DOI:
10.1039/D1SC03727G
(Edge Article)
Chem. Sci., 2021,
12, 11652-11658
Single-crystal-to-single-crystal synthesis of a pseudostarch via topochemical azide–alkyne cycloaddition polymerization†
Received
8th July 2021
, Accepted 5th August 2021
First published on 6th August 2021
Abstract
There is high demand for polysaccharide-mimics as enzyme-stable substitutes for polysaccharides for various applications. Circumventing the problems associated with the solution-phase synthesis of such polymers, we report here the synthesis of a crystalline polysaccharide-mimic by topochemical polymerization. By crystal engineering, we designed a topochemically reactive crystal of a glucose-mimicking monomer decorated with azide and alkyne units. In the crystal, the monomers arrange in head-to-tail fashion with their azide and alkyne groups in a ready-to-react antiparallel geometry, suitable for their topochemical azide–alkyne cycloaddition (TAAC) reaction. On heating the crystals, these pre-organized monomer molecules undergo regiospecific TAAC polymerization, yielding 1,4-triazolyl-linked pseudopolysaccharide (pseudostarch) in a single-crystal-to-single-crystal manner. This crystalline pseudostarch shows better thermal stability than its amorphous form and many natural polysaccharides.
Introduction
There is a growing interest in synthesizing polymers modeled after natural polysaccharides in view of their improved properties, enzymatic stability, etc.1 Syntheses of several pseudopolysaccharides containing monosaccharide units or their mimics connected by unnatural linkages have been achieved.1b,2 Inositols (hexahydroxy cyclohexanes),3 being structural mimics of hexose sugars, are attractive monomers for the synthesis of pseudopolysaccharides.4 However, unlike monosaccharides, which can homopolymerize in view of their electrophilic (carbonyl) and nucleophilic (hydroxyl groups) functionalities, inositols do not have such complementary reactive groups and require an external linker to form polymers. The 1,2,3-triazole unit is a robust, unnatural linker that confers many attractive properties to polymers.5 The use of conventional solution-phase synthesis of triazoles for polymer synthesis is limited due to the difficult purification of the polymer from catalysts, reagents and side products.6,7 Circumventing these issues, here we report the uncatalyzed, regiospecific single-crystal-to-single-crystal (SCSC) synthesis of a starch-like pseudopolysaccharide (Fig. 1) from myo-inositol via regiospecific topochemical azide–alkyne cycloaddition (TAAC) polymerization.
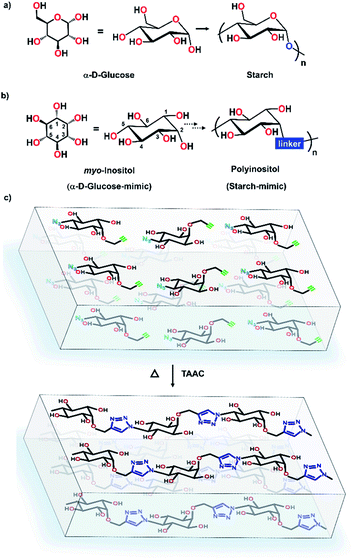 |
| Fig. 1 (a and b) Structural similarities of α-D-glucose and myo-inositol & starch and polyinositol. (c) Schematic representation of SCSC synthesis of polyinositol. | |
Results and discussion
Design and synthesis of monomer 1
Topochemical polymerization, lattice-controlled polymerization reactions driven by proximally placed reactive groups of monomers in the crystal, offers a solvent-free and catalyst-free method for making homogeneous, stereoregular crystalline polymers in high yield.8,9 The main challenge in the area of topochemical reactions lies in the engineering of the crystal packing to position the reacting groups at proximity in an orientation suitable for their reaction.10 Cyclohexane/pyranoside derivatives having vicinal anti-diol consisting of two equatorial hydroxy groups consistently arrange in a zigzag fashion forming hydrogen-bonded 1D-chains, in their crystals (Fig. 2a, ESI, Section 2†).11 We envisioned that strategically functionalizing the 2- and 5- positions of myo-inositol with complementary reactive groups (CRGs) viz. azide and alkyne, would yield tetrol 1 having two such vicinal diol units on opposite sides (Fig. 2b). This tetrol would crystallize to form a two-dimensional hydrogen-bonded layered network and stacking of such layers along the third dimension would place the azide and alkyne from adjacent layers at proximity (Fig. 2c and d). Such an arrangement would facilitate TAAC polymerization, along the direction perpendicular to the hydrogen-bonded 2D-layer, to form triazole-linked polyinositol (Fig. 2d).
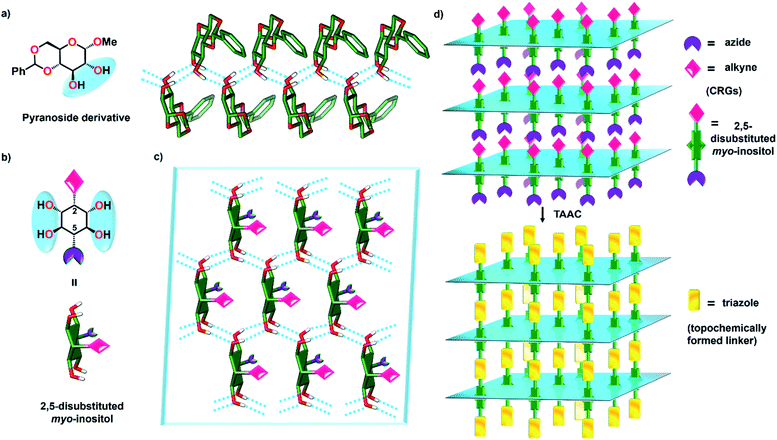 |
| Fig. 2 (a) 1D hydrogen-bonded zigzag arrangement in crystal structures of a representative pyranoside derivative possessing diequatorial vicinal diol. (b) myo-Inositol derivative decorated with CRGs at 2- and 5-positions. (c) Expected 2D hydrogen-bonded layer in the myo-inositol derivative. (d) Proposed head-to-tail arrangement of myo-inositol derivatives having CRGs at 2- & 5-positions and their topochemical polymerization reaction. | |
To test this hypothesis, we have synthesized tetrol 1 (Scheme 1, ESI, Section 3†). Briefly, myo-inositol (3) was protected to yield the symmetric ditriflate 5, which on selective sequential nucleophilysis with azide followed by DMSO resulted in azido-myo-inositol derivative 7.12 Propargylation of the remaining –OH group, followed by acidic hydrolysis yielded tetrol 1 as a white solid. We obtained the plate-like crystals (mp 317 °C) of tetrol 1via slow evaporation of its saturated solution in acetonitrile.
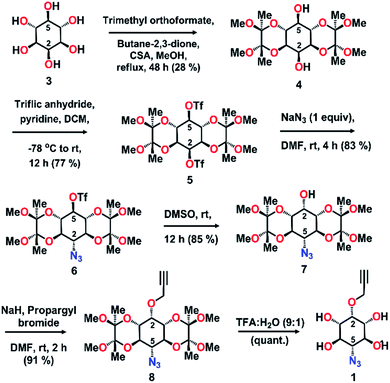 |
| Scheme 1 Synthesis of tetrol 1. | |
Single-crystal X-ray diffraction (SCXRD) analysis revealed that the tetrol 1 crystallizes in P21/n space group with one molecule in the asymmetric unit (Fig. 3a and Table S1†). In the crystal, the inositol ring adopts the chair conformation. The hydroxyl groups of each vicinal diol motif make intermolecular hydrogen bonding forming a hydrogen-bonded 2D-layer in the ‘ac’ plane, as anticipated (Fig. 3c). Such layers stack along ‘b’ direction and molecules in adjacent layers connect through CH⋯O hydrogen bonds (C9H9⋯O3, Table S2†). This stacked packing places the azide and alkyne groups of molecules in adjacent layers at proximity (3.37–3.43 Å) in a ready-to-react antiparallel arrangement (Fig. 3d).13 The antiparallel arrangement suggests the possibility of forming 1,4-triazolyl linked polyinositol along the stacking direction.
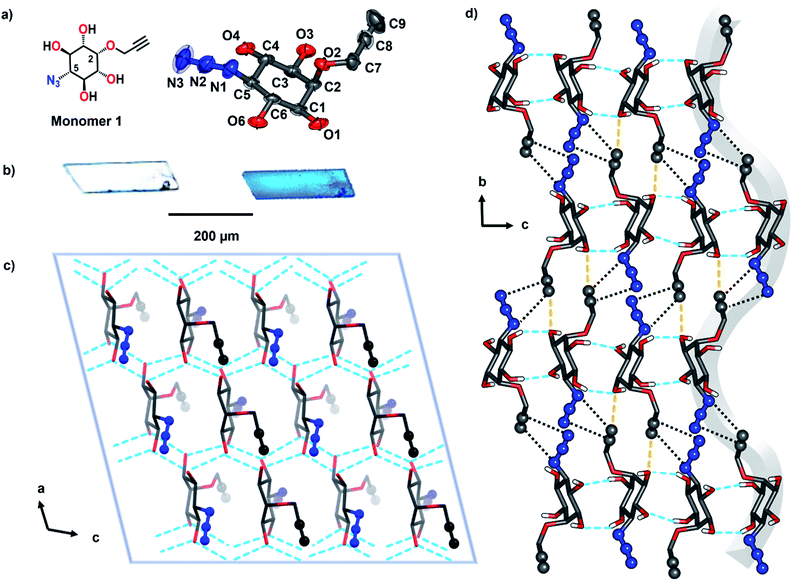 |
| Fig. 3 (a) Chemical structure and ORTEP diagram of tetrol 1. The thermal ellipsoids are set at 50% probability. (b) Photograph of the tetrol 1 single crystal taken under normal light and a polarizer. (c) Crystal packing of the tetrol 1 in ‘ac’ plane showing a 2D hydrogen-bonded layer. (d) Crystal packing viewed along ‘a’ direction. The OH⋯O hydrogen bonds and the CH⋯O hydrogen bonds are shown as blue and orange dotted lines, respectively. Black dotted lines represent the short contacts between the reactive terminals of antiparallelly arranged azide and alkyne units. The direction of polymer chain growth is along the wavy line (along ‘b’ direction) shown in the background. H-atoms (except OH) are hidden for clarity. | |
SCSC polymerization of monomer 1
Crystals of tetrol 1 are stable at room temperature but undergo reaction at higher temperatures (80 °C and above). We have monitored the progress of the solid-state reaction using time-dependent 1H NMR spectroscopy. For this, we kept crystals of the monomer 1 at 80 °C and small portions were withdrawn at various time intervals and subsequently analyzed by 1H NMR (ESI, Section 5†). By the time-dependent 1H NMR spectral analysis, the start of the reaction was assessed from the appearance of triazolyl proton signals in the region 7.8–8.0 ppm. With the progress of time, a gradual decrease in intensity of peaks corresponding to the monomer and the concomitant increase in the intensity of peaks corresponding to the product were observed (Fig. S2†). By 140 h, the monomer was completely consumed and a single sharp triazolyl proton signal at 7.96 ppm revealed the regiospecificity of the reaction. The 1H NMR and 13C NMR spectra of the polymer 2 were very clear, with distinct signals for each proton and carbon atom of the repeating unit, due to the linkage-homogeneity (Fig. 4). Structural analysis using various NMR techniques revealed the presence of only 1,4-triazolyl linkages between the inositol units (ESI, Section 6†). Such a regiospecificity in this uncatalyzed solid-state reaction is possible only under strict lattice-control. It is to be noted that the uncatalyzed thermal cycloaddition reaction (Huisgen reaction) in solution proceeds without any selectivity and leads to the formation of a heterogeneous mixture of products (ESI, Section 7†), but Cu(I)-catalyzed (click) reaction in solution yields a mixture of 1,4-triazolyl-linked cyclic oligomers. The solid-state reaction followed a sigmoidal kinetics with a lag phase (0–48 h), followed by an exponential growth phase (48–120 h) and a plateau phase (Fig. S2†).
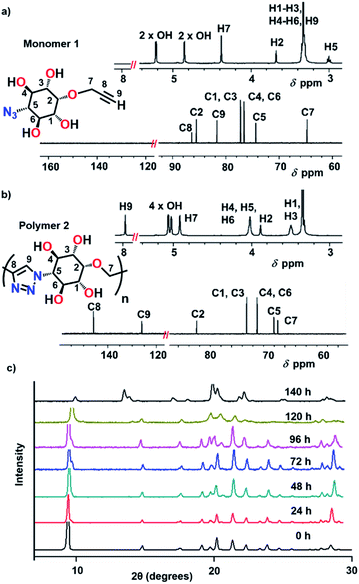 |
| Fig. 4
1H NMR and 13C NMR spectra of (a) monomer 1 and (b) polymer 2 recorded in DMSO-d6. (c) Time-dependent PXRD analyses of monomer 1 heated at 80 °C. | |
We have monitored the crystallinity throughout the reaction by recording PXRD profiles of the crystals withdrawn at different stages of reaction. Sharp signals were observed at every stage of the reaction suggesting that the crystallinity is maintained throughout the reaction. The peaks corresponding to the monomer crystal gradually disappeared or shifted and new sharp signals appeared with time (Fig. 4c). From the time-dependent PXRD data, it is clear that the monomer gradually converts to the polymer 2 in a crystal-to-crystal fashion. The morphology of the smaller crystals (length ∼ 200 μm) was unaffected even after the complete reaction. Optical polarizing microscopy of the fully reacted crystals showed a birefringence pattern suggestive of its single crystalline nature (Fig. 5b). Though rare, such reactions that occur in a SCSC manner allow structure determination of the product by SCXRD.14
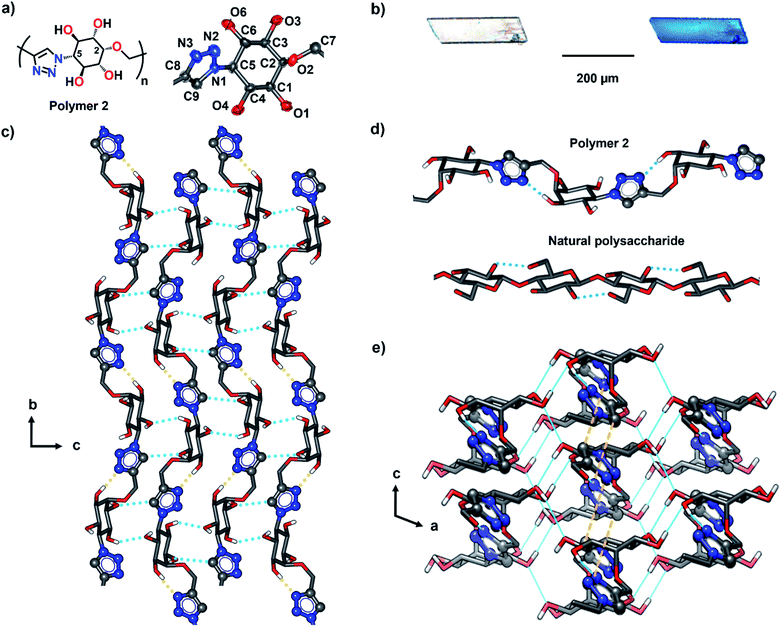 |
| Fig. 5 (a) Chemical structure and ORTEP diagram of the polymer 2. The thermal ellipsoids are set at 50% probability. (b) Photograph of the polymer single crystal taken under normal light and a polarizer. (c) Packing of polymer chains in ‘bc’ plane showing reaction between the hydrogen-bonded layers (along ‘b’ direction). The blue dotted lines represent OH⋯O and CH⋯O hydrogen bonds and the yellow dotted lines represent intra-chain OH⋯N hydrogen bonds. (d) Comparison of the chain conformations of polymer 2 and a natural polysaccharide. The intrachain hydrogen bonds are represented in blue lines. (e) Packing of polymer chains viewed along ‘b’ direction, showing interchain hydrogen bonds. The triazole ring is represented in a ball-and-stick model and the H atoms (except OH) are hidden for clarity. | |
Structure and properties of polymer 2
We determined the crystal structure of the fully reacted crystals by SCXRD analysis (monoclinic P21/c with Z = 4). As anticipated, TAAC reaction occurred regiospecifically in SCSC fashion and resulted in the formation of 1,4-triazolyl linked polymer 2 exclusively (Fig. 5 and Table S1†). Also, the TAAC reaction occurred along ‘b’ direction connecting the hydrogen-bonded layers (Fig. 5c). In the crystal, each polymer chain adopts a wavy conformation and the adjacent inositol units are cork-screwed 180° as in the case of natural polysaccharides (Fig. 5d). Intramolecular OH⋯N hydrogen bonding between the triazole linker and two inositol units on its either side stabilize this conformation (Fig. 5d). This is similar to the intra-chain hydrogen bonding between the adjacent monomers in natural polysaccharides.15 All the four hydroxyl groups in the repeating unit are involved in intermolecular (interchain) hydrogen bonding. Each polymer chain makes OH⋯O hydrogen bonding with four neighbouring chains along ‘a’ direction and weak CH⋯N hydrogen bonds with two other neighbouring molecules along ‘c’ direction (Fig. 5e). For the polymerization to occur, it is necessary that both the azide and alkyne moieties move closer.
A comparison of the arrangement of molecules in the monomer and polymer crystal structures reveals that upon polymerization, the distance between the adjacent monomer units decreased by almost 1 Å (Fig. S8 and S9†). This molecular motion results in a change in unit-cell dimensions of the polymer from that of the monomer. Since the reaction occurs along the ‘b’ direction, the unit cell parameter ‘b’ decreases by 9.2% and the parameters ‘a’ and ‘c’ increase by 2.1% and 6.7% respectively, resulting in an overall decrease in the volume of the unit cell by 3.1% (Table S1 and Fig. S8†).
The polymer 2 crystals are soluble in DMSO and N-methylpyrrolidone. A MALDI-TOF MS analysis of the polymer 2 was carried out, which showed presence of up to 43-mer of the polyinositol (Fig. 6a). We also found that oligomers up to 8-mer are soluble in water (ESI, Section 9†). Gel permeation chromatography (GPC) analysis of the fully reacted sample revealed a unimodal distribution (dispersity, Đ ∼ 3), a number-average molar mass of 4.9 kg mol−1 and the weight-average molar mass was calculated as 14.2 kg mol−1 (Fig. 6b, ESI, Section 10†). Evaporation of a DMSO solution of the polymer yields opaque polymer with a film-like texture (Fig. 6c, ESI, Section 11†). PXRD analysis of the film revealed its amorphous nature (Fig. 6d). We investigated the thermal stabilities of both the crystalline and amorphous forms of the polymer 2 using thermogravimetric analysis (TGA). While the crystals of the polymer are stable up to 344 °C, the amorphous polymer decomposed at a lower temperature of 312 °C (Fig. 6e). Thus, it is clear that the crystalline polymer shows higher thermal stability than its amorphous form. The close and ordered packing due to hydrogen bonds is responsible for the enhanced thermal stability of the crystalline polymer. Also, the crystalline polymer 2 showed better thermal stability than many natural polysaccharides.16
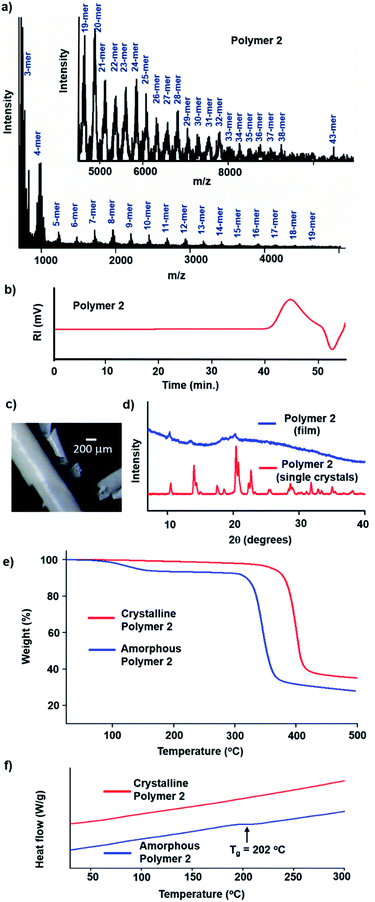 |
| Fig. 6 (a) MALDI spectrum and (b) GPC elution curve for the polymer 2. (c) Photograph of the polymer 2 film. (d) Comparison of PXRD patterns of polymer 2 single crystals and polymer 2 film. Comparison of (e) TGA thermograms and (f) DSC thermograms of crystalline and amorphous polymer 2 (heating rate: 5 °C min−1). | |
Differential scanning calorimetry (DSC) analysis of the crystalline polymer 2 did not show any glass transition (Fig. 6f) as anticipated in view of its crystalline nature, similar to crystalline amylose.17 In contrast, the amorphous polymer 2 undergoes a glass transition at 202 °C, comparable to gelatinized starch.18 Polymers with such high-glass transition temperatures are important in the context of stable plastics required for several applications.19
Conclusions
In conclusion, circumventing the problems associated with the conventional solution-phase polymer synthesis, we have adopted a solid-state synthesis for the polymerization of a cyclitol-derived monomer. By applying crystal-engineering principles and taking a cue from the unique packing of a class of pyranose derivatives, we designed a cyclitol monomer that crystallizes such that its molecules arrange in head-to-tail fashion with the proximal placement of the azide and alkyne units of adjacent molecules. Upon heating these crystals, the monomer undergoes SCSC TAAC polymerization, in a regiospecific manner, to yield an enzyme-stable, crystalline pseudopolysaccharide, poly-1,4-triazolyl-myo-inositol. Despite solvent-free and catalyst-free conditions, due to the confinement offered by the crystal lattice, the reaction was not only regiospecific but also yielded linear polymer. This polycarbasugar shares key characteristics of natural polysaccharides, including the preservation of stereochemistry, dense hydroxyl functionality, and rigid backbone, in addition to being enzyme-stable, possessing higher thermal stability and higher glass transition temperature, making it a better candidate for many applications. This is the first successful synthesis of a polymer of a cyclitol using topochemical polymerization. This report exemplifies the fidelity of crystal engineering in designing topochemical reactions in general and in the synthesis of crystalline polymers. Given the availability of many structurally diverse carbasugars such as nine inositols,3 conduritols, quercitols, other numerous naturally occurring cyclitols,20 the possibility of extension of TAAC polymerization approach to make structurally and functionally diverse pseudopolysaccharides is huge.
Data availability
Crystallographic data for monomer 1 and polymer 2 have been deposited at the CCDC under accession numbers 2038869 and 2038870, respectively.†
Author contributions
KMS conceived the idea and designed the project. AR and AS performed the experiments. AR and KMS analysed the results and wrote the manuscript.
Conflicts of interest
There are no conflicts to declare.
Acknowledgements
KMS thanks the Department of Science and Technology, Govt. of India for a SwarnaJayanti Fellowship (DST/SJF/CSA02/2012-13) & Science and Engineering Research Board for a research grant (SERB/CRG/000577/2018).
References
-
(a) J. A. Galbis, M. d. G. García-Martín, M. V. de Paz and E. Galbis, Chem. Rev., 2016, 116, 1600–1636 CrossRef CAS PubMed
;
(b) R. Xiao and M. W. Grinstaff, Prog. Polym. Sci., 2017, 74, 78–116 CrossRef CAS
;
(c)
Y. Yu and M. Delbianco, in Recent Trends in Carbohydrate Chemistry, ed. A. P. Rauter, B. E. Christensen, L. Somsák, P. Kosma and R. Adamo, Elsevier, 2020, ch. 9, pp. 333–371 Search PubMed
.
-
(a) M. Metzke, J. Z. Bai and Z. Guan, J. Am. Chem. Soc., 2003, 125, 7760–7761 CrossRef CAS PubMed
;
(b) E. L. Dane and M. W. Grinstaff, J. Am. Chem. Soc., 2012, 134, 16255–16264 CrossRef CAS PubMed
;
(c) K. Mikami, A. T. Lonnecker, T. P. Gustafson, N. F. Zinnel, P.-J. Pai, D. H. Russell and K. L. Wooley, J. Am. Chem. Soc., 2013, 135, 6826–6829 CrossRef CAS PubMed
;
(d) A. S. Balijepalli and M. W. Grinstaff, Acc. Chem. Res., 2020, 53, 2167–2179 CrossRef CAS PubMed
;
(e) T. M. McGuire, J. Bowles, E. Deane, E. H. E. Farrar, M. N. Grayson and A. Buchard, Angew. Chem., Int. Ed., 2021, 60, 4524–4528 CrossRef CAS PubMed
.
- M. P. Thomas, S. J. Mills and B. V. L. Potter, Angew. Chem., Int. Ed., 2016, 55, 1614–1650 CrossRef CAS PubMed
.
-
(a) P. Wessig and K. Möllnitz, J. Org. Chem., 2012, 77, 3907–3920 CrossRef CAS PubMed
;
(b) T. Shimokawaji and A. Sudo, J. Polym. Sci. Part A: Polym. Chem., 2020, 58, 1229–1235 CAS
.
-
(a) J.-F. Lutz, Angew. Chem., Int. Ed., 2007, 46, 1018–1025 CrossRef CAS PubMed
;
(b) A. Qin, J. W. Y. Lam and B. Z. Tang, Chem. Soc. Rev., 2010, 39, 2522–2544 RSC
;
(c)
J. K. Fink, in High Performance Polymers, ed. J. K. Fink, William Andrew Publishing, 2nd edn, 2014, ch. 9, pp. 221–240 Search PubMed
.
- C. J. Pickens, S. N. Johnson, M. M. Pressnall, M. A. Leon and C. J. Berkland, Bioconjug. Chem., 2018, 29, 686–701 CrossRef CAS PubMed
.
-
(a) K. D. Bodine, D. Y. Gin and M. S. Gin, J. Am. Chem. Soc., 2004, 126, 1638–1639 CrossRef CAS PubMed
;
(b) M. V. Rivas, G. Petroselli, R. Erra-Balsells, O. Varela and A. A. Kolender, RSC Adv., 2019, 9, 9860–9869 RSC
.
-
(a) K. Hema, A. Ravi, C. Raju, J. R. Pathan, R. Rai and K. M. Sureshan, Chem. Soc. Rev., 2021, 50, 4062–4099 RSC
;
(b) L. Zhu, H. Tran, F. L. Beyer, S. D. Walck, X. Li, H. Ågren, K. L. Killops and L. M. Campos, J. Am. Chem. Soc., 2014, 136, 13381–13387 CrossRef CAS PubMed
;
(c) Y. L. Li, C.-T. Zee, J. B. Lin, V. M. Basile, M. Muni, M. D. Flores, J. Munárriz, R. B. Kaner, A. N. Alexandrova, K. N. Houk, S. H. Tolbert and Y. Rubin, J. Am. Chem. Soc., 2020, 142, 18093–18102 CrossRef CAS PubMed
;
(d) I. Levesque, J. R. Néabo, S. Rondeau-Gagné, C. Vigier-Carrière, M. Daigle and J.-F. Morin, Chem. Sci., 2014, 5, 831–836 RSC
.
-
(a) A. Sun, J. W. Lauher and N. S. Goroff, Science, 2006, 312, 1030 CrossRef CAS PubMed
;
(b) T. Itoh, T. Suzuki, T. Uno, M. Kubo, N. Tohnai and M. Miyata, Angew. Chem., Int. Ed., 2011, 50, 2253–2256 CrossRef CAS PubMed
;
(c) T.-J. Hsu, F. W. Fowler and J. W. Lauher, J. Am. Chem. Soc., 2012, 134, 142–145 CrossRef CAS PubMed
;
(d) A. Pathigoolla, R. G. Gonnade and K. M. Sureshan, Angew. Chem., Int. Ed., 2012, 51, 4362–4366 CrossRef CAS PubMed
;
(e) L. Dou, Y. Zheng, X. Shen, G. Wu, K. Fields, W.-C. Hsu, H. Zhou, Y. Yang and F. Wudl, Science, 2014, 343, 272–277 CrossRef CAS PubMed
;
(f) R. Z. Lange, G. Hofer, T. Weber and A. D. Schlüter, J. Am. Chem. Soc., 2017, 139, 2053–2059 CrossRef CAS PubMed
;
(g) X. Jiang, X. Cui, A. J. E. Duncan, L. Li, R. P. Hughes, R. J. Staples, E. V. Alexandrov, D. M. Proserpio, Y. Wu and C. Ke, J. Am. Chem. Soc., 2019, 141, 10915–10923 CrossRef CAS PubMed
;
(h) Q.-H. Guo, M. Jia, Z. Liu, Y. Qiu, H. Chen, D. Shen, X. Zhang, Q. Tu, M. R. Ryder, H. Chen, P. Li, Y. Xu, P. Li, Z. Chen, G. S. Shekhawat, V. P. Dravid, R. Q. Snurr, D. Philp, A. C. H. Sue, O. K. Farha, M. Rolandi and J. F. Stoddart, J. Am. Chem. Soc., 2020, 142, 6180–6187 CrossRef PubMed
;
(i) F. Hu, W. Hao, D. Mücke, Q. Pan, Z. Li, H. Qi and Y. Zhao, J. Am. Chem. Soc., 2021, 143, 5636–5642 CrossRef CAS PubMed
.
-
(a) S.-Y. Yang, X.-L. Deng, R.-F. Jin, P. Naumov, M. K. Panda, R.-B. Huang, L.-S. Zheng and B. K. Teo, J. Am. Chem. Soc., 2014, 136, 558–561 CrossRef CAS PubMed
;
(b) K. Biradha and R. Santra, Chem. Soc. Rev., 2013, 42, 950–967 RSC
;
(c) S. Kusaka, A. Kiyose, H. Sato, Y. Hijikata, A. Hori, Y. Ma and R. Matsuda, J. Am. Chem. Soc., 2019, 141, 15742–15746 CrossRef CAS PubMed
;
(d) I. E. Claassens, L. J. Barbour and D. A. Haynes, J. Am. Chem. Soc., 2019, 141, 11425–11429 CrossRef CAS PubMed
;
(e) S. P. Yelgaonkar, G. Campillo-Alvarado and L. R. MacGillivray, J. Am. Chem. Soc., 2020, 142, 20772–20777 CrossRef CAS PubMed
;
(f) J. J. Dotson, I. Liepuoniute, J. L. Bachman, V. M. Hipwell, S. I. Khan, K. N. Houk, N. K. Garg and M. A. Garcia-Garibay, J. Am. Chem. Soc., 2021, 143, 4043–4054 CrossRef CAS PubMed
.
- R. Luboradzki, O. Gronwald, M. Ikeda, S. Shinkai and D. N. Reinhoudt, Tetrahedron, 2000, 56, 9595–9599 CrossRef CAS
.
- A. Ravi and K. M. Sureshan, Angew. Chem., Int. Ed., 2018, 57, 9362–9366 CrossRef CAS PubMed
.
- G. M. J. Schmidt, Pure Appl. Chem., 1971, 27, 647–678 CAS
.
-
(a)
U. Muller, in Symmetry Relationships between Crystal Structures: Applications of Crystallographic Group Theory in Crystal Chemistry, Oxford University Press, 2013, ch. 11, pp. 217–224 CrossRef
;
(b) A. D. Schlüter, T. Weber and G. Hofer, Chem. Soc. Rev., 2020, 49, 5140–5158 RSC
.
- Y. Nishiyama, J. Sugiyama, H. Chanzy and P. Langan, J. Am. Chem. Soc., 2003, 125, 14300–14306 CrossRef CAS PubMed
.
-
(a) S. S. Kim, S. J. Kim, Y. D. Moon and Y. M. Lee, Polymer, 1994, 35, 3212–3216 CrossRef CAS
;
(b) M. A. Villetti, J. S. Crespo, M. S. Soldi, A. T. N. Pires, R. Borsali and V. Soldi, J. Therm. Anal. Calorim., 2002, 67, 295–303 CrossRef CAS
;
(c) H. Yang, R. Yan, H. Chen, D. H. Lee and C. Zheng, Fuel, 2007, 86, 1781–1788 CrossRef CAS
;
(d) X. Liu, Y. Wang, L. Yu, Z. Tong, L. Chen, H. Liu and X. Li, Starch/Stärke, 2013, 65, 48–60 CrossRef CAS
.
- M. Scandola, G. Ceccorulli and M. Pizzoli, Int. J. Biol. Macromol., 1991, 13, 254–260 CrossRef CAS PubMed
.
- A. Mizuno, M. Mitsuiki and M. Motoki, J. Agric. Food Chem., 1998, 46, 98–103 CrossRef CAS PubMed
.
- H. T. H. Nguyen, P. Qi, M. Rostagno, A. Feteha and S. A. Miller, J. Mat. Chem. A, 2018, 6, 9298–9331 RSC
.
-
Y. Kobayashi, in Glycoscience: Chemistry and Chemical Biology, ed. B. O. Fraser-Reid, K. Tatsuta and J. Thiem, Springer, Berlin, Heidelberg, 2008, pp. 1913–1997 Search PubMed
.
Footnote |
† Electronic supplementary information (ESI) available. CCDC 2038869 and 2038870. For ESI and crystallographic data in CIF or other electronic format see DOI: 10.1039/d1sc03727g |
|
This journal is © The Royal Society of Chemistry 2021 |
Click here to see how this site uses Cookies. View our privacy policy here.