components in the photoluminescence of alloy phases and provides further evidence for the highly homogenous nature of these alloys. The trends in PL emission peaks observed for different series of binary alloys have been corelated with the trends in their optical band gaps as well as Raman spectroscopic features and the stereoisomeric preference for mer or fac forms. We have shown that the formation of molecular alloys of Inq3 with Alq3 leads to a supramolecular entrapment of the less favored mer stereoisomer of Inq3 in the alloy crystal lattice, leading to red shifts in the emission properties. Alloy crystal engineering using molecular components of chemical analogues as demonstrated in this study could be an efficient design strategy to fine tune the photophysical properties of functional molecular materials.
Data availability
The crystallographic information files have been deposited in the CCDC database with CCDC deposition numbers 2073957–2073962, 2074033–2074036, 2074042–2074044, 2074050–2074051, 2074080–2074081.
Author contribution
RT and SPT performed conceptualisation, data curation, formal analysis, investigation, methodology, and writing of the original draft. HL, AHM, MB and VB performed data curation, formal analysis and methodology. BBI performed conceptualisation, funding acquisition, project administration, supervision, discussion, review and editing.
Conflicts of interest
There are no conflicts to declare.
Acknowledgements
This work was supported by the Villum Foundation grant to BBI. VB acknowledges Novo Nordisk foundation (grant number NNF20OC0061417). SPT acknowledges EU funding for Marie Skłodowska-Curie Individual fellowship (grant number 798633). We thank Prof. Brian Julsgaard for useful discussions.
References
- G. R. Desiraju, J. Am. Chem. Soc., 2013, 135, 9952–9967 CrossRef CAS PubMed.
- D. S. Reddy, D. C. Craig and G. R. Desiraju, Chem. Commun., 1994, 1457–1458, 10.1039/C39940001457.
- M. K. Mishra, U. Ramamurty and G. R. Desiraju, J. Am. Chem. Soc., 2015, 137, 1794–1797 CrossRef CAS PubMed.
- S. P. Thomas, R. Sathishkumar and T. N. Guru Row, Chem. Commun., 2015, 51, 14255–14258 RSC.
- M. Lusi, CrystEngComm, 2018, 20, 7042–7052 RSC.
- S. Chakraborty, S. Joseph and G. R. Desiraju, Angew. Chem., Int. Ed., 2018, 57, 9279–9283 CrossRef CAS PubMed.
- M. Paul, S. Chakraborty and G. R. Desiraju, J. Am. Chem. Soc., 2018, 140, 2309–2315 CrossRef CAS PubMed.
- S. Chakraborty and G. R. Desiraju, Cryst. Growth Des., 2018, 18, 3607–3615 CrossRef CAS.
- M. Dabros, P. R. Emery and V. R. Thalladi, Angew. Chem., Int. Ed., 2007, 46, 4132–4135 CrossRef CAS PubMed.
- M. Lusi, Cryst. Growth Des., 2018, 18, 3704–3712 CrossRef CAS.
- X. Xu, B. Shan, S. Kalytchuk, M. Xie, S. Yang, D. Liu, S. V. Kershaw and Q. Miao, Chem. Commun., 2014, 50, 12828–12831 RSC.
- M. Schwarze, W. Tress, B. Beyer, F. Gao, R. Scholz, C. Poelking, K. Ortstein, A. A. Günther, D. Kasemann, D. Andrienko and K. Leo, Science, 2016, 352, 1446 CrossRef CAS PubMed.
- C. J. Adams, M. F. Haddow, M. Lusi and A. G. Orpen, Proc. Natl. Acad. Sci. U.S.A., 2010, 107, 16033 CrossRef CAS PubMed.
- W.-Q. Liao, D. Zhao, Y.-Y. Tang, Y. Zhang, P.-F. Li, P.-P. Shi, X.-G. Chen, Y.-M. You and R.-G. Xiong, Science, 2019, 363, 1206 CrossRef CAS PubMed.
- R. A. Wiscons, V. Coropceanu and A. J. Matzger, Chem. Mater., 2019, 31, 6598–6604 CrossRef CAS.
- J.-H. Dou, Z.-A. Yu, J. Zhang, Y.-Q. Zheng, Z.-F. Yao, Z. Tu, X. Wang, S. Huang, C. Liu, J. Sun, Y. Yi, X. Cao, Y. Gao, J.-Y. Wang and J. Pei, J. Am. Chem. Soc., 2019, 141, 6561–6568 CrossRef CAS PubMed.
- S. P. Thomas, R. Thomas, T. B. E. Gronbech, M. Bondesgaard, A. H. Mamakhel, V. Birkedal and B. B. Iversen, J. Phys. Chem. Lett., 2021, 3059–3065, DOI:10.1021/acs.jpclett.1c00614.
- X. Han, Y. Lei, Q. Liao and H. Fu, Angew. Chem., Int. Ed. Engl., 2021, 60, 3037–3046 CrossRef CAS PubMed.
- C. W. Tang and S. A. VanSlyke, Appl. Phys. Lett., 1987, 51, 913–915 CrossRef CAS.
- C. H. Chen and J. Shi, Coord. Chem. Rev., 1998, 171, 161–174 CrossRef CAS.
- V. A. Montes, R. Pohl, J. Shinar and P. Anzenbacher Jr, Chem.–Eur J., 2006, 12, 4523–4535 CrossRef CAS PubMed.
- J. P. Heiskanen and O. E. O. Hormi, Tetrahedron, 2009, 65, 8244–8249 CrossRef CAS.
- C. Dai, Z. Wei, Z. Chen, X. Yan, J. Fan, Y. Yang, Z. Pang and S. Han, ACS Appl. Energy Mater., 2018, 1, 4367–4373 CrossRef CAS.
- M. Rajeswaran, T. N. Blanton, C. W. Tang, W. C. Lenhart, S. C. Switalski, D. J. Giesen, B. J. Antalek, T. D. Pawlik, D. Y. Kondakov, N. Zumbulyadis and R. H. Young, Polyhedron, 2009, 28, 835–843 CrossRef CAS.
- R. Katakura and Y. Koide, Inorg. Chem., 2006, 45, 5730–5732 CrossRef CAS PubMed.
- C. F. R. A. C. Lima, R. J. S. Taveira, J. C. S. Costa, A. M. Fernandes, A. Melo, A. M. S. Silva and L. M. N. B. F. Santos, Phys. Chem. Chem. Phys., 2016, 18, 16555–16565 RSC.
- Y. Lei, Y. Sun, Y. Zhang, H. Zhang, H. Zhang, Z. Meng, W.-Y. Wong, J. Yao and H. Fu, Nat. Commun., 2018, 9, 4358 CrossRef PubMed.
- M. A. Spackman and D. Jayatilaka, CrystEngComm, 2009, 11, 19–32 RSC.
- M. J. Turner, S. P. Thomas, M. W. Shi, D. Jayatilaka and M. A. Spackman, Chem. Commun., 2015, 51, 3735–3738 RSC.
- M. Brinkmann, B. Fite, S. Pratontep and C. Chaumont, Chem. Mater., 2004, 16, 4627–4633 CrossRef CAS.
- S.-Y. Hung, R.-L. Kao, K.-Y. Lin, C.-C. Yang, K.-S. Lin, Y.-C. Chao, J.-S. Wang, J.-L. Shen and K.-C. Chiu, Mater. Chem. Phys., 2015, 154, 100–106 CrossRef CAS.
- M. Halls and R. Aroca, Can. J. Chem., 1998, 76, 1730–1736 CAS.
- J. H. Nobbs, Rev. Prog. Coloration Relat. Top., 1985, 15, 66–75 CrossRef.
- J. Tauc, Mater. Res. Bull., 1968, 3, 37–46 CrossRef CAS.
- P. Makuła, M. Pacia and W. Macyk, J. Phys. Chem. Lett., 2018, 9, 6814–6817 CrossRef PubMed.
- J. G. Mahakhode, S. J. Dhoble, C. P. Joshi and S. V. Moharil, Bull. Mater. Sci., 2011, 34, 1649–1651 CrossRef CAS.
- B. J. Chen, X. W. Sun and Y. K. Li, Appl. Phys. Lett., 2003, 82, 3017–3019 CrossRef CAS.
- R. I. Avetisov, A. A. Akkuzina, A. G. Cherednichenko, A. V. Khomyakov and I. C. Avetissov, Dokl. Chem., 2014, 454, 6–8 CrossRef CAS.
- A. R. Freitas, M. Silva, M. L. Ramos, L. L. G. Justino, S. M. Fonseca, M. M. Barsan, C. M. A. Brett, M. R. Silva and H. D. Burrows, Dalton Trans., 2015, 44, 11491–11503 RSC.
- W. Yang, X. Chen, H. Su, W. Fang and Y. Zhang, Chem. Commun., 2015, 51, 9616–9619 RSC.
- A. W. Varnes, R. B. Dodson and E. L. Wehry, J. Am. Chem. Soc., 1972, 94, 946–950 CrossRef CAS PubMed.
- H. S. Jung, P. S. Kwon, J. W. Lee, J. I. Kim, C. S. Hong, J. W. Kim, S. Yan, J. Y. Lee, J. H. Lee, T. Joo and J. S. Kim, J. Am. Chem. Soc., 2009, 131, 2008–2012 CrossRef CAS PubMed.
- S. Pal, N. Chatterjee and P. K. Bharadwaj, RSC Adv., 2014, 4, 26585–26620 RSC.
Footnotes |
† Electronic supplementary information (ESI) available. See DOI: 10.1039/d1sc03714e |
‡ These authors contributed equally. |
§ Current address: Department of Chemistry, Indian Institute of Technology Delhi, New Delhi-110016, India. |
|
This journal is © The Royal Society of Chemistry 2021 |
Click here to see how this site uses Cookies. View our privacy policy here.