DOI:
10.1039/D1SC04071E
(Edge Article)
Chem. Sci., 2021,
12, 13209-13215
Nickel-catalyzed enantioselective 1,2-vinylboration of styrenes†
Received
24th July 2021
, Accepted 7th September 2021
First published on 7th September 2021
Abstract
A novel nickel-catalyzed asymmetric 1,2-vinylboration reaction has been developed to afford benzylic alkenylboration products with high yields and excellent enantioselectivities by using a chiral bisoxazoline ligand. Under optimized conditions, a wide variety of chiral 2-boryl-1,1-arylvinylalkanes are efficiently prepared from readily available olefins and vinyl halides in the presence of bis(pinacolato)diboron as the boron source in a mild and easy-to-operate manner. This three-component cascade protocol furnishes exceptional chemo- and stereoselectivity, and its usefulness is illustrated by its application in asymmetric modifications of several structurally complex natural products and pharmaceuticals.
Introduction
Difunctionalization of alkenes through a transition-metal catalyzed process has gradually evolved as a significant opportunity for establishing two newly formed stereocenters in a single step, thus allowing rapid access to molecular complexity.1 Of these methods, the carboboration of alkene precursors is emerging as a versatile and efficient approach to prepare aliphatic boron intermediates.2 Due to the excellent accessibility of diverse functional groups from the carbon–boron bond, this method provides researchers compelling approaches to obtain boron-containing intermediates and substantial opportunities for downstream diversifications.3 In the past few decades, many efforts have been devoted to the study of several classes of alkene carboboration reactions,4 in which the reactions vary based on the electrophiles and catalysts employed,5 especially in the 1,2-arylboration of vinylarenes by an elegant dual metal synergistic catalysis.6–8 For incorporation of aryl groups, Cu/Pd-,7 Cu/Ni-,8 Pd-,9 or Cu-catalysis10 has been proven to be effective (Fig. 1, top). Recently, stimulated by the special reactivity of nickel,11 the transient alkyl-Ni species generated from olefin insertion into a Ni–B bond have also been investigated by Brown12 and Yin,13 affording racemic 1,2-arylboration examples (Fig. 1, middle). The majority of these methods utilize activated alkenes (e.g., alkenyl arenes, vinyl-silanes, and strained alkenes) to increase the rate of an insertion event prior to the direct reaction between the electrophiles and nucleophiles (e.g., B2pin2 or [M]-Bpin). In the nickel-catalyzed cross-coupling of C(sp2)-electrophiles, the formation of stable C(sp2)-Ni intermediates by oxidative addition of the electrophiles to low-stage Ni is generally involved.14 Thus, special alkyl benzylic substrates are competent for vinylation with vinyl halides, possibly due to matched reactivities.
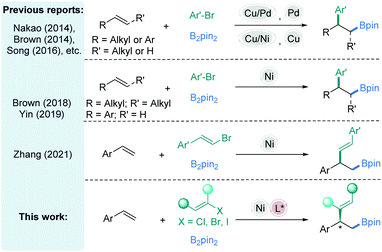 |
| Fig. 1 Transition-metal catalyzed carboboration of alkenes. | |
The nickel-catalyzed vinylboration of alkenylarenes is worth being developed. Recently, Zhang15f reported a nickel-catalyzed alkenylboration of alkenylarenes and vinyl bromides to obtain the racemic 2-boryl-1,1-arylvinylalkanes (Fig. 1, middle). We reasonably questioned whether the general strategy described above could be expanded to asymmetric vinylation when a classic cross-coupling partner, a vinyl halide,14,15 is used. Furthermore, carboboration of asymmetric alkenes is infrequent and the enantioselectivity and chemoselectivity of different classes of olefins are rarely discussed in a single system concurrently. From this perspective we explored a process for enantioselective 1,2-vinylboration of alkenes catalyzed by a chiral Ni-complex with intriguing results (Fig. 1, bottom).11a,16–19 More specifically, these reactions are highly chemo- and stereoselective, operated with near equimolar quantities of the alkenes and vinyl halides, and occur at mild temperature. Structurally complex substrates derived from natural products and approved drugs are well tolerated. The preliminary mechanistic studies are also discussed.
Results and discussion
Optimization of the reaction conditions
We initiated our investigation by exploring the enantioselective vinylboration of styrene (1) with (E)-(2-bromovinyl)benzene (2) and bis(pinacolato)diboron (B2pin2) as model substrates using chiral bis(oxazoline) ligands (Table 1). An extensive screening of the reaction parameters revealed that the use of NiBr2·DME (10 mol%), L1 (10 mol%), and B2pin2 (1.2 equiv.) as the boron source and LiOMe (1.5 equiv.) as the base in 1,4-dioxane at 10 °C delivered compound 3 in 85% yield and 94% ee (entry 1). Under the selected conditions, ligands appeared to have great influence on this asymmetric transformation, while replacing L1 with other chiral dibox ligands L2–L5 did not result in better outcomes in both yields and ees (entries 2–5). With other ligands such as 2,6-bis((S)-4-isopropyl-4,5-dihydrooxazol-2-yl)pyridine (iPr-Box), less desired vinylation products were produced (Table S1,† entries 8–12). Replacement of LiOMe by LiOtBu led to a decrease in yield and ee (entry 6). THF was shown to be an unsuitable solvent (entry 7). Reaction at 0 °C (entry 8) led to a comparable yield and ee, while somewhat lower yield and ee were obtained at 25 °C (entry 9). The addition of a pre-coordinated L1·NiBr2 complex (10 mol%) as the catalyst to replace NiBr2·DME (10 mol%) and L1 (10 mol%) led to evidently higher yield and ee (entry 10). Change of other parameters such as nickel sources led to inferior yields and enantioselectivities (Table S1,† entries 4–7).
Table 1 Optimization for the formation of 3
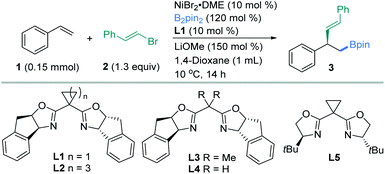
|
Entry |
Variation from standard conditionsa |
Yieldb [%] |
eec [%] |
Standard conditions: 1 (0.150 mmol, 1.0 equiv.), 2 (0.195 mmol, 1.3 equiv.), B2pin2 (0.180 mmol, 1.2 equiv.), NiBr2·DME (10 mol%), L1 (10 mol%), LiOMe (0.225 mmol, 1.5 equiv.), 1,4-dioxane (1.0 mL), 10 °C, 14 h.
Yields determined by crude 1H NMR using 2,5-dimethylfuran as the internal standard. The yield in parentheses is the isolated yield.
The ee values were determined by HPLC on a chiral stationary phase.
|
1 |
None |
85 |
94 |
2 |
L2 instead of L1 |
54 |
81 |
3 |
L3 instead of L1 |
34 |
48 |
4 |
L4 instead of L1 |
26 |
17 |
5 |
L5 instead of L1 |
20 |
73 |
6 |
LiOtBu instead of LiOMe |
50 |
85 |
7 |
THF instead of 1,4-dioxane |
38 |
89 |
8 |
0 °C |
80 |
93 |
9 |
25 °C |
53 |
80 |
10 |
L1·NiBr2 |
92 (93) |
96 |
Enantiomerically pure chiral ligands were used to explore the nickel-catalyzed vinylboration listed in Tables 1 and S1.† The ligand effects appear to be pivotally important to the yields and ees. Consequently, we tentatively speculate that L1, L2 and L5 adopting a rigid cycloalkyl linker features a larger bond angle of the C(oxazoline)–C(methylene)–C(oxazoline) bond than those in L3, L4 (entries 3 and 4) and L8 (Table S1,† entry 10), which appears to have a crucial effect on the enantioselectivity. Further comparison of the results arising from L1, L2 and L5 (entries 1, 2 and 5) provides a reasonable conclusion that the rigid tricyclic fused rings in L1 offer an optimal steric and rigidity requirement for enantioselective control. Systematic evaluation of various chiral ligands showed that both high enantiomeric excess and yields were obtained with a chiral nickel-bis(oxazoline) L1·NiBr2 (ref. 15a and 15b) for a wide range of vinyl halides with styrenes.20
Substrate scope
With the optimal reaction conditions in hand, we sought to explore the generality of this three-component reaction. Firstly, a wide range of β-aryl-substituted (E)-alkenyl bromides bearing electron-poor (4–9) or electron-rich substituents (10–17) on the arene afforded the desired product smoothly (Fig. 2). The electronic properties of alkenyl halides did not show an obvious effect on the efficiency of this transformation. Emphatically, (E)-alkenyl chloride (3b), (E)-alkenyl iodide (17) and (Z)-alkenyl bromide (12b) were shown to participate in the reaction to provide satisfactory results. In addition, β-naphthyl-substituted (E)-alkenyl bromide (18) was also proved to be compatible. Good coupling results with excellent enantioselectivities were also observed for β-heteroaromatic-substituted (E)-alkenyl bromides containing various functional groups or moieties such as indole (19), furan (20), thiophene (21) or pyridine (22, 23). However, a slight decrease in ee was observed when 1,3-dienyl bromide (24) was used as the substrate. Notably, α-alkyl substituted alkenyl bromides, such as 2-bromoindene (25), are also competent coupling partners, while β-alkyl-substituted (E)-alkenyl bromide (26) is an active substrate but with reduced ee. Nonetheless, aryl bromides such as methyl 4-bromobenzoate are not suitable in this asymmetric system for lack of reactivity.
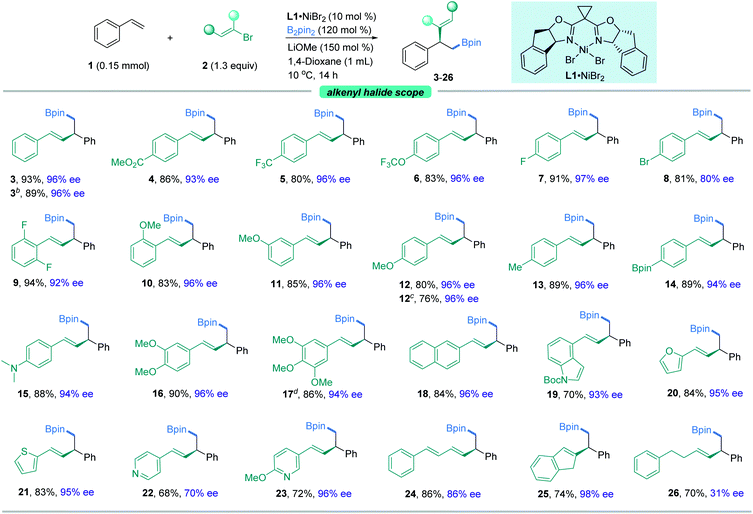 |
| Fig. 2 The scope of alkenyl halides. aThe standard reaction conditions; isolated yields are provided (average of 2 independent runs); ee is determined by chiral HPLC analysis. b(E)-(2-Chlorovinyl)benzene instead of 2. c(Z)-1-(2-Bromovinyl)-4-methoxybenzene instead of 2. d(E)-5-(2-Iodovinyl)-1,2,3-trimethoxybenzene instead of 2. | |
Our attention then shifted to the scope of the alkene partner. As shown in Fig. 3, styrenes bearing a variety of substituents on the aromatic ring, both electron-deficient ones such as esters, aldehydes, trifluoromethyl moieties and halides (27–37) and electron-rich ones (38–42), underwent this vinylboration smoothly with high levels of enantiocontrol. Signally, this asymmetric catalyst system couples an alkenyl bromide in the presence of an aryl halide (F, Cl, Br, or I) with moderate to high selectivity (31–37). Notably, the existence of the fluorine group gave excellent ee compared to its Cl−, Br−, and I− counterparts. Under these exceptionally mild reaction conditions, even a sensitive functional group like a boronic acid pinacol ester (39) remained intact. It was found that ortho-substituted styrenes (33, 35, 36, 42) were also suitable substrates but with slightly lower ees. Moreover, the naphthyl-containing olefin derived product (43) could be prepared by this method as well in good enantioselectivity. Besides, heterocycles such as benzothiophene (44), benzofuran (45), thiophene (46), furan (47), and pyridine (48) were also competent coupling partners. An internal alkene, such as indene (49), could also give rise to the cis-product in moderate yield with lower ee. Alkenylferrocene (50) could also undergo the reaction, providing access to good enantio-enriched ferrocene derivatives. Interestingly, substrates containing two symmetric vinyl groups such as 1,4-divinylbenzene could still make the transformation feasible to afford the double vinylboration product in good yield and ee (51). In this case, two chiral centers are established simultaneously in an efficient manner.
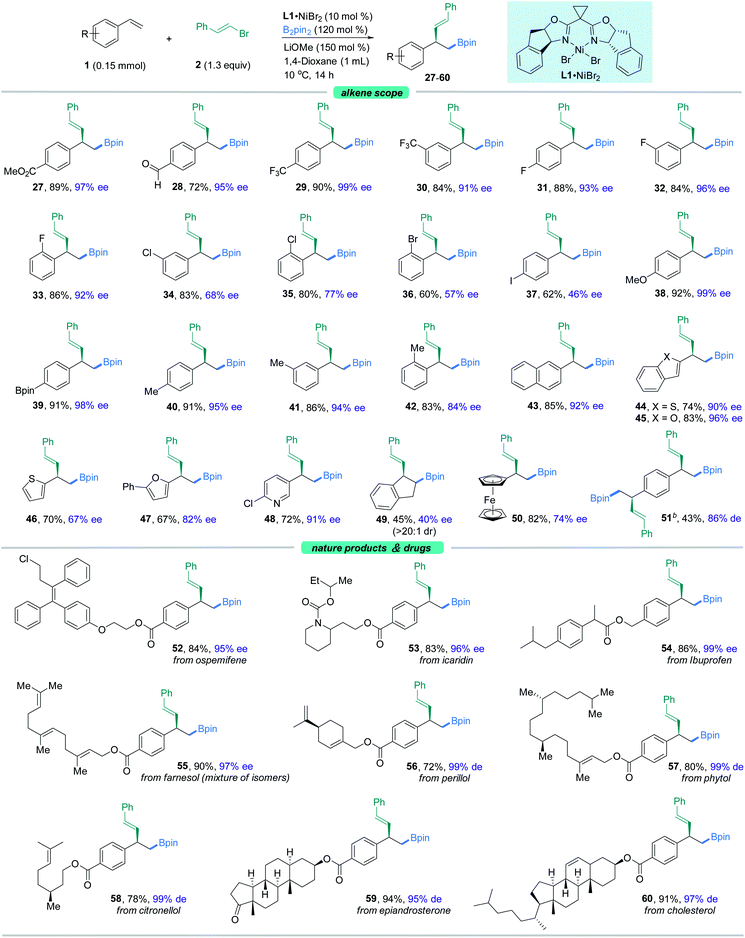 |
| Fig. 3 The scope of alkenes. aThe standard reaction conditions; isolated yields are provided (average of 2 independent runs); ee is determined by chiral HPLC analysis. b1,4-Divinylbenzene instead of styrene, 2.6 equiv. of 2 and 2.4 equiv. of B2pin2 were used. | |
To further expand the usefulness of this tactic, we attached the styrene moiety to a variety of more structurally complex drugs and natural products. Remarkably, all of these substrates examined in our work underwent the transformation in excellent yields and enantioselectivities (52–60). The reaction system is capable of distinguishing the activated olefin over the unactivated olefin, providing the site specific transformation and enantiocontrol. As shown in Fig. 3, the alkenyl derivatives from pharmaceuticals and repellents such as ospemifene (52), icaridin (53), and ibuprofen (54) were amenable to this reaction, demonstrating the viability of this nickel-catalyzed asymmetric 1,2-vinylboration for modifications of bioactive molecules. Derivatives from naturally occurring alcohols such as farnesol (55), perillol (56), phytol (57), citronellol (58), epiandrosterone (59), and cholesterol (60) were also competent substrates, enabling access to the desired adducts in synthetically useful yields and ees. It is worth noting that many of these substrates exhibit extremely high enantioselectivity, up to 99% ee.
Mechanistic consideration
Several competition experiments were conducted to compare the relative reactivities of different types of C
C bond (Fig. 4a). The vinylboration protocol described here exhibited excellent chemoselectivity when equal amounts of two olefins were present. In the first competition experiment with cyclohexene, only the product of 3 was isolated in 53% yield (Fig. 4a-1). In an independent reaction, cyclohexene showed no reactivity under the standard conditions either. These results indicated that the nitrogen-based ligand enabled a different reactivity from the prior ligand-free system.12b Furthermore, only product 3 from styrene was observed in 66% yield in the competition reaction with an unactivated monosubstituted olefin (Fig. 4a-2). Intriguingly, a high selectivity was also displayed in the competition reaction with allylbenzene (Fig. 4a-3). Accordingly, the addition of three olefins as above all sparingly inhibited the styrene vinylboration. However, the addition of a strong electron-deficient olefin, methyl acrylate, highly influenced the reactivity (Fig. 4a-4).
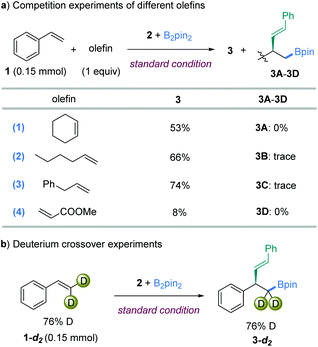 |
| Fig. 4 Competition and deuterium experiments. | |
To gain insight into the details of the olefin insertion step, a terminal D-labeled olefin 1-d2 was synthesized and examined in the vinylboration reaction. The product 3-d2 was isolated in 90% yield, with almost no deuterium lost at the terminal position (Fig. 4b). These results combined with the exclusive cis-diastereoselectivity reveal that reversible β-H elimination does not likely occur in the transformation.
Next, intermolecular protoboration studies12b (Fig. S1 and S2†) supported the generation of the Ni(I) complex (III) (Fig. 5). The long-lived radical intermediates might not be involved in the reaction processes according to the control experiment results12c,15f (Fig. S3†). However, the electron paramagnetic resonance (EPR) experiment result was consistent with Yin's report.13b Accordingly, a proposed catalytic cycle was considered based on the present related literature12,13 and the preliminary mechanism researched to rationalize this transformation. As illustrated in Fig. 5, the reaction is initiated by the generation of a Ni(I) species (I) from the Ni(II) precatalyst through a comproportionation process, which undergoes transmetalation with B2pin2 to generate the Ni(I)-Bpin (II). Then, olefin migratory insertion in an anti-Markovnikov fashion leads to the formation of a stable benzyl-Ni(I) species (III),21 which subsequently reacts with a vinyl halide to deliver a Ni(III) intermediate IV. Eventually, reductive elimination from IV generates the asymmetric vinylboration product 3 and regenerates the Ni(I) species (I). However, we cannot exclude the pathway of bimetallic transmetalation between two nickel species at this stage.22,23
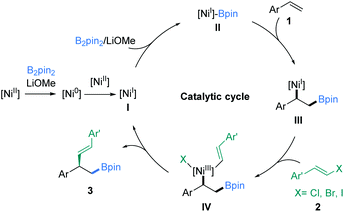 |
| Fig. 5 Proposed catalytic pathway. | |
Conclusions
In summary, we have developed a chiral bisoxazoline ligand-enabled, Ni-catalyzed asymmetric anti-Markovnikov vinylboration reaction. A set of 2-boryl-1,1-arylvinylalkanes, significant pharmaceutically interesting scaffolds, are efficiently synthesized from simple alkenes and vinyl halides in the presence of a diboron reagent under mild conditions. The present method is tolerant of a variety of functional groups, affording the coupling products generally in modest to high yields and excellent enantioselectivities. Significantly, this work provides a convenient tool for asymmetric functionalization of natural products and drugs by facile introduction of chiral benzylic alkenylboration subunits. The preliminary mechanistic study indicates that a reversible β-H elimination is less likely to occur after the formation of a stable benzylic nickel species. Further mechanistic studies are currently in progress in our laboratory.
Data availability
All experimental data and detailed procedures are available in the ESI.†
Author contributions
Y. Y. and J. L. designed the research. Y. Y. performed the experiments, analyzed the data, and wrote the paper. B. X. and S. J. synthesized some of the vinyl halides. R. B., S. L., T. X. and X.-Y. Y. revised the paper.
Conflicts of interest
There are no conflicts to declare.
Acknowledgements
This work was supported by the National Natural Science Foundation of China (81730108, 81973635, and 82073686), Hangzhou Normal University startup fund (4125C5021920419), Hangzhou Normal University School of Medicine Teaching Reform Fund (4125b30100112), the Ministry of Science and Technology of China (High-end foreign experts program, G20200217005), Hangzhou's “115” plan to introduce overseas intelligence projects (20200215).
Notes and references
- For reviews on alkene difunctionalization:
(a) R. I. McDonald, G. Liua and S. S. Stahl, Chem. Rev., 2011, 111, 298 CrossRef PubMed;
(b) V. Saini, B. J. Stokes and M. S. Sigman, Angew. Chem., Int. Ed., 2013, 52, 11206 CrossRef CAS PubMed;
(c) G. Yin, X. Mu and G. Liu, Acc. Chem. Res., 2016, 49, 2413 CrossRef CAS PubMed;
(d) J. R. Coombs and J. P. Morken, Angew. Chem., Int. Ed., 2016, 55, 2636 CrossRef CAS PubMed;
(e) J. Derosa, V. T. Tran, V. A. Van der Puyl and K. M. Engle, Chem. Sci., 2018, 9, 5278 RSC;
(f) Y. Li, D. Wu, H.-G. Cheng and G. Yin, Angew. Chem., Int. Ed., 2020, 59, 7990 CrossRef CAS PubMed;
(g) X. Qi and T. Diao, ACS Catal., 2020, 10, 8542 CrossRef CAS PubMed;
(h) B. Tian, P. Chen, X. Leng and G. Liu, Nat. Catal., 2021, 2, 172 CrossRef.
-
(a) M. Suginome, Chem. Rec., 2010, 10, 348 CrossRef CAS PubMed;
(b) S. N. Mlynarski, C. H. Schuster and J. P. Morken, Nature, 2013, 505, 386 CrossRef PubMed;
(c) K. Semba, T. Fujihara, J. Terao and Y. Tsuji, Tetrahedron, 2015, 71, 2183 CrossRef CAS;
(d) E. C. Neeve, S. J. Geier, I. A. I. Mkhalid, S. A. Westcott and T. B. Marder, Chem. Rev., 2016, 116, 9091 CrossRef CAS PubMed.
-
(a) F.-S. Han, Chem. Soc. Rev., 2013, 42, 5270 RSC;
(b) D. Leonori and V. K. Aggarwal, Acc. Chem. Res., 2014, 47, 3174 CrossRef CAS PubMed;
(c) D. Leonori and V. K. Aggarwal, Angew. Chem., Int. Ed., 2015, 54, 1082 CrossRef CAS PubMed;
(d) C. Sandford and V. K. Aggarwal, Chem. Commun., 2017, 53, 5481 RSC;
(e) B. S. L. Collins, C. M. Wilson, E. L. Myers and V. K. Aggarwal, Angew. Chem., Int. Ed., 2017, 56, 11700 CrossRef CAS PubMed;
(f) H. Wang, C. Jing, A. Noble and V. K. Aggarwal, Angew. Chem., Int. Ed., 2020, 59, 16859 CrossRef CAS PubMed.
-
(a) H. Ito, Y. Kosaka, K. Nonoyama, Y. Sasaki and M. Sawamura, Angew. Chem., Int. Ed., 2008, 47, 7424 CrossRef CAS PubMed;
(b) H. Y. Cho and J. P. Morken, J. Am. Chem. Soc., 2008, 130, 16140 CrossRef CAS PubMed;
(c) H. Ito, T. Toyoda and M. Sawamura, J. Am. Chem. Soc., 2010, 132, 5990 CrossRef CAS PubMed;
(d) C. Zhong, S. Kunii, Y. Kosaka, M. Sawamura and H. Ito, J. Am. Chem. Soc., 2010, 132, 11440 CrossRef CAS PubMed;
(e) W. Su, T.-J. Gong, X. Lu, M.-Y. Xu, C.-G. Yu, Z.-Y. Xu, H.-Z. Yu, B. Xiao, Fu and Y. Angew, Chem., Int. Ed., 2015, 54, 12957 CrossRef CAS PubMed;
(f) T. W. Butcher, E. J. McClain, T. G. Hamilton, T. M. Perrone, K. M. Kroner, G. C. Donohoe, N. G. Akhmedov, J. L. Petersen and B. V. Popp, Org. Lett., 2016, 18, 6428 CrossRef CAS PubMed;
(g) J. C. Green, M. V. Joannou, S. A. Murray, J. M. Zanghi and S. J. Meek, ACS Catal., 2017, 7, 4441 CrossRef CAS PubMed.
-
(a) D. Hemming, R. Fritzemeier, S. A. Westcott, W. L. Santos and P. G. Steel, Chem. Soc. Rev., 2018, 47, 7477 RSC;
(b) S. Z. Sun, L. Talavera, P. Spiess, C. Day and R. Martin, Angew. Chem., Int. Ed., 2021, 60, 11740 CrossRef CAS PubMed;
(c) X. Yu, H. Zheng, H. Zhao, B. C. Lee and M. J. Koh, Angew. Chem., Int. Ed., 2021, 60, 2104 CrossRef CAS PubMed.
- For reviews on dual metal catalysis:
(a) D. R. Pye and N. P. Mankad, Chem. Sci., 2017, 8, 1705 RSC;
(b) K. Semba and Y. Nakao, J. Synth. Org. Chem. Jpn., 2017, 75, 1133 CrossRef CAS;
(c) K. Johnson and D. J. Weix, Science, 2019, 363, 819 CrossRef CAS PubMed.
- For reviews on Cu/Pd-catalysis:
(a) K. Semba and Y. Nakao, J. Am. Chem. Soc., 2014, 136, 7567 CrossRef CAS PubMed;
(b) K. B. Smith, K. M. Logan, W. You and M. K. Brown, Chem.–Eur. J., 2014, 20, 12032 CrossRef CAS PubMed;
(c) K. M. Logan, K. B. Smith and M. K. Brown, Angew. Chem., Int. Ed., 2015, 54, 5228 CrossRef CAS PubMed;
(d) K. M. Logan and M. K. Brown, Angew. Chem., Int. Ed., 2017, 56, 851 CrossRef CAS PubMed;
(e) B. Chen, P. Cao, X. Yin, Y. Liao, L. Jiang, J. Ye, M. Wang and J. Liao, ACS Catal., 2017, 7, 2425 CrossRef CAS;
(f) Y. Huang and K. M. Brown, Angew. Chem., Int. Ed., 2019, 58, 6048 CrossRef CAS PubMed;
(g) A. Bergmann, S. Dorn, K. Smith, K. Logan and M. K. Brown, Angew. Chem., Int. Ed., 2019, 58, 1719 CrossRef CAS PubMed.
- K. Semba, Y. Ohtagaki and Y. Nakao, Org. Lett., 2016, 18, 3956 CrossRef CAS PubMed.
-
(a) K. Yang and Q. Song, Org. Lett., 2016, 18, 5460 CrossRef CAS PubMed;
(b) K. Yang and Q. Song, J. Org. Chem., 2016, 81, 1000 CrossRef CAS PubMed;
(c) H. Pang, D. Wu, H. Cong and G. Yin, ACS Catal., 2019, 9, 8555 CrossRef CAS.
-
(a) K. B. Smith, Y. Huang and M. K. Brown, Angew. Chem., Int. Ed., 2018, 57, 6146 CrossRef CAS PubMed;
(b) W. Zhuang, P. Chen and G. Liu, Chin. J. Chem., 2021, 39, 50 CrossRef CAS.
- For reviews on nickel catalysis:
(a) S. Z. Tasker, E. A. Standley and T. F. Jamison, Nature, 2014, 509, 299 CrossRef CAS PubMed;
(b) V. P. Ananikov, ACS Catal., 2015, 5, 1964 CrossRef CAS.
-
(a) P. Gao, L.-A. Chen and M. K. Brown, J. Am. Chem. Soc., 2018, 140, 10653 CrossRef CAS PubMed;
(b) K. M. Logan, S. R. Sardini, S. D. White and M. K. Brown, J. Am. Chem. Soc., 2018, 140, 159 CrossRef CAS PubMed;
(c) S. R. Sardini, A. L. Lambright, G. L. Trammel, H. H. Omer, P. Liu and M. K. Brown, J. Am. Chem. Soc., 2019, 141, 9391 CrossRef PubMed;
(d) L.-A. Chen, A. Lear, P. Gao and M. K. Brown, Angew. Chem., Int. Ed., 2019, 58, 10956 CrossRef CAS PubMed;
(e) S. Joung, A. M. Bergmann and M. K. Brown, Chem. Sci., 2019, 10, 10944 RSC;
(f) A. K. Simlandy, S. Sardini and M. K. Brown, Chem. Sci., 2021, 12, 5517 RSC.
-
(a) W. Wang, C. Ding, Y. Li, Z. Li, Y. Li, L. Peng and G. Yin, Angew. Chem., Int. Ed., 2019, 58, 4612 CrossRef CAS PubMed;
(b) W. Wang, D. Chao, H. Pang, Z. Li, Y. Li, L. Peng and G. Yin, Org. Lett., 2019, 21, 3968 CrossRef CAS PubMed;
(c) Y. Li, H. Pang, D. Wu, Z. Li, W. Wang, H. Wei, Y. Fu and G. Yin, Angew. Chem., Int. Ed., 2019, 58, 8872 CrossRef CAS PubMed;
(d) Y. Li, H. Wei, D. Wu, Z. Li, W. Wang and G. Yin, ACS Catal., 2020, 10, 4888 CrossRef CAS;
(e) W. Wang, C. Ding and G. Yin, Nat. Catal., 2020, 3, 951 CrossRef CAS.
-
(a) J. Liu, Q. Ren, X. Zhang and H. Gong, Angew. Chem., Int. Ed., 2016, 55, 15544 CrossRef CAS PubMed;
(b) Y. Ye, H. Chen, J. L. Sessler and H. Gong, J. Am. Chem. Soc., 2019, 141, 820 CrossRef CAS PubMed;
(c) K. E. Poremba, S. E. Dibrell and S. E. Reisman, ACS Catal., 2020, 10, 8237 CrossRef CAS PubMed;
(d) J. Liu, Y. Ye, J. L. Sessler and H. Gong, Acc. Chem. Res., 2020, 53, 1833 CrossRef CAS PubMed;
(e) W. Xue, X. Jia, X. Wang, X. Tao, Z. Yin and H. Gong, Chem. Soc. Rev., 2021, 50, 4162 RSC.
-
(a) A. H. Cherney and S. E. Reisman, J. Am. Chem. Soc., 2014, 136, 14365 CrossRef CAS PubMed;
(b) N. Suzuki, J. L. Hofstra, K. E. Poremba and S. E. Reisman, Org. Lett., 2017, 19, 2150 CrossRef CAS PubMed;
(c) A. M. Olivares and D. J. Weix, J. Am. Chem. Soc., 2018, 140, 2446 CrossRef CAS PubMed;
(d) X. Wang, G. Ma, Y. Peng, C. E. Pitsch, B. J. Moll, T. D. Ly, X. Wang and H. Gong, J. Am. Chem. Soc., 2018, 140, 14490 CrossRef CAS PubMed;
(e) Y. Ye, H. Chen, K. Yao and H. Gong, Org. Lett., 2020, 22, 2070 CrossRef CAS PubMed;
(f) P. Zhang, C. Zou, Q. Zhao and C. Zhang, Org. Chem. Front., 2021, 8, 2589 RSC.
- For a mechanistically distinct Ni-catalyzed intramolecular carboboration of alkynes, see:
(a) A. Yamamoto and M. Suginome, J. Am. Chem. Soc., 2005, 127, 15706 CrossRef CAS PubMed;
(b) M. Daini, A. Yamamoto and M. Suginome, Asian J. Org. Chem., 2013, 2, 968 CrossRef CAS.
- For recent examples of Ni-catalyzed hydroarylation and hydroalkylation, see:
(a) X. Lu, B. Xiao, Z. Zhang, T. Gong, W. Su, J. Yi, Y. Fu and L. Liu, Nat. Commun., 2016, 7, 11129 CrossRef PubMed;
(b) S. A. Green, J. L. M. Matos, A. Yagi and R. A. Shenvi, J. Am. Chem. Soc., 2016, 138, 12779 CrossRef CAS PubMed;
(c) Y. He, Y. Cai and S. Zhu, J. Am. Chem. Soc., 2017, 139, 1061 CrossRef CAS PubMed;
(d) J. Liu, H. Gong and S. Zhu, Angew.
Chem., Int. Ed., 2021, 60, 4060 CrossRef CAS PubMed;
(e) Y. He, H. Song and S. Zhu, Nat. Commun., 2021, 12, 638 CrossRef CAS PubMed;
(f) D. Qian, S. Bera and X. Hu, J. Am. Chem. Soc., 2021, 143, 1959 CrossRef CAS PubMed;
(g) S. Bera, R. Mao and X. Hu, Nat. Chem., 2021, 13, 270 CrossRef CAS PubMed;
(h) S. Wang, J.-X. Zhang, T.-Y. Zhang, H. Meng, B.-H. Chen and W. Shu, Nat. Commun., 2021, 12, 2771 CrossRef CAS PubMed;
(i) J.-W. Wang, Y. Li, W. Nie, Z. Chang, Z.-A. Yu, Y.-F. Zhao, X. Lu and Y. Fu, Nat. Commun., 2021, 12, 1313 CrossRef CAS PubMed;
(j) S. Cuesta-Galisteo, J. Schörgenhumer, X. Wei, E. Merino and C. Nevado, Angew. Chem., Int. Ed., 2021, 60, 1605 CrossRef CAS PubMed.
- For recent examples of dicarbofunctionalization of alkenes by Ni-catalysis, see:
(a) A. García-Domínguez, Z. Li and C. Nevado, J. Am. Chem. Soc., 2017, 139, 6835 CrossRef PubMed;
(b) B. Shrestha, P. Basnet, R. K. Dhungana, S. KC, S. Thapa, J. M. Sears and R. Giri, J. Am. Chem. Soc., 2017, 139, 10653 CrossRef CAS PubMed;
(c) J. Derosa, V. T. Tran, M. N. Boulous, J. S. Chen and K. M. Engle, J. Am. Chem. Soc., 2017, 139, 10657 CrossRef CAS PubMed;
(d) W. Shu, A. García-Domínguez, M. T. Quirós, R. Mondal, D. J. Cárdenas and C. Nevado, J. Am. Chem. Soc., 2019, 141, 13812 CrossRef CAS PubMed;
(e) X. Wei, W. Shu, A. García-Domínguez, E. Merino and C. Nevado, J. Am. Chem. Soc., 2020, 142, 13515 CrossRef CAS PubMed;
(f) H.-Y. Tu, F. Wang, L. Huo, Y. Li, S. Zhu, X. Zhao, H. Li, F.-L. Qing and L. Chu, J. Am. Chem. Soc., 2020, 142, 9604 CAS.
- For representative examples of Ni-catalyzed reactions involving diboron reagents, see:
(a) K. Hirano, H. Yorimitsu and K. Oshima, Org. Lett., 2007, 9, 5031 CrossRef CAS PubMed;
(b) Y. Sumida, H. Yorimitsu and K. Oshima, Org. Lett., 2008, 10, 4677 CrossRef CAS PubMed;
(c) A. S. Dudnik and G. C. Fu, J. Am. Chem. Soc., 2012, 134, 10693 CrossRef CAS PubMed.
- For β-substituted styrenes, no desired product was obtained, and the major by-products were the reduction of the corresponding vinylarene for the vinylarenes with moderate yields.
- B.-L. Lin, L. Liu, Y. Fu, S.-W. Luo, Q. Chen and Q.-X. Guo, Organometallics, 2004, 23, 2114 CrossRef CAS.
- It is similar to the dual metal catalysis reaction, please see ref. 6 and 7.
-
(a) L. S. Hegedus and D. H. P. Thompson, J. Am. Chem. Soc., 1985, 107, 5663 CrossRef CAS;
(b) R. Shrestha, S. C. M. Dorn and D. J. Weix, J. Am. Chem. Soc., 2013, 135, 751 CrossRef CAS PubMed;
(c) S. Biswas and D. J. Weix, J. Am. Chem. Soc., 2013, 135, 16192 CrossRef CAS PubMed;
(d) D. J. Weix, Acc. Chem. Res., 2015, 48, 1767 CrossRef CAS PubMed.
Footnotes |
† Electronic supplementary information (ESI) available. See DOI: 10.1039/d1sc04071e |
‡ These authors contributed equally. |
|
This journal is © The Royal Society of Chemistry 2021 |
Click here to see how this site uses Cookies. View our privacy policy here.