DOI:
10.1039/D2CB00033D
(Review Article)
RSC Chem. Biol., 2022,
3, 495-518
Non-invasive radionuclide imaging of trace metal trafficking in health and disease: “PET metallomics”
Received
1st February 2022
, Accepted 7th April 2022
First published on 11th April 2022
Abstract
Several specific metallic elements must be present in the human body to maintain health and function. Maintaining the correct quantity (from trace to bulk) and location at the cell and tissue level is essential. The study of the biological role of metals has become known as metallomics. While quantities of metals in cells and tissues can be readily measured in biopsy and autopsy samples by destructive analytical techniques, their trafficking and its role in health and disease are poorly understood. Molecular imaging with radionuclides – positron emission tomography (PET) and single photon emission computed tomography (SPECT) – is emerging as a means to non-invasively study the acute trafficking of essential metals between organs, non-invasively and in real time, in health and disease. PET scanners are increasingly widely available in hospitals, and methods for producing radionuclides of some of the key essential metals are developing fast. This review summarises recent developments in radionuclide imaging technology that permit such investigations, describes the radiological and physicochemical properties of key radioisotopes of essential trace metals and useful analogues, and introduces current and potential future applications in preclinical and clinical investigations to study the biology of essential trace metals in health and disease.
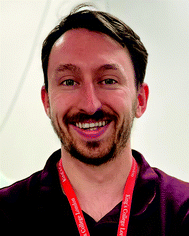
George Firth
| George Firth studied biomedical science at the University of Hull, where he completed a BSc and MSc. With his supervisor Dr Graeme J. Stasiuk, he designed novel zinc sensing probes for prostate cancer. He has since completed an MRes and PhD in medical imaging at King's College London under Prof Philip J. Blower, developing skills in radiochemistry, metallomics and molecular imaging. He is currently a post-doctoral researcher at KCL, applying positron emission tomography (PET) imaging of radioactive essential metals (52Mn, 64Cu, 62Zn) to non-invasively study metal trafficking in vivo in health and disease. |

Julia E. Blower
| Julia Blower completed her PhD at King's College London under Prof Tony Gee, focusing on developing novel 13N and 11C radiochemistry. She gained experience at St Thomas’ Hospital Clinical PET Centre, implementing carbon-11 PET tracers for clinical use. In 2015, Julia took up a post-doctoral position at Memorial Sloan Kettering Cancer Center, New York with Prof Jason Lewis, gaining experience in bioconjugate labelling (68Ga, 64Cu, 18F, 131I) and biological evaluation techniques. Julia is now a Research Fellow in Molecular Imaging within the Centre for Medical Engineering, KCL, responsible for facilitating development of new radiotracers through preclinical stages, towards clinical translation. |
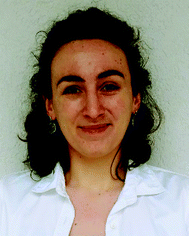
Joanna J. Bartnicka
| Joanna completed her PhD in Molecular Imaging in 2021 at the School Of Biomedical Engineering and Imaging Sciences, King's College London, under the supervision of Prof Phil Blower. She dedicated her doctoral studies to developing methodologies for PET metallomics, with a focus on copper trafficking in cancer. After completing her studies Joanna took up the role of a scientific project officer at the Joint Research Centre of the European Commission, where she is involved in the investigation of the environmental epidemiology of cancer, with a focus on population exposure to carcinogenic metals. |
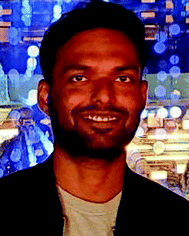
Aishwarya Mishra
| Aishwarya Mishra graduated from University of Mumbai in chemistry. Presently, he is pursuing a PhD at CDT in Medical Imaging based at King's College London and Imperial College London. His interests include chemical synthesis, nanomedicines, drug delivery, gallium radiochemistry and preclinical validation. He has a keen interest in public and school engagement and has helped organise major science festivals including the Great Exhibition Road Festival. He has held key team positions in the European Society of Molecular Imaging (ESMI) and Controlled Release Society (CRS). |
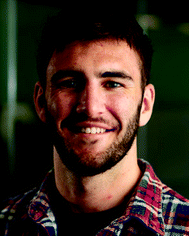
Aidan M. Michaels
| Aidan received his BSc (Hons) in Medical Biochemistry from the University of Leicester in 2018. He is currently a final year PhD student in medical imaging at King's College London, developing a combination of in vivo imaging techniques employing 23Na MRI for probing intracellular and extracellular sodium ion concentration and 82Rb PET for probing Na/K ATPase activity in tumours and in the heart to offer a hitherto unexplored window on dysregulated metabolism in vivo. He is also the host of the RadioNuclear Podcast, making medically-driven research breakthroughs more accessible to the general public. |
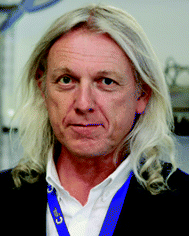
Philip J. Blower
| Since 2006 Phil Blower has been Head of the Imaging Chemistry and Biology Department at King's College London in the Division of Imaging Sciences and Biomedical Engineering. His research interests are best summarised as “molecular imaging” mainly using inorganic chemistry tools. He has built a large interdisciplinary research group with wide interests covering radiopharmaceutical chemistry and biology for PET, SPECT and radionuclide therapy, applying inorganic chemistry from all parts of the periodic table to nuclear medicine. He has published >200 peer-reviewed papers and supervised >40 successful PhD students. |
Introduction
Trace metals, such as iron, copper, zinc and manganese, are essential for the normal function of living organisms. In health, the absorption, storage, utilisation and excretion of these important metals are under strict control; deficiency or overload adversely affects health. Dysregulation of normal metal homeostasis has been implicated in a number of diseases, including cancer, neurological disorders such as Alzheimer's disease and inflammatory conditions such as rheumatoid arthritis (Fig. 1A), and is receiving heightened interest among biomedical researchers. A diverse range of tools and analytical techniques is available to study the biological behaviour of metals, each with its own strengths and weaknesses. The concentration of trace metals in tissues can be investigated by analytical methods such as inductively coupled-plasma mass spectrometry (ICP-MS), synchrotron based methods such as X-ray fluorescence microscopy, and fluorescent metal-binding probes. These are powerful tools to study metal distribution in tissues and cells and some have been developed into cell-level imaging techniques to determine the cellular, and even sub-cellular, location of trace metals within tissues. For example, mass spectrometric detection methods such as laser ablation (LA) ICP-MS and secondary ion mass spectrometry (SIMS) can provide 2-dimensional maps of trace metal distribution in tissue sections.1 However, the majority of these techniques require ex vivo tissue samples from autopsy or biopsy and hence are invasive, and the analytical method destructive. They provide a snapshot of concentration and location in time, and each sample is analysed at only one time point. This perspective is necessary to examine the static consequence of chronic and prolonged accumulation of metals in cells and tissues, but not sufficient because it provides no information on the dynamic processes that led to it. In order to provide a perspective on the acute, dynamic handling of trace metals in vivo, at the whole body or organ level, non-invasive and non-destructive techniques are needed.
 |
| Fig. 1 Radioactive metals allow for the non-invasive study of essential trace metals in vivo in health and disease. (A) A summary of diseases implicated in metal homeostasis dysregulation that can be studied using radionuclide imaging. (B) Example of a total-body [18F]FDG PET scan highlighting glucose avid tissues.2 (C) Periodic table colour-coded to highlight elements with useful radioisotopes that can be used to image trace metal biology. Radionuclides with metallomics applications are discussed in individual sections throughout this review. | |
Molecular imaging methods employed clinically in the field of nuclear medicine offer a solution. Nuclear medicine uses picomolar quantities of radioactive substances to study biological processes by three-dimensional, non-invasive dynamic imaging of their distribution in the whole body, exploiting the tissue-penetrating power of gamma radiation. Positron emission tomography (PET) and single photon emission computed tomography (SPECT) are radionuclide imaging techniques that provide three-dimensional information on the spatial and temporal biodistribution of radioactivity in the body. In the clinic, the PET radiotracer fluorodeoxyglucose ([18F]FDG) containing the positron-emitting radionuclide 18F accounts for over 90% of all scans and remains the standard for the diagnosis of cancer by providing information on glucose metabolism (Fig. 1B). 99mTc, a gamma emitter, is the most commonly used medical radioisotope, accounting for over 90% of SPECT scans and the vast majority of all nuclear medicine scans. A diverse range of 99mTc- and 18F-labelled radiopharmaceuticals are employed to study a broad range of biophysical processes, including imaging renal and hepatic function, bone metastases, cardiac perfusion and function, pulmonary ventilation and perfusion. In recent years, emphasis in nuclear medicine has shifted towards development of radiotracers that target specific molecular processes using radiolabelled metabolites, receptor-binding peptides or tumour-targeted antibodies. Often the radiolabels used in these biomolecular radiopharmaceuticals are metals (99mTc, 64Cu, 68Ga, 89Zr etc.). Well-established examples include 89Zr-DFO-trastuzumab and 68Ga-PSMA.3–5 The principle of using radioactive isotopes of metals for routine diagnostic medicine and for research, in humans and in animal models, is thus well-established. The use of radiometals to study the trafficking and metabolism of the metals themselves, by PET and SPECT imaging, is less well-established because the radiometals most convenient and available to use in nuclear medicine do not match the list of biologically essential metals. Recently however, recognition of the value of using radiometals to image the biology of the metal has grown, and methods to produce useful, imageable radioisotopes of the biologically important metals (e.g. manganese, iron, copper and zinc) are being developed. Thus, ‘PET metallomics’ is an emerging paradigm that uses positron- (and gamma-) emitting radiometals to non-invasively study metal trafficking in vivo, both in animals and humans.6 Though there are other imaging modalities that can provide information on metal trafficking non-invasively, this review is focused specifically on PET and SPECT radiometals. Magnetic resonance imaging (MRI) contrast agents have been discussed where relevant, but are not the main focus of this review.
This review will provide a timely summary of PET and SPECT radiometals that can be implemented to study essential trace metals dynamically and non-invasively across the whole-body, to develop understanding of the links between aberrant metal trafficking and disease, and, potentially, to diagnose disease in patients and monitor the effects of treatment. Established and emerging radionuclides that can image endogenous metals (Fig. 1C), classified by periodic group, will be discussed, including recent developments in radionuclide production and imaging technology that will help pave the way for this emerging field. Questions that can be addressed by such methods, and potential clinical applications, will be discussed.
Group 1 (Na, K, Rb)
Alkali metals have critical roles in biology. Together, sodium and potassium are responsible for establishing the transmembrane electrochemical gradient in most cells, and facilitate the generation and transmission of action potentials along neurons and into tissues.7 Sodium and potassium exist in the ionic +1 oxidation state (Na+ and K+). Na+ is the primary cation of extracellular fluid, with a typical healthy concentration of 135–145 mM.8 K+ is the most prevalent cytosolic cation in eukaryotic organisms. In humans, 98% of K+ is intracellular with an average intracellular concentration of 140–150 mM in healthy cells.9 The concentration gradient across the plasma membrane (high intracellular potassium, low extracellular potassium; low intracellular sodium, high extracellular sodium) and the accompanying electrical potential gradient (cytosol negative relative to extracellular space) is driven and maintained mainly by the Na+, K+-adenosine triphosphatase (Na+/K+-ATPase). This active, ATP-consuming membrane pump is ubiquitously expressed in the body, but the rate of enzymatic activity and thus the flux of Na+ out and K+ in, in a ratio of 3
:
2, across the plasma membrane varies in different tissues and organs.10 The heart has a greater uptake of K+ and other cationic radiotracers compared to other healthy tissues, and this underpins the uptake mechanism of some cardiovascular perfusion imaging agents (see below).
Due to the ubiquitous and essential function of Na+ and K+, radionuclides of sodium and potassium have been of interest to those investigating the biology of these ions in vivo. Several radioisotopes of sodium are available, but few have radiological properties that support imaging. 22Na (β+, t1/2 = 2.6 years) has been used to image sodium transport in plants,11–13 but has seen limited use in humans due to its long half-life which results in high radiation absorbed dose to subjects receiving it. 24Na (β−, t1/2 = 15 hours) has a shorter half-life that is more suited to studying sodium trafficking, but its poorly-penetrating beta emission limits its use to in vitro measurements with a beta detector. Many of the radioisotopes of potassium are likewise unsuitable for modern imaging techniques given their β− decay. The short half-life of 38K (t1/2 = 7.6 min) and its requirement for an on-site cyclotron producing a high-energy (ca. 30 MeV) proton beam prevent widespread availability. It was, however, used for imaging studies in pre-clinical models at the dawn of PET imaging15,16 and in-human investigations were performed to evaluate its utility as a perfusion imaging agent, exploiting its high and rapid accumulation in myocytes via the Na+/K+-ATPase.15,17 The conclusions drawn from these studies were that 38K accumulates readily in cardiac tissue and has lower uptake in the liver compared to other myocardial perfusion imaging agents such as [62Cu]Cu–PTSM (an established perfusion imaging agent at the time of investigation).17 Advances in technology supporting PET imaging may warrant revisiting this radionuclide for its classical use of cardiovascular imaging or for studies of changes to the in vivo biodistribution of K+ in different disease states. However, its short half-life and cyclotron production will hinder widespread adoption.
The poor availability of sodium and potassium imaging radionuclides can be partially mitigated by use of surrogate radioisotopes of chemically analogous metals. 82Rb and 201Tl fulfil this role for potassium. 82Rb (β+, t1/2 = 76 s) is the only group 1 radionuclide that has seen significant use in nuclear medicine. The key to its widespread use is its production via the 82Sr/82Rb generator, which has a shelf-life of ∼1 month. 82Rb formed by decay of 82Sr can be eluted as [82Rb]RbCl with physiological saline directly into a patient via intravenous (i.v.) infusion – a necessity as the rapid decay does not permit manipulations with syringes or radiochemistry. The short half-life of 82Rb allows repeated dosing and imaging studies to be performed, for example, preceding and following a therapeutic intervention, while keeping the radiation dose to the patient low. Consistent with its periodic location immediately below potassium, and despite a slightly larger ionic radius, Rb+ is able to enter cells via the Na+/K+-ATPase, one of many transporters responsible for potassium influx, and therefore has potential applications in the field of metallomics as a K+ mimic, as well as myocardial perfusion imaging. The in vivo pharmacokinetics and pharmacodynamics of 82Rb have made it a routine option for imaging myocardial perfusion, with initial 82Rb uptake in metabolically active tissue proportional to blood flow. This results in comparable quality images to the traditional radiotracers used for SPECT imaging (Fig. 2A).14,18
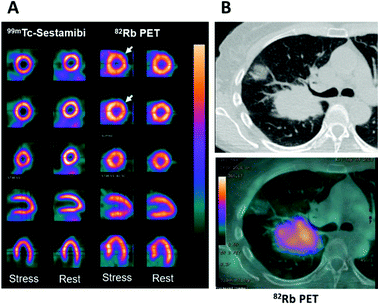 |
| Fig. 2 Applications of potassium mimetics in nuclear medicine. (A) Representative myocardial perfusion imaging with 99mTc-Sestamibi SPECT and 82Rb-PET.14 From top to bottom: axial apical, mid and basal sections, coronal and sagittal slices. (B) Clinical CT scan of a lung cancer patient (top) with a corresponding axial section from a 82Rb PET scan (bottom) (courtesy of A. Groves, UCL). Myocardial perfusion images are adapted and reproduced with permission under a Creative Commons Attribution (CC-BY) License from ref. 14, Front. Med., copyright© 2015. | |
The utilisation of 82Rb has recently expanded beyond myocardial perfusion imaging into the field of clinical oncology where it has been used to image tumour perfusion (Fig. 2B). Critically, the inferences drawn from these investigations require further probing to distinguish tumour cell uptake of 82Rb compared to accumulation in the tumoural interstitial fluid. Notably, the first in-human investigations have indicated that prognostic information can be gathered from 82Rb imaging of tumours. Jochumsen et al. demonstrated increased uptake in higher grade prostate cancer compared to lower grade.19 Like all blood–borne radiotracers, the tissue accumulation is governed both by perfusion and the abundance of the specific molecular target or binding mechanism. Which of these contributors dominates, and hence whether or not 82Rb imaging can quantify Na+/K+-ATPase activity (either in cardiac or tumour imaging), remains to be fully determined. In the aforementioned prostate cancer study, 82Rb uptake did not correlate with cell or Na+/K+-ATPase optical density; on the other hand other studies suggest that 82Rb is not a pure perfusion tracer either.20 These findings warrant further investigation, particularly whether Na+/K+-ATPase activity is the driving mechanism for uptake and whether tissue uptake reflects Na+/K+-ATPase activity or merely perfusion. Repurposing 82Sr/82Rb generators for oncological imaging may be a future trend in clinical oncology offering a complementary imaging method to 18F-FDG. The short half-life and rapid clearance could enable greater prognostic information with minimal increase to the scan time and radiation absorbed dose to patients, helping to stratify patients more effectively.
The short half-life of 82Rb is advantageous as it permits multiplexed imaging (i.e. imaging several radiotracer distributions rather than just one, which may become possible using Total Body PET2) with other radioactive metals to map their trafficking in health and disease. However, it also restricts imaging to very early time points, where perfusion may be the main determinant of uptake, rather than the molecular trapping mechanism and thus negating any molecular information value. SPECT imaging with the longer half-life 201Tl may offer a solution to this problem. Although not endogenous to the human body, and toxic in large quantities, thallium in the ionic +1 oxidation state (Tl+) can be used as a surrogate marker of K+. Due to the similarity of its ionic radius (150 pm) to that of K+ (138 pm), it has similar affinity for potassium channels such as the Na+/K+-ATPase. Thus, cyclotron-produced 201Tl (γ, t1/2 = 3.04 days, 69–81 keV X-rays), intravenously administered in nano- to picomolar quantities as thallous chloride ([201Tl]TlCl), has historically been used in the clinic to image myocardial perfusion with gamma scintigraphy and SPECT.21201Tl is rapidly cleared from the blood and localises primarily to the heart and skeletal muscle with clearance through the kidneys.22 Poor delivery from impaired blood flow (ischaemia) or damaged tissue gives rise to ‘cold spots’, and thus provides clinically relevant information that is used in the visualisation and diagnosis of myocardial infarction. 201Tl imaging can in principle provide information on Na+/K+-ATPase activity but this remains to be validated in vivo and clinically.
The utility of 201Tl is not limited to just myocardial imaging; clinical investigations of glioblastomas,23 lymphoma, breast and lung cancers have also been performed.24 These studies, largely from the 1980's and 1990's, demonstrated use in distinguishing benign from malignant lesions and evaluating chemotherapy response and tumour malignancy. Additional studies in the context of imaging potassium biology in cancer are required, especially given that the Na+/K+ ATPase could be a therapeutic target for cancer treatment.
Although 201Tl has played an important historical role in the development of nuclear medicine, it is much less widely used now than in the past, and has largely been replaced as a myocardial perfusion imaging agent by 99mTc-labelled tracers, which accumulate in myocardium by mechanisms unrelated to Na+/K+ ATPase activity.18 The disadvantages of 201Tl are largely associated with its emission properties. The low energy of the photon emissions causes high soft tissue attenuation and thus poor image quality. In addition, the long half-life, while offering the advantage of international transport and late time-point imaging, also imparts high radiation dose to subjects; likewise the unusually high number (ca. 37 per decay) of Auger electrons emitted as part of the decay process add substantially to the radiation dose absorbed by the cell in which the radionuclide resides, inducing significant cellular toxicity.25,26 Nevertheless, some institutes still rely on 201Tl for imaging myocardial viability for its high specificity and sensitivity for coronary artery disease,27 and there is renewed interest in the radiobiology of 201Tl because the aforementioned Auger electron emissions may offer radionuclide therapy applications.28,29
Group 2 (Mg, Ca, Sr, Ra)
Like group 1, group 2 lacks radioisotopes of essential metals that are suitable for imaging. The majority find applications as surrogates for imaging calcium. The beta emitter 89Sr (β−, 100%, t1/2 = 53 days), administered as strontium chloride, has been used for many years as a palliative therapy for painful bone metastases in cancer. Accumulation of strontium in sites of active bone mineral deposition (e.g. bone metastases) is thought to be driven by calcium mimicry. However, 89Sr lacks positron and gamma emissions and so cannot be imaged. The gamma emissions of 85Sr (γ, 100%, t1/2 = 65 days) can in principle be used to determine its quantitative biodistribution but the long half-life limits clinical applications. 223Ra (α, 94%, t1/2 = 11.4 days), used similarly in the form of radium chloride, also accumulates in sites of bone mineralisation and is a potent therapeutic in bone metastases.30 Although it has some imageable gammas (<2% abundance), a substantial radiation dose from its alpha emissions make it an unrealistic tool for imaging calcium flux. Manganese and cobalt have also been reported to display calcium mimetic behaviour,31–33 but given that these metals are endogenous they have their own regulatory pathways which in some cases may be shared with other metals, making their use for the study of calcium trafficking unrealistic (both of these metals, and the evidence for their similar behaviour to calcium, are discussed in respective sections below).
Positron emitting radioisotopes of magnesium have half-lives <10 seconds and so cannot be practically imaged.
Group 3–5 (Ti, V, Sc)
Groups 3 through 5, although containing key radionuclides used within nuclear medicine such as 89Zr (long half-life positron emitter used as an antibody3,34 and cell35 radiolabel) and 90Y (high energy beta emitter used in radionuclide therapy), almost entirely lack biologically relevant metals. Vanadium is essential for some organisms,36 but is not currently recognised as an essential metal in humans. Interest in vanadium lies in its application in vanadium-based drugs, such as complexes of the vanadate (H2VO4−) anion or the vanadyl (VO2+) cation. For example, orally administered vanadium complexes have shown efficacy in the treatment of hyperglycaemia in diabetes due to their insulinomimetic behaviour,37 but mechanisms remain poorly characterised. Gastrointestinal absorption rate and tissue distribution of these complexes could be addressed with radiovanadium PET. Positron emitting radioisotopes of vanadium – 47V (β+, t1/2 = 33 min) and 48V (β+, 50%, t1/2 = 16 days) – are available and could be used for these purposes, but have seen little use so far.
The generator-produced 44Sc (β+, 94%, t1/2 = 3.97 h) and its therapeutic counterpart 47Sc (β−, 100%, t1/2 = 3.35 days) have attracted interest in nuclear medicine as a theranostic pair (an imaging radionuclide and a therapeutic radioisotope of the same element),38 though in the context of this review scandium lacks biological significance. Titanium also has a useful radioisotope for PET imaging – 45Ti (β+, 85%, t1/2 = 3.08 h),38 but has little application in PET metallomics except perhaps in relation to imaging titanium toxicity from titanium implants.39
Group 6 (Cr, Mo, W)
Chromium in its trivalent oxidation state is found in most foods and nutrient supplements. The highest levels in human tissues are found in the kidney and liver.40 Whether chromium is an essential element in humans remains a topic of debate.41 Several studies have shown that chromium supplementation has a positive impact on glycaemic control in diabetes, though conflicting data have also been reported.42 Hexavalent chromium is toxic and was shown to be a human carcinogen when inhaled.43 Homeostatic mechanisms that control chromium in vivo are unknown, but low-molecular-weight chromium-binding substance (LMWCr, also known as chromodulin) and transferrin are likely to play a role. 49Cr (β+, 93%, t1/2 = 42.3 min) has attractive emission properties for PET imaging of chromium biology, at least in animal models, though its radioactive daughter 49V (t1/2 = 329 days) limits its use. The gamma rays produced by 51Cr (γ, 9.9%, t1/2 = 27.8 days) are of too high energy, and its half-life is too long, for imaging applications. However, it has seen historical use in nuclear medicine for non-imaging tracer applications in haematology and nephrology.44
Molybdenum is found in the body in approximately ten-fold greater quantity compared to chromium (9.3 vs. 1.8 mg respectively in a 70 kg human) and similar quantity to manganese (12 mg).41 Molybdenum is essential for humans; sulfite oxidase and xanthine oxidase both require it for their function.45 Notable molybdenum radioisotopes include 90Mo (β+, 24.9%, t1/2 = 5.6 h), which is the parent isotope for niobium-90 (β+, 53%, t1/2 = 14.6 h), and 99Mo (β−, t1/2 = 66 h), widely known in nuclear medicine as the parent in the 99mTc generator. Neither of these molybdenum isotopes are well-suited for metallomics-based imaging applications.
To date, no essential role for tungsten in humans has been recognised, though some microbial enzymes that require it have been identified.45 Chronic environmental overexposure to tungsten, particularly of those working in metallurgy, may induce toxicity. Beta emitters such as 185W (β−, t1/2 = 75.1 days) and 187W (β−, t1/2 = 23.7 h) can be used for ex vivo radioactivity measurements of tungsten trafficking.46187W has low energy gamma emissions (72–134 keV)47 that may have SPECT potential.
Group 7 (Mn)
Manganese, predominantly found in its +2 oxidation state in biological environments, is an essential metal with key roles such as defence against reactive oxygen species (ROS), not only in enzymes such as manganese superoxide dismutase and catalase but also in non-enzymatic forms.48–51 It also has crucial roles in reproduction,52 bone growth,53 the urea cycle,54 immune function55 and blood glucose control.56 The proteins that work in unison to mediate manganese import, export and storage are poorly understood. Without a reliable fluorescent probe or medical imaging agent tailored to manganese, our understanding of manganese handling has not progressed as fast as that of copper and zinc.
Disruption of manganese homeostasis can lead to overload and toxicity, i.e. manganism, a parkinsonian-like movement disorder characterised by chronic overload of manganese in the basal ganglia of the brain.57–59 Mutations in manganese transporters such as SLC30A10 (ZnT10) and SLC39A14 (ZIP14) disrupt the normal regulation of manganese absorption in the gut and excretion from the liver respectively. This leads to an increased concentration of manganese in the blood and subsequent deposition of manganese in the brain.60 Elevated concentrations of Mn(II) are detectable by MRI because of its paramagnetism, forming the basis for the clinical gold standard technique for diagnosing manganism. Both Mn(II) and Fe(III) cause hypointensity on T2-weighted MRI scans; however, T1-weighted imaging allows differentiation between Mn(II) and Fe(III) as only Mn(II) leads to hyperintensity. Interestingly, similar MRI brain findings have been reported in smelting workers, who showed the same MRI signature characteristic of manganese overexposure despite lacking clinical symptoms of manganese intoxication.61–65 Other causes of manganism that present with similar MRI brain appearances include overexposure of workers in certain occupational/environmental contexts, such as mining and welding,66 prolonged periods of parenteral nutrition,67 chronic liver disease involving impaired hepatobiliary excretion of manganese,68 and, among drug addicts, intake of manganese-contaminated ephedrone.69,70
The late 90s saw the introduction of MRI with administered exogenous free Mn2+, later termed manganese-enhanced MRI (MEMRI), as a tool for peripheral neuron tracing,71 based on the characteristic ability of Mn2+ ions to move in an anterograde manner along neurons. The mechanism controlling this transport remains unknown, though evidence from mice deficient in L-type Ca2+ channel 1.2 (Cav1.2) suggests Ca mimicking may be involved.72 The use of MEMRI in neuroscience has been reviewed.73 The large quantities of manganese that must be administered for adequate MR contrast, which are greatly in excess of those found biologically, unavoidably perturb homeostasis of manganese and probably other trace metals, and may be toxic. MEMRI is unlikely, therefore, to provide a clinically useful or indeed realistic summary of normal manganese handling in vivo. Manganese-based complexes are of interest as a replacement to Gd-based contrast agents that have come under intense scrutiny over the years due to the prevalent off-target accumulation of toxic exogenous Gd3+. However, the design and application of manganese-based MRI contrast agents is beyond the scope of this review and has been covered by others.
Radionuclide imaging offers an alternative and complementary approach to MRI to investigate in vivo acute dynamic trafficking of manganese. Several positron emitting radioisotopes of manganese, with emissions and half-lives suitable for applications in PET imaging, have become available in recent years: 52mMn (21.1 min), 51Mn (46.2 min), and 52gMn (also referred to as 52Mn, 5.6 days). The long-lived 52Mn is suited to imaging biological processes with a relatively long half-life, including the labelling of cells,74 antibodies75 and nanoparticles,74 and has been hailed as an emerging option for immunoPET with potential advantage over the currently widely-used 89Zr, which has a slightly shorter half-life (3.3 d). The production of 52Mn has recently been optimised by Fonslet et al., and can now be performed at facilities with access to a modest energy medical cyclotron (16 MeV) via the 52Cr(p,n)52Mn reaction.76 Although several centres worldwide now produce 52Mn for research purposes, and owing to its long half-life, it can be shipped internationally, few biological studies of 52Mn PET have been published. They include studies that reported the biodistribution of 52Mn administered intravenously as manganese(II) chloride in mice, rats and non-human primates.33,77–80 Uptake was highest in pancreas, salivary glands and, at early time points, myocardium (Fig. 3). The significant uptake of manganese in the pancreas, seen in both PET and MRI studies, may be of particular interest for the non-invasive assessment of β-cell mass. Pharmacological modulation of 52Mn uptake was achieved in isolated islets and the global pancreas in vivo with voltage-dependant calcium channel (VDCC) inhibitors and stimulators.33 These studies, combined with reduced 52Mn uptake in streptozotocin (STZ)-induced diabetic mouse pancreata, provide evidence that manganese import is mediated by VDCCs and can be used to estimate β-cell mass. STZ treatment has been shown to impact pancreatic vasculature raising the possibility that decreased perfusion, rather than changes in β-cell mass and function, may underlie reduction in manganese uptake.81 What is perhaps overlooked is the assumption that radiomanganese and paramagnetic manganese localise primarily to the endocrine component of the pancreas compared to the exocrine – a necessity for determining β-cell mass and function. A recent PET/MRI approach to assess β-cell mass and function was carried out using a radiolabelled GLP-1R antagonist, [64Cu]Cu-NODAGA-40Lys-Exendin-4, and paramagnetic manganese as MnCl2, which was then correlated with autoradiography and LA-ICP-MS (Fig. 4).82 LA-ICP-MS images revealed that initial manganese accumulation is inversely related to the β-cell specific radiotracer at 1 h p.i. However, manganese translocates from the exocrine pancreas to the endocrine pancreas by 24 h p.i. This has implications for MEMRI protocols for β-cell mass assessment showing that only late time points actually reflect the secretory function of the pancreas. As an example of possible use of radionuclides in addressing these issues, it would be possible to carry out a similar study correlating acute dynamics of manganese with 52Mn-PET and autoradiography, using picomolar quantities of contrast agent (2000 times lower concentration than MEMRI) that are less likely to perturb manganese handling, alongside chronic manganese concentration measurement by LA-ICP-MS, in healthy and diabetic pancreata. This could then be extended to longitudinal assessment of transplanted β-cells survival and function, as islet transplantation therapy results in temporary insulin independence for patients with type 1 diabetes.
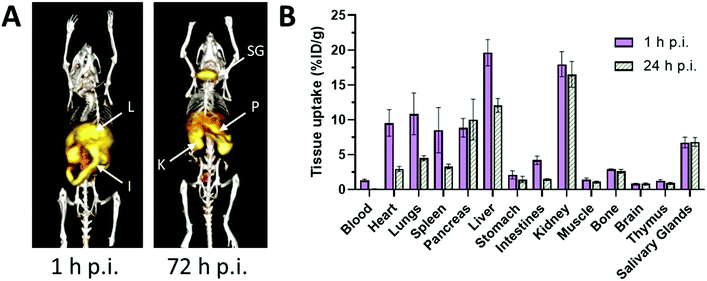 |
| Fig. 3 Biodistribution and pharmacokinetics of 52Mn, administered i.v. as MnCl2, in healthy mice. (A) Representative serial maximum intensity projection (MIP) PET images of healthy mice at 1 and 72 hours p.i. 52Mn is primarily distributed to abdominal organs such as liver (L), kidney (K) and intestines. At 72 h p.i., 52Mn is found predominantly in the kidneys, pancreas (P) and salivary glands (SG). (B) Ex vivo biodistribution of 52Mn in aqueous solution following i.v. injection at 1 and 24 h p.i. Adapted with permission under a Creative Commons Attribution (CC-BY) License from ref. 79, PLoS One, copyright© 2017. | |
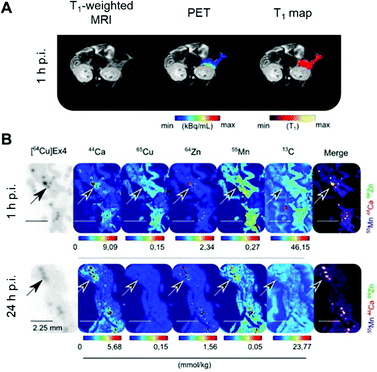 |
| Fig. 4 Assessment of β-cell mass and function by PET/MRI. (A) PET/MRI images of the pancreas in mice following co-injection of the β-cell specific radiotracer [64Cu]Cu-NODAGA-40Lys-Exendin-4 and paramagnetic MnCl2. (B) LA-ICP-MS images revealed typical metal tissue distribution for the pancreas, notably high Zn concentrated in the islets (highlighted by arrows) co-localised with [64Cu]Ex4 autoradiographs. Interestingly, Mn was found predominately in the exocrine tissue at 1 h p.i., and transitioned to islets by 24 h p.i. Adapted with permission under a Creative Commons Attribution (CC-BY) License from ref. 82, Theranostics, copyright© 2020. | |
The limitations of MEMRI applied to neural tracing, discussed above, could in principle be overcome by use of 52Mn PET, although the low spatial resolution of PET imaging would severely limit its use in small animals; this would be a far less significant limitation in humans. Recent studies with 52Mn-PET have indeed mapped the movement of manganese along the mesolimbic and nigrostriatal pathways of the brain,83 as well as olfactory uptake into the frontal cortex and amygdala following intranasal administration in non-human primates.80 Neuronal tract tracing with PET with 52Mn in the clinic might have widespread applications, from imaging neuronal repair following surgery to neuronal development and impairment. The shorter-lived (and hence preferable for dosimetry reasons) 51Mn could also be used as it has fewer unwanted gamma emissions but its use may be limited by its long-lived gamma-emitting daughter 51Cr.
Applications of manganese-PET in cardiology may be of interest following the observation that high myocardial uptake is observed up to 24 h p.i. This has enabled imaging of myocardial infarcts in dogs.84
Group 8 (Fe)
Iron is an important endogenous metal that mediates critical biological processes including oxygen delivery via the iron-containing porphyrin haem in haemoglobin, oxidation via cytochrome P450 and oxidative phosphorylation via FeS clusters in the electron transport chain. A human adult contains ∼3–5 g of iron, 80% of which is found in erythrocyte haemoglobin.85 Free, labile/aquated iron is harmful for the body due to its involvement in the generation of ROS species through Fenton reactions. Therefore, iron, like many other endogenous metals, is highly regulated in the body and sequestered as metalloprotein complexes.86,87 Iron transitions between redox states of +2 and +3 in the body allowing its transport via transferrin to demanding tissues and cells. Non-haem iron is transported into cells by two mechanisms: transferrin (Tf)-bound iron uptake, via receptor-mediated endocytosis after binding to transferrin receptor 1 (TfR1), and non-Tf-bound iron (NTBI) uptake. Under physiological conditions, almost all circulating iron in plasma is bound to Tf,88 the iron-binding sites of which are normally about 30% saturated. Below 16% saturation indicates iron deficiency, and >60% is a sign of iron overload. The trafficking of iron has been studied to understand its homeostatic regulation under normal conditions and how this goes awry in associated diseases.89 For example, the varying concentration of iron in the brain is a subject of investigation of its impact on neurodegenerative disorders such as Wilson's, Alzheimer's and Parkinson's diseases.90,91 However, very few imaging studies have been performed due to lack of ideal iron radioisotopes. To investigate the whole body dynamics of iron handling, three radioisotopes of iron have potential use but all are far from ideal. 55Fe (γ, t1/2 = 2.73 years) and 59Fe (γ, t1/2 = 44 days) are gamma emitters with long half-lives that are non-ideal because of high radiation doses. The cyclotron-produced positron emitting 52Fe (β+, 55%, t1/2 = 8.28 h) has an optimal half-life and positron branching ratio for PET imaging, but interpretation of results remains complicated due to its decay to the positron emitting 52mMn (β+, 96%, t1/2 = 21 min). PET is unable to differentiate between the two radionuclides, and images represent a summed biodistribution of 52Fe and 52mMn.92 Tissue-dependent correction factors for humans have been described by Lubberink et al.,92 although its complex decay continues to make measurements extremely challenging. Nevertheless, it has seen applications in imaging cerebral uptake in Wilson's disease,93 studying 52Fe uptake in blood and bone marrow related disorders, tracking the life cycle of red blood cells94–96 and determining the pharmacokinetics of iron supplements.92,97
In a bid to find a radioactive isotope that gives a useful readout of iron biodistribution without unfavourable decay modes, surrogate markers, notably radioisotopes of gallium, have been studied. Although gallium shares only some of the biological characteristics of iron, it has some highly suitable radioisotopes with a range of nuclear properties that make them attractive for studying limited aspects of iron metabolism provided the differences between iron and gallium are understood and taken into account. Ga3+ shares a similar ionic radius with Fe3+ (62 pm vs. 64.5 pm respectively) in hexacoordinated complexes,98 and hard ligands containing oxygen and nitrogen donor atoms are favoured by both ions. The similarity between Fe3+ and Ga3+ was used as inspiration in the development of tris(hydroxypyridinone) (THP) chelators for gallium; they are now utilised in the clinic as 68Ga-THP-PSMA to detect prostate cancer.5,99–101
66Ga (β+, 57%, t1/2 = 9.5 h), 67Ga (γ, t1/2 = 78 h), 68Ga (β+, 89%, t1/2 = 68 min) and 72Ga (β−, γ, t1/2 = 14.3 h) are the most common radioisotopes of gallium with half-lives suitable for performing nuclear imaging studies. 67Ga is a gamma photon and Auger electron emitter, making it a useful radionuclide in SPECT imaging and potentially as a radionuclide therapy.102,103 It is produced by high-energy proton irradiation of an enriched 67Zn target using a cyclotron. Its long half-life is compatible with imaging studies over several days which is useful for the detection of lymphoma,104,105 inflammation106 and infection.107 However, PET is often favoured over SPECT due to its superior resolution and easier dynamic acquisition in preclinical settings. The advantage of economical and steady supply of 68Ga, due to its generator-based production from 68Ge (t1/2 = 271 d), has led to its ubiquity in imaging centres without access to a medical cyclotron. 68Ga decays to 68Zn via positron emission (89%, 1.89 MeV, t1/2 = 68 min) and, although its half-life is much shorter than 67Ga, it is sufficient for in vivo investigations up to 4 hours.
Like Fe3+, Ga3+ is rapidly sequestered in the blood by serum proteins, mainly transferrin. Ga also binds to lactoferrin, a protein released by neutrophils at sites of inflammation and which has higher iron affinity than transferrin.108 Coupled with the high expression of TfR1, trafficking via transferrin is believed to be the basis for the use of [67Ga]Ga–citrate for imaging infection and inflammation, particularly for identifying infection sites in fever of unknown origin (FUO) patients. In the 1980s, the 67/68Ga transferrin and lactoferrin complexes were used extensively to investigate tumours and their uptake mechanism.109,110 Lactoferrin-bound Ga has been also used to track lymphocytes at the site of inflammation.10667Ga-citrate has shown increased uptake at sites of oedema.111
Uptake of 67/68Ga in infection sites may also be due to binding to siderophores – small iron-chelating molecules secreted by bacteria and fungi to enable them to scavenge iron. Many siderophores form gallium complexes that are isostructural with the iron complexes. For example, both the Fe3+ and Ga3+ complexes of the siderophore desferrioxamine B (DFO) are taken up by bacteria, offering potential for imaging infection.112–116
The above examples illustrate ways in which gallium radionuclides can serve as iron surrogates. However, there are of course profound differences between gallium and iron that need to be accounted for. Unlike Fe3+, Ga3+ cannot be reduced to the +2 oxidation state in physiological conditions. Therefore, Ga3+ does not compete with Fe2+ to bind to haemoglobin and myoglobin,117 nor is it transported by NTBI mechanisms (e.g. DMT-1 and ZIP14). Pharmacokinetic differences have been demonstrated between [67Ga]Ga-citrate and [59Fe]Fe-citrate; uptake of the two tracers in bone, spleen and liver is similar, but there are significant differences in tumour (lymphoma and myeloma) and kidney uptake.118
The vast majority of imaging studies of radiogallium trafficking have been performed with [67Ga]Ga-citrate. Other forms of administered radiogallium, including unchelated forms (acetate, chloride) may result in differing kinetics of transchelation to transferrin, which may complicate interpretation of scans. In the absence of effective chelators, the biodistribution tends to vary, with higher uptake in liver and spleen sometimes seen, possibly due to gallium hydroxide colloid formation which is hard to control reproducibly in the absence of chelators.113,119,120
Group 9 (Co)
The human body contains approximately 1 mg of cobalt, 85% of which is in the form of vitamin B12. Cobalamin (vitamin B12) is characterised by a porphyrin-like corrin nucleus consisting of four pyrrole subunits and a 5,6-dimethylbenzimidazole pendant arm, which together chelate the Co(III) ion in an octahedral geometry. Cobalamin mediates crucial biological processes including DNA synthesis and fatty acid and amino acid metabolism. Therefore, there is significant value in studying the pharmacokinetics and pharmacodynamics of this important vitamin in health and in cancer and other pathologies.121 The Schilling test,122 a historic test for cobalamin deficiency whereby the patient is given an oral dose of cobalamin labelled with a long-lived cobalt radioisotope, 57Co or 58Co (half-life 272 days and 71 days respectively, hence unsuitable for imaging), followed by urine sampling (no imaging was involved). Imaging with a suitable radionuclide of cobalt would produce additional insight into cobalt and B12 metabolism, but this potential has not been exploited to date.
There are several radioactive isotopes of cobalt, including the aforementioned 57Co and 58Co as well as an interesting therapeutic option, the Auger electron emitter 58mCo (t1/2, 9.04 h). Only 55Co (β+, 76%, t1/2 = 17.5 h) is suitable for radionuclide imaging. It is produced using a deuteron beam (8.5 MeV) from a cyclotron via the 54Fe(d,n)55Co reaction.123–125 A limitation on the widespread adoption of this production method is the low natural abundance, and hence high cost, of the target 54Fe. Other production methods are available using, for example, nickel targets, but these are often associated with the production of a larger number of radioactive impurities (e.g. the long lived 56Co (77 d) and 57Co (271.8 d)). The half-life of 55Co is amenable to the radiolabelling of structurally diverse targeting molecules including peptides and nanobodies, mostly using macrocyclic bifunctional chelators based on DOTA,123,126,127 NOTA,123 and linear complexes such as EDTA.128
Application of 55Co as an imaging tool to study cobalt trafficking has scarcely been implemented. Mastren et al. investigated the biodistribution of 55CoCl2 in mice bearing subcutaneous HCT-116 colon cancer tumours as a control for their 55Co-labelled radiopharmaceuticals.123 PET imaging at 24 h and 48 h post i.v. administration highlighted liver, kidney and intestinal uptake (Fig. 5A). Uptake was also observed in the heart and tumour. Ex vivo biodistribution revealed that 55Co retention gradually declined in all tissues from 2 h to 24 h and 48 h p.i. (Fig. 5B). The PET images also highlighted the poor imaging resolution associated with this radionuclide due to its high positron energies (1021 keV, 26% and 1499 keV, 46%). Heart uptake of 55Co has been tentatively attributed to analogy in its transport to calcium. Despite low blood–brain barrier (BBB) penetration in healthy subjects, PET imaging of the brain in multiple sclerosis (MS),129 onset of epileptic seizures,130 stroke31,131,132 and vascular dementia32 has exploited the putative property of 55Co as a PET tracer for calcium flux. The potential analogy to other aspects of calcium metabolism such as calcification have not been studied and the calcium-mimic hypothesis remains speculative. Notably, the 55Co biodistribution study performed by Mastren et al. lacks data on the pancreas, although prior studies in rats have shown some pancreas uptake.133 Unlike other radioactive trace metals which are mostly reabsorbed or retained by the kidneys, cobalt is readily excreted into the urine.
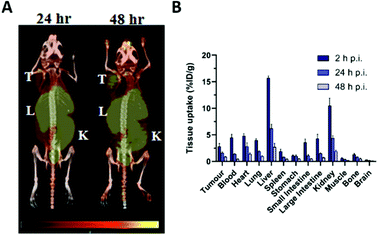 |
| Fig. 5 Imaging cobalt trafficking with 55Co-PET in a subcutaneous colon cancer mouse model. Biodistribution of 55Co in female Nu/Nu mice bearing subcutaneous HCT-116 colon tumours on the upper right flank. (A) PET images at 24 h and 48 h post i.v. administration of 55CoCl2 demonstrate uptake in the tumour (T) and clearance through the liver (L), kidney (K), and intestines. (B) Ex vivo biodistribution at 2, 24 and 48 h p.i. Adapted with permission under a Creative Commons Attribution (CC-BY) License from ref. 111, Mol. Imaging, copyright© 2015. | |
Group 10 (Ni, Pt)
Group 10 transition metals have no known essential role in humans, therefore the main interest in studying their biology is in relation to their toxicology, as environmental contaminants or as metallodrugs. 57Ni (β+, 43.4%, t1/2 = 35.6 h) has been briefly explored as a PET imaging radiolabel for metal chelating drugs (doxorubicin).134 To our knowledge, only one study has actually used 57Ni to study the toxicology of nickel itself with intestinal absorption after oral administration estimated between 1.7 and 10% and uptake predominantly in the kidney and liver.135
195mPt (γ, t1/2 = 4 days) is of particular potential interest to explore the pharmacology and pharmacokinetics of platinum anti-cancer drugs such as cisplatin. It is produced by thermal neutron irradiation of an enriched 194Pt target. Its emissions include several gamma photons (60–100 keV) that can be imaged by SPECT. Clinical trials with radiolabelled cisplatin are currently underway. Abundant Auger emissions also make 195mPt, and 193mPt (t1/2 = 4.33 days), potential therapeutic options.136–138
Group 11 (Cu, Ag, Au)
Copper is an essential trace metal central to many biochemical and physiological processes, and disruption of its trafficking, accumulation and excretion is both cause and consequence of many pathologies, including inherited diseases such as Wilson's disease (WD), diseases of ageing such as dementias, and cancer. It is to be expected, therefore, that molecular imaging of copper trafficking using radiocopper might offer both important insights into the mechanisms underlying these key processes, and methods for diagnosis and monitoring of treatment. Indeed, radioisotopes of copper remain some of the most utilised radiometals described in this review. However, their applications to date have largely involved copper radionuclides as radiolabels for protein- and peptide-conjugates for PET imaging of specific molecular targets. Copper radionuclides with applications in nuclear medicine include: 60Cu (β+, t1/2 = 23.7 min), 61Cu (β+, t1/2 = 3.3 h), 62Cu (β+, t1/2 = 9.76 min), 64Cu (β+, 17.8%, β−, 38.4%, t1/2 = 12.7 h), and 67Cu (β−, 100%, t1/2 = 62.0 h). All except 67Cu offer a range of attributes useful in PET, while 67Cu has great potential for radionuclide therapy. Incorporation of these radionuclides into several copper(II) chelators has been described,139 including a number of bifunctional derivatives which have permitted labelling of biomolecules such as antibodies and peptides. Redox and ligand exchange properties of copper itself are critical in some applications, notably in the bisthiosemicarbazone family of complexes, whose redox chemistry is key to their utility in PET imaging of hypoxia and perfusion.140 Both cyclotron-produced 64Cu and generator-produced 62Cu were utilised for the synthesis of radiocopper bis(thiosemicarbazone) complexes,141–146 but the short half-life of 62Cu is particularly exciting, presenting opportunities for repeated imaging studies with multiple tracers in the same patient. The ability to ship the 62Zn/62Cu generator from cyclotron sites to hospitals (62Zn, t1/2 = 9.3 h) allowed for the clinical use of 62Cu tracers.143,145,146 However, the most widely available and used copper radioisotope is 64Cu, which due to its versatile half-life (t1/2 = 12.7 h), established cyclotron production method and favourable positron energy (0.655 MeV) found multiple applications in clinical oncologic PET imaging with small molecules, peptides and antibodies.147–152
Due to the presence of β+/β− emissions in the 64Cu and 67Cu decay schemes, and Auger electrons in the former, these radioisotopes lend themselves to radionuclide therapy. Therapeutic doses of 64Cu- and 67Cu-labelled tumour-targeting molecules153–158 or radiocopper salts159,160 demonstrated therapeutic efficacy in several preclinical tumour models, and some showed favourable tumour retention161 or partial therapeutic response162,163 in cancer patients.
These applications illustrate the breadth of interest in and utility of copper radionuclides as radiolabels for molecular imaging. However, their application to the study of the trafficking of copper itself have received much less attention and their potential is only recently being realised. Examples of such applications are discussed in the following sections.
Visualising whole-body copper fluxes with 64Cu PET
While PET imaging of copper trafficking is a relatively new field, radioactive copper isotopes have been used for decades to determine copper distribution after oral or i.v. administration in animals, followed by tissue and blood sampling and ex vivo gamma counting.165 Radiocopper blood clearance and excretion studies were also performed in humans to support the diagnosis of patients with Wilson's disease,166–169 an inherited disorder of copper metabolism. PET imaging with 64Cu-based tracers has been introduced to extend observation from specified tissues and body fluids to whole-body copper trafficking. The majority of preclinical and clinical PET studies have utilised oral or i.v. 64Cu formulations of copper chloride164 (the chemical form of 64Cu produced during the purification process). In some studies it has been buffered with acetate170 or citrate,171 or injected as [64Cu]Cu–histidine complexes.172 Upon i.v. administration, weakly chelated 64Cu associates with copper-transporting serum proteins – mainly albumin, which distribute copper to target tissues.173,174 However, how these different administration forms affect the kinetics of copper exchange between serum components and subsequent delivery to cells remains largely unknown. After i.v. administration, ionic radiocopper salts exhibit high liver uptake with hepatobiliary clearance, some spleen and kidney uptake, low brain uptake (at least at 64Cu imaging time-scales of several days) and barely detectable urinary excretion. A recent side-by-side comparison of i.v. and orally administered acetate-buffered 64Cu in healthy human subjects (Fig. 6)170 showed that oral administration led to lower activity in liver and peripheral organs, and higher in the intestines, presumably due to incomplete intestinal absorption.
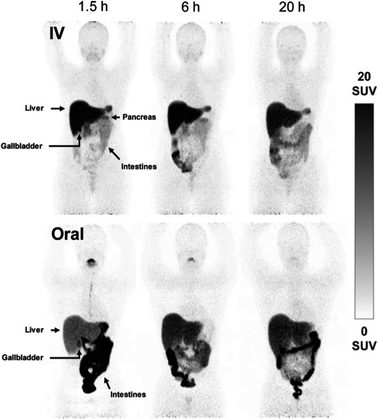 |
| Fig. 6 Comparison of i.v. and orally administered acetate-buffered [64Cu]CuCl2 in healthy human subjects. Whole-body MIPs in two healthy individuals, showing redistribution of 64Cu from the bloodstream to the peripheral organs with pronounced hepatic accumulation following i.v. injection of acetate-buffered 64Cu (upper panels), while the oral administration of 64Cu resulted in significant 64Cu signal in the intestines and slower distribution of 64Cu throughout the body due to limited absorption from the gastrointestinal tract. Adapted with permission under a Creative Commons Attribution (CC-BY) License from ref. 169, EJNMMI Radiopharmacy and Chemistry, copyright© 2020. | |
Cancer
Copper is required for cellular processes implicated in tumour growth, angiogenesis and metastasis.175 Due to their avidity for copper, some tumours can be visualised by PET imaging with i.v. injected ionic radiocopper. This has been demonstrated in preclinical models of hepatoma,176 hepatocellular carcinoma,177 melanoma,159,178 glioblastoma,160,179 neuroblastoma,180 prostate,181,182 head and neck179 and breast183 cancers. The utility of this approach for cancer diagnosis or staging largely depends on its anatomical localisation as [64Cu]Cu–chloride has high uptake in abdominal organs but low bladder accumulation. The low urinary excretion of [64Cu]Cu–chloride allowed for its use in the detection of primary tumour lesions in prostate cancer patients (Fig. 7),164,184 while its low normal brain uptake lends itself to visualising brain cancer lesions, demonstrated in patients with glioblastoma multiforme.185 In the latter case, it is unknown whether high radiocopper accumulation was a result of upregulated copper transport pathways in the tumour lesions or merely of disruption of the BBB, which is often reported in gliomas.186
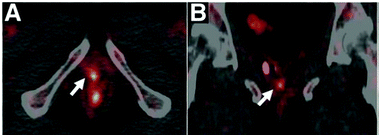 |
| Fig. 7 Clinical PET imaging with i.v. injected [64Cu]Cu–chloride to detect biochemical relapse in prostate cancer patients. The tumour is located in the pelvic region and is indicated with an arrow. This research was originally published in J. Nucl. Med., Piccardo et al.64CuCl2 PET/CT in Prostate Cancer Relapse. J. Nucl. Med., 2018, 59, 444–451. © SNMMI.164 | |
The molecular determinants of high radiocopper accumulation in tumours are not fully understood. 64Cu uptake is partially dependent on the expression of high affinity copper uptake protein 1 (CTR1), which is the main known cellular copper importer. In a prostate cancer xenograft model, silencing CTR1 decreased tumour uptake of [64Cu]Cu–chloride by almost 50%,182 while CTR1 overexpression in breast cancer xenografts doubled [64Cu]Cu–chloride tumour uptake.183 However, the analysis of five cancer lines of various origin found no correlation between their CTR1 mRNA levels and in vivo uptake of [64Cu]Cu–chloride in xenografts.179 Apart from CTR1, radiocopper accumulation in tumours could be modulated by the expression of copper export proteins or intracellular copper scavengers. Since radiocopper in blood is largely associated with serum proteins, such as albumin, its delivery and retention in tumours could also be attributed to the local changes in the vasculature and lymphatic drainage affecting the accumulation of larger molecules.187 Increased 64Cu retention was also reported in the sites of traumatic brain injury188 or muscular injury189 but similarly to the studies in cancer models, the mechanisms of accumulation in the injured tissues were not elucidated.
Several groups have also appreciated the potential of 64Cu PET for visualising the changes in 64Cu trafficking following therapeutic interventions, which could be used to learn about their mechanisms of action, assess their efficacy and visualise side effects. For example, Parmar et al.180 used PET imaging with [64Cu]Cu–chloride to show that the experimental drug dextran-catechin targeting copper metabolism in neuroblastoma cells effectively reduced 64Cu uptake specifically in the tumour, without affecting 64Cu accumulation in other organs. Numerous other molecules, which either bind copper and remove it from the body (copper chelators) or exert toxicity in synergy with elevated intracellular copper, have been evaluated in preclinical cancer models with promising results.175 Some have been evaluated as adjuvant anti-cancer therapies in clinical trials.190–192 One such study incorporated 64Cu PET imaging to assess the tumour avidity for copper.193
Wilson's disease
Wilson's disease (WD) is a recessive genetic disorder caused by mutation of the copper export protein ATP7B.194 It causes systemic copper overload, particularly in the liver and, most intractably, brain. The disease is often undetected due to limitations of current diagnostic methods195 and it is fatal if untreated.196,197 To diagnose WD, clinicians use a combination of blood and urine tests that have high false positive/negative rates.197 Confirmation of high copper content in the liver following biopsy is the most reliable biochemical test but it is invasive and prone to sampling errors, while genetic testing is challenging due to the presence of any of over 700 different ATP7B mutations.194 This motivated several groups to explore the possibility of using non-invasive PET imaging with 64Cu for WD diagnosis. Studies using ATP7B−/− knock-out mice revealed that 24 hours after i.v.198 or oral199 administration of [64Cu]Cu–chloride, radiocopper liver overload and impaired biliary excretion was clearly distinguishable in this WD mouse model, compared to control mice. PET imaging with 64Cu was also used in longitudinal studies tracing whole-body copper fluxes during disease progression, demonstrating age-dependent changes in radiocopper liver uptake and urinary excretion200 (Fig. 8) or brain accumulation201 in the mouse model of WD. In the latter case however, brain uptake of [64Cu]Cu–chloride was very low, which made meaningful comparisons difficult. Clinical studies confirmed the utility of PET imaging as a tool for WD diagnosis. Compared to the healthy controls, WD patients had demonstrably impaired biliary excretion of 64Cu from the liver and as a result, significantly higher ratio of hepatic 64Cu retained at 20 hours and 1.5 hours after i.v. injection of [64Cu]Cu–chloride.202 It was possible to derive an uptake ratio value, which discriminated between the two groups without any overlap, thus presenting a tool to diagnose WD based on the measurable impaired hepatic copper clearance.
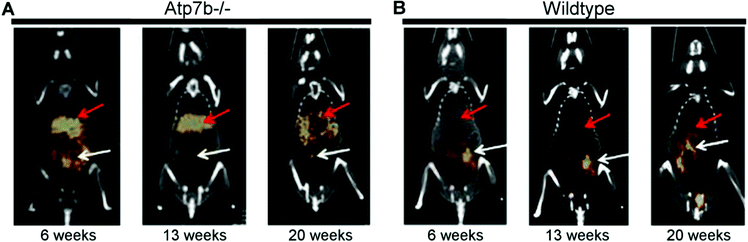 |
| Fig. 8 Preclinical 64Cu-PET imaging in a mouse model of WD. (A) Representative PET/CT images 24 hours after oral administration of [64Cu]Cu–chloride visualise hepatic copper overload and urinary excretion during disease progression in the Atp7B−/− mouse model of WD, compared to reduced uptake in the wildtype control (B). Red arrows and white arrows identify 64Cu present in the liver and gastrointestinal tract respectively. Adapted with permission under a Creative Commons Attribution (CC-BY) License from ref. 199, PLoS One, copyright© 2012. | |
Apart from the potential of 64Cu-based tracers for supporting WD diagnosis, they could also be used to monitor treatment response. PET imaging with [64Cu]Cu–histidine was used to demonstrate how injection of healthy hepatocytes reconstituted normal biliary excretion of 64Cu in a rat model of Wilson's disease.172 Nomura et al.203 used a similar approach in a mouse model of Menkes disease (another genetic disease of aberrant copper trafficking204) and with [64Cu]Cu–chloride PET imaging compared side-by-side how two different copper-modulating molecules, disulfuram and D-penicillamine, re-distributed 64Cu differently between organs (Fig. 9). These examples set a precedent for the integration of 64Cu PET in the preclinical drug development process, as well as in clinical trials for non-invasive assessment of experimental drugs or treatment regimens in individual patients. Copper chelators have been used for treatment of WD for decades205 and other experimental therapies are under development.194 Use of the short-lived radionuclide 62Cu may prove particularly useful in some of these potential applications, since it would allow for repeated scanning of the same subject, e.g. before and after therapy. With the advent of total-body PET and the improvements that this innovative technology provides,2,206 we anticipate studies taking full advantage of shorter-lived radionuclides.
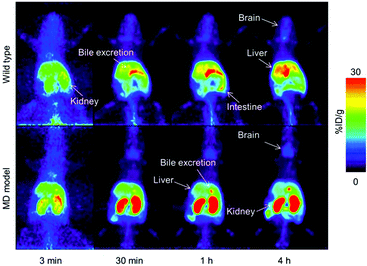 |
| Fig. 9 Imaging treatment response in a mouse model of Menkes disease with 64Cu-PET. Preclinical PET imaging with i.v. injected [64Cu]Cu–chloride to assess 64Cu redistribution after treatment with disulfiram and D-penicillamine. This research was originally published in J. Nucl. Med. Nomura et al. PET imaging analysis with 64Cu in disulfiram treatment for aberrant copper biodistribution in Menkes disease mouse model. J. Nucl. Med., 2014, 55, 845–851. © SNMMI.203 | |
Neurodegeneration
After the liver, the brain is the organ with the second largest copper content in the body,207 which reflects high requirement for copper for enzyme function,208,209 synaptic signalling210 and myelination of neurons.211 With its high rate of aerobic respiration, the brain is particularly vulnerable to redox stress so copper levels need to be very tightly controlled. This could be why brain exhibits remarkably slow kinetics of copper accumulation – upon i.v. injection of radiocopper in rats, the peak of brain accumulation did not occur until 13–17 days p.i., while other tissues were already in the radiotracer efflux phase.212 Due to the unique functions and kinetics of copper in this organ, it is of particular interest to non-invasively study brain copper trafficking in vivo, especially because copper imbalance accompanies numerous neurodegenerative diseases.213,214 Such studies could enhance our understanding of whether deregulated metal homeostasis is an underlying cause or consequence in particular types of neurodegeneration and whether changes are global or regiospecific, or acute or chronic. As shown in WD mice201 and in healthy mice,215 short term (<2 days) imaging with ionic 64Cu gives very low signal in the brain with uptake restricted to the ventricles. This inspired the use of an alternative approach – using a lipophilic tracer [64Cu]Cu–GTSM, a radiocopper bis(thiosemicarbazone) complex which, upon i.v. administration, non-specifically delivers 64Cu to tissues (including brain) and releases it intracellularly due to the shift in the redox environment. Other bis(thiosemicarbazone complexes such as [64Cu]Cu–ATSM and [64Cu]Cu–PTSM only dissociate under more hypoxic conditions,216 and therefore would not be expected to release 64Cu indiscriminately in perfused tissues, as does [64Cu]Cu-GTSM. This property of [64Cu]Cu–GTSM allows for subsequent observation of copper retention and efflux processes, without limitation of initially restricted delivery. PET imaging with i.v. injected [64Cu]Cu–GTSM demonstrated increased 64Cu brain uptake in the βPP/PS1 mouse model of Alzheimer's disease (AD).217 Using the same approach, changes in the 64Cu clearance kinetics and brain biodistribution of 64Cu were reported in two neurodegeneration mouse models: the TASTPM model of AD218 (Fig. 10) and in mice modelling Niemann-Pick C disease,219 a genetic lysosomal storage disorder. The exact intracellular fate of 64Cu released from the [64Cu]Cu–GTSM complex in vivo and how it compares with the fate of 64Cu delivered to cells via CTR1 (which is presumed to follow highly chaperoned endogenous intracellular copper trafficking pathways) remains unknown.
 |
| Fig. 10 Imaging copper trafficking in Alzheimer's disease with 64Cu-PET. Preclinical PET imaging of the head after i.v. injection of [64Cu]Cu–GTSM demonstrating alterations in the brain and spinal cord copper clearance in a mouse model of AD (TASTPM). This research was originally published in J. Nucl. Med. Torres et al. PET Imaging of Copper Trafficking in a Mouse Model of Alzheimer's Disease. J. Nucl. Med., 2016, 57, 109–114. © SNMMI.218 | |
Silver does not have a known biological function but it has long been used in medicine due to its antimicrobial properties.220 An example of a silver-based medicine used nowadays is silver sulfadiazine, which is applied in ointment to disinfect burn wounds. Silver formulations, in metallic and nanoparticle forms, have also been used to coat medical implants and prostheses. However, pharmacokinetics of silver in the body is not fully described, nor are the mechanism underlying potential systemic leaching of silver from the sites of application (as an ointment or from medical devices).220 Silver radioisotopes such as 104Ag (β+, t1/2 = 69 min), 104mAg (β+, t1/2 = 34 min) and 106mAg (β+, t1/2 = 8.3 d) may be useful for imaging the fate of silver-based compounds.
Similarly to silver, gold has a long history in traditional medicine but also forms a basis of modern approved drugs, such as gold salt auranofin approved to treat rheumatoid arthritis.221 Another application of gold is in the synthesis of gold nanoparticles, which received much interest in the field of nanomedicine due to their versatility and stability in biological media. There is also considerable interest in repurposing gold compounds for cancer therapy and as antimicrobial agents. However, despite these applications, there is still no consensus on what the key molecular mechanisms underlying the therapeutic action of gold salts and their toxicity profile may be; knowledge gaps also exist in our understanding of the fate of gold compounds in the body.221 Some of these questions could be addressed by SPECT imaging of gold compounds using gold radioisotopes; indeed such approach has been demonstrated in imaging the pharmacokinetics of gold nanoparticles using 199Au (β−, γ, t1/2 = 3.2 d).222198Au (β−, γ, t1/2 = 2.7 d) has some gamma emissions that can be imaged with SPECT.
Group 12 (Zn, Cd, Hg)
At least 10% of the human genome encodes zinc proteins, amounting to over 3000 proteins. In keeping with its importance, the homeostasis of zinc is regulated by an array of proteins that bind, store and release zinc to meet cellular demand. The ZRT/IRT-like protein (ZIP) family (SLC39A), consisting of 14 members, is responsible for elevating cytosolic zinc levels. It does this by importing zinc into the cell from the extracellular fluid, or through the release of zinc from organelles such as the endoplasmic reticulum and mitochondria. Export of zinc from the cell is controlled by a smaller family of cation diffusion facilitator proteins known as the zinc transporters (ZnT). This export includes moving zinc from the cytosol to the extracellular space, but also the compartmentalisation of zinc into organelles. Disruption of the expression and activity of these transporters, combined with zinc deficiency and overload, have been associated with several diseases including cancer,223–227 Alzheimer's disease228,229 and diabetes.230–232 Despite these hints of the pathological importance of zinc biology, its role in maintaining health and the regulation and dysregulation of their trafficking – from diet to tissues, tissues to tissues and excretion – in diseases such as dementias, cancer and diabetes – remain poorly understood.
Much effort has been invested in recent decades in the synthesis of fluorescence sensors that can be used in vitro to study zinc biology. An extensive library of such probes has been developed,233–242 combining Zn2+ chelating units, such as di-2-picolylamine (DPA), and a fluorescent reporter that, following zinc binding, initiates a change in emission properties (e.g. quantum yield and/or wavelength). Due to the limited tissue penetration associated with fluorescence, these probes have rarely been implemented in vivo. Recent efforts have been made to design medical imaging contrast agents to study zinc in vivo,241,242,245–247 and these have proved useful to study labile zinc pools. However, tools providing complementary information on the dynamic flux of zinc within the body are lacking. 65Zn (β+, t1/2 = 243.8 days) is the longest lived positron-emitting radioisotope of zinc and has the longest history as a research tool; it allows zinc biodistribution to be followed for over a year, which has seen historic use in the study of zinc absorption and metabolism in rodents248,249 and humans.250,251 However, its long half-life means 65Zn is unsuited to imaging and its use depends on tissue biopsy and blood/urine sampling. Its application in animals has diminished in recent years as its long half-life introduces problems associated with contamination and disposal. Nevertheless, 65Zn still remains an important radioisotope for studies zinc in plant biology,252 cell uptake/efflux and as a standard for the calibration of gamma-ray detectors.
In the last ten years, the production and translation of radioactive zinc isotopes more suitable for PET imaging have begun to emerge. The favourable positron emission of 63Zn (β+, 93%, t1/2 = 38.5 min) make it a desirable option for imaging zinc biodistribution in vivo. Degrado et al. successfully established a reliable production method for 63Zn using a low-energy cyclotron via the 63Cu(p,n)63Zn reaction using 14 MeV protons and an isotopically enriched 63Cu-copper nitrate liquid target. Cation exchange purification yielded 63Zn as [63Zn]Zn–citrate ready for in vivo administration. Preliminary PET imaging studies of [63Zn]Zn–citrate in mice following i.v. administration showed that, like other transition radiometals, 63Zn is rapidly cleared from the blood and is distributed primarily to abdominal organs (Fig. 11A). Ex vivo biodistribution at 60 minutes p.i. showed high uptake in the pancreas (standard uptake value (SUV) 8.8 ± 3.2), liver (6.0 ± 1.9), intestine (4.7 ± 2.1), and kidney (4.2 ± 1.3). This distribution was similar to that observed in humans with PET imaging (Fig. 11B and C). These initial human investigations identify potential future uses for this radionuclide, including imaging hepatic, renal and pancreatic function in the context of liver cirrhosis and diabetes respectively. Zinc dyshomeostasis has also been implicated in Alzheimer's disease, with a putative role in the aggregation of β-amyloid proteins that accumulate in brains of patients.229 Against this background, 63Zn-PET has been used in a small clinical study in patients with Alzheimer's disease (Fig. 11B). Although the penetration of 63Zn across the BBB is modest (brain SUV <0.5), uptake was enough to show that there was no significant difference in uptake between patients with Alzheimer's disease compared to healthy elderly volunteers. However, efflux of 63Zn from the brain was slower in the Alzheimer's cohort – in contrast to 64Cu where efflux was shown to be increased in a mouse model of Alzheimer's disease.218,254 This suggests that a tracer that facilitates increased brain uptake, similar in principle to the aforementioned studies with 64Cu–GTSM, using a lipophilic metastable zinc chelator (ionophore), might allow zinc efflux from brain to be imaged as a diagnostic pathological marker.
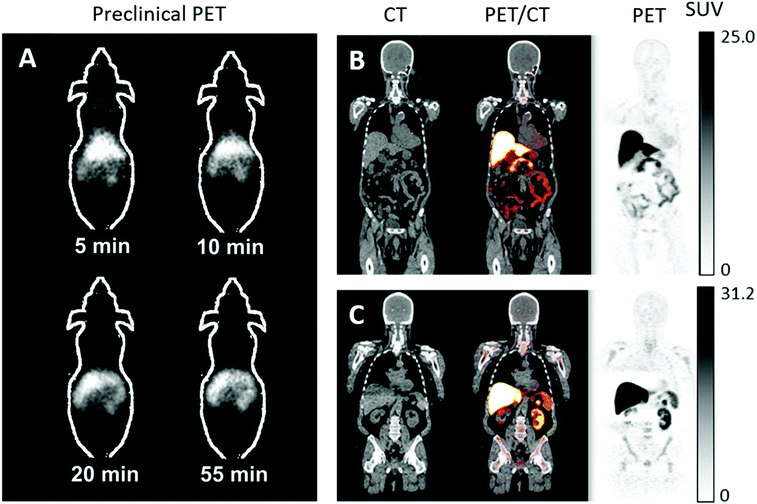 |
| Fig. 11
63Zn-PET can be used to study zinc trafficking in vivo up to 2 hours. (A) Serial small-animal PET images of healthy male B6.SJL mice after i.v. administration of [63Zn]Zn–citrate demonstrate predominant abdominal uptake. (B) CT, PET and fused PET/CT images are shown in a representative patient with Alzheimer's disease and healthy elderly participant (C) at 45 to 70 minutes p.i. of [63Zn]Zn–citrate. Uptake was observed in the liver, pancreas, spleen, kidneys, intestines and bone marrow with no qualitative differences between the groups. Adapted with permission from Degrado et al.243,244 | |
The short half-life of 63Zn limits applications to biological processes with a turnover time less than two hours. This is sufficient to monitor delivery of zinc from blood to tissues, but a longer-lived positron emitter is required to study excretion and redistribution of zinc from tissues to tissues over several days. 62Zn was discussed briefly in the previous section as the parent isotope in the 62Zn/62Cu generator. However, 62Zn also has applications of its own, and has previously been used to image pancreatic exocrine function255 and to label a few zinc-radiopharmaceuticals.256,257 Despite its useful half-life (9.3 hours), which allows for imaging over a few days, its complex decay via62Cu has therefore resulted in this radionuclide being overlooked as a viable option for studying zinc trafficking. In a sample of 62Zn, once equilibrium is reached, more than 95% of the emitted positrons are from the daughter radionuclide 62Cu, raising the question of whether in vivo imaging reflects the distribution of 62Zn or redistribution following decay to 62Cu. Recent observations by Firth et al. are beginning to address this question.253 The authors surmised that after 1 h p.i., each positron emitting radionuclide has spent >98% of its in vivo lifetime as 62Zn (a consequence of the 60-fold longer half-life of 62Zn compared to 62Cu), and unless 62Cu is extremely rapidly redistributed following conversion, then the vast majority of positrons should reflect trafficking of zinc, not copper. Preclinical PET investigations with [62Zn]Zn–citrate in mice (shown in Fig. 12A) demonstrated a similar biodistribution to [63Zn]Zn–citrate at 1 h p.i. but not to [64Cu]Cu–citrate; notably, uptake of 62Zn in the pancreas was much greater than the 64Cu control. Greater accumulation of 62Zn than 64Cu was also noted in the heart, spleen, bone, brain, salivary glands and prostate and seminal vesicles. These differences are consistent with the hypothesis that 62Zn is handled in vivo primarily as zinc and not as copper. The half-life of 62Zn (t1/2 = 9.3 hours) offers longitudinal imaging beyond the 2 hour imaging window of 63Zn, up to 2 days. 62Zn activity at 24 h p.i. was decreased in liver, pancreas and kidney, but was increased in the brain. This radionuclide therefore provides an important tool for imaging zinc trafficking in vivo and can be distributed nationally owing to its longer half-life. Monitoring the kinetics of zinc at very early time points remains complicated thanks to the complex decay of 62Zn, therefore a partnership between 63Zn, for investigations at early time points, and 62Zn, for imaging longer periods of time, may help address important biological questions.
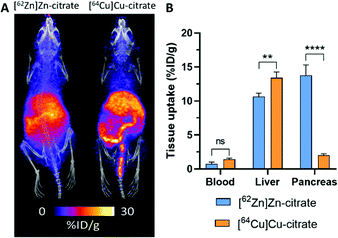 |
| Fig. 12
62Zn-PET can be used to study zinc trafficking in vivo over 2 days despite complex decay via62Cu. (A) Frontal maximum intensity projection (MIP) PET/CT images of female BALB/c mice (10–11 weeks old) injected i.v. with [62Zn]Zn–citrate (left) and [64Cu]Cu–citrate (right). (B) Ex vivo biodistribution at 24 h p.i. shows significant pancreatic uptake for 62Zn contrasting the lower uptake observed with 64Cu. Adapted with permission from ref. 253. | |
Implementing radiozinc to image zinc homeostasis in cancer is also of significant interest. The large body of evidence that supports the decline in endogenous zinc with prostate cancer suggests that zinc may be a useful diagnostic biomarker.223–226 However, diagnostic PET is typically a hot-spot technique, and given that radiozinc delivery is likely to be reduced in prostate cancer, a cold spot representing cancerous tissue compared to high background may limit its clinical utility. Preclinical studies to investigate this are warranted, as well as in other cancers where zinc homeostasis is dysregulated such as breast and pancreatic cancers.
Group 12 also contains two metals that are not biologically essential but are known toxic environmental pollutants: cadmium and mercury. Cadmium toxicity can manifest in adverse effects ranging from renal failure, bone demineralisation and cancer.258 It is a pollutant of particular concern due to its ubiquitous presence in the industrial environment and its long half-life in human tissues (10–30 years in the kidney). Nuclear reactor production of 115Cd (β−, t1/2 = 2.2 days) was described in the context of manufacturing 115mIn generators, since 115mIn is its decay product.259115Cd use was also reported in in vitro transfer studies in human blood cells.260 Another radiocadmium isotope, 115mCd (β−, t1/2 = 44.6 days), was used as a label for cadmium metabolism studies in humans.261 Both radionuclides are unsuitable for imaging.
Mercury is toxic upon inhalation, ingestion and dermal exposure. Its organic form methylmercury is a potent neurotoxin.262 Mercury has been listed by the World Health Organization (WHO) as one of the ten chemicals of major public health concern (along with three other metals/metalloids: arsenic, cadmium and lead), which together contribute to a significant disease and death burden worldwide.263 Gamma emitting 197Hg (γ, 77 keV, t1/2 = 64 h) and 203Hg (β−, γ, 279 keV, t1/2 = 47 days) were historically used in preparation of radiotracers for brain tumour and kidney imaging,264,265 or for radiotracer distribution studies.266,267 Its potential as an imaging tracer or label could be harnessed to study the toxicokinetics of mercury and its compounds and potential chelation therapies.
Groups 13–18 (Pb, As, F, Cl, I)
The elements of groups 13–18 comprise both metals not known to be essential in humans, and non-metals that include some essential elements; hence they are somewhat tangential to this review. Nevertheless several of them represent important links between radionuclide imaging and essential element biology and hence warrant brief discussion here. Radioisotopes of carbon (11C),268 nitrogen (13N),269,270 fluorine (18F)271 have all seen varied use in nuclear medicine for radiolabelling small molecules, although the range of tracers labelled with 13N and 15O is very narrow because of their short half-lives (10 minutes and 2 minutes, respectively). 11C (β+, 100%, t1/2 = 20 min) allows for the synthesis of a very wide range of radiolabelled analogues of biomolecules including key nutrients such as glucose, amino acids, fatty acids and vitamins for in vivo PET imaging.
The classification of fluorine as an essential element is contentious; certainly it has a role in stabilising the mineral component of teeth. When injected in the form of fluoride ions, 18F accumulates quickly at sites of active bone mineral remodelling, such as bone cancers and metastases;272 thus, in principle, [18F]fluoride offers a means to use PET imaging to study fluoride trafficking in other pathological contexts such as calcification in arteries and atherosclerotic plaque (which has recently become a clinical reality),273 or specific trafficking mechanisms (if they exist) in healthy subjects. This application has not yet been implemented. Generally, 18F is used as a radiolabel for organic molecules. In the context of PET imaging of metal trafficking, a 18F radiolabelled fluorescent zinc sensor, consisting of a small lipophilic chelator that can enter cells passively and becomes trapped within them when bound to zinc ions, has recently been reported with the goal of non-invasively imaging endogenous Zn pools in vivo in diseases such as prostate cancer.242 The biological validation and potential of such a radiolabelled probe is yet to be reported. Given the plethora of fluorescence probes for the detection of metals, other 18F radiolabelled metal sensors can be envisaged.
Iodine is an essential trace element, forming a constituent of thyroid hormones. Imaging the trafficking of iodine using gamma-emitting radionuclides 123I (γ, 159 keV, t1/2 = 13 h), 125I (γ, 35 keV, t1/2 = 60 days), and more recently positron-emitting 124I (β+, 23%, t1/2 = 4.2 days), usually in the form of iodide, is one of the oldest manifestations of nuclear medicine, both diagnostically and therapeutically (using the beta-emitter 131I, t1/2 = 8.1 days) in thyroid disease and thyroid cancer. Its accumulation in thyroid, stomach and salivary glands has been attributed since the 1990s to activity of the sodium–iodide symporter in these tissues.274 Hence, radionuclide imaging with iodine radionuclides offers utility in basic studies of iodine trafficking mechanisms, which has not been widely exploited outside clinical diagnosis and therapy.
Chloride is the major extracellular anion in the human body and is responsible for osmotic pressure, acid–base balance and gastric HCl production. The short half-life of 34mCl (β+, 53%, t1/2 = 32 min) necessitates on-site production, and reliable production methods to facilitate this are currently under development. 34mCl provides an underutilised synthon that will allow the synthesis of many unstudied chlorine-containing biological compounds. 34mCl is also of interest, though yet to be studied, to image chlorine transport in vivo. Cystic fibrosis, seizures, osteopetrosis and myotonia are associated with disruption to chloride homeostasis, and our understanding of the underlying biology of these conditions may benefit from PET imaging studies with 34mCl.
Other elements in groups 13–18 deserve mention which, despite having no essential biological role, are of interest in the metallomics field because of their toxicity and industry- or environment-related disease. Lead is one of the key metal environmental pollutants, giving rise to renal, cardiovascular and brain toxicity, and children are the most vulnerable population to lead neurotoxicity.262 Lead has radionuclides with promise as a theranostic pair – 212Pb (β−, t1/2 = 10.6 h) is of interest as an in vivo generator of the alpha emitter bismuth-212 (t1/2 = 60.6 min) and is in early stages of development for radionuclide therapy of cancer when conjugated to tumour-targeting biomolecules,275 and 203Pb (γ, 81%, 279
keV, t1/2 = 51.9 h) is a gamma emitter being developed as an imaging counterpart. Availability of 203Pb may stimulate imaging studies of the trafficking and biodistribution of lead assimilated from the environment. Similarly, the ready availability of 201Tl, discussed in earlier sections as a potassium analogue, could be used to study the toxicology of thallium.
Gallium radionuclides have already been discussed previously in the context of imaging iron trafficking.
Arsenic is of interest both as an environmental toxin, exposure to which causes skin lesions, cardiovascular effects and several types of cancer,276 and as the anticancer drug arsenic trioxide.277 Arsenic radionuclides have received some attention for nuclear medicine applications (e.g. as a radiolabel for cancer-targeting molecules or nanoparticles),222,278,279 but have seen relatively little use to study the pharmacology or toxicology of arsenic. Among the 29 known arsenic radioisotopes, six have a half-life suitable for use in studying arsenic biology; three of these are positron emitters: 71As (β+, 28%, t1/2 = 2.72 days), 72As (β+, 88%, t1/2 = 1.08 days) and 74As (β+, 60%, t1/2 = 17.77 days), and can therefore be imaged with PET. 71As and 72As can be produced by alpha bombardment of a gallium target or via proton bombardment of enriched germanium. Alternative production of 72As using a 72Se/72As generator has also been proposed with similar if not greater radionuclidic purity compared to germanium bombardment,280,281 but the relatively short-lived parent (t1/2 = 8.4 days) limits its use to one month. The long half-life of 71As and 72As is comparable to the established PET radionuclide 89Zr, suggesting similar applications such as attachment to antibodies and proteins for receptor mapping, with the advantage over 89Zr of fewer high-energy gamma emissions. However, arsenic radiochemistry is far less developed and worldwide production and supply of clinical grade 72As is not yet established. Growing availability of radioarsenic suitable for radionuclide imaging will facilitate the study of arsenic biology, particularly to better understand the mechanisms underpinning arsenic poisoning and the mechanism of action and trafficking of the anti-cancer drug, arsenic trioxide.
Conclusion and future directions
Interest in the biological roles of trace metals (and other trace elements) has resurged in recent years alongside developments in analytical methods, both to improve understanding of the relevant biology and to diagnose and treat disease. Methodological developments such as ICP-MS which have been adapted to 2D imaging (e.g. LA-ICP-MS, SIMS etc.) to map elemental distribution at cellular level in cells and tissue slices have been particularly influential. Such methods can deliver a snapshot of in vitro or ex vivo distribution at a single time point on selected tissue samples. Recent developments in production and availability of radioisotopes of biological trace metals, or their surrogates, and toxic metals, are now making it possible to perform molecular imaging of trace metal trafficking at the whole body level in living subjects, including humans. Such radionuclides have conventionally been used largely as radiolabels for larger biomolecules, but by using them to study the biology of the metals themselves, PET and SPECT imaging with these radionuclides can now offer new and complementary insights with a window on the dynamic processes that lead to element distributions, non-invasively and longitudinally. This review has outlined examples of current and potential uses of such radionuclides. By collaborating with centres that host molecular imaging facilities, metallomics and bioinorganic chemistry researchers can now transition their studies from in vitro and cell level to in vivo and clinical investigations. Such studies have progressed to clinical translation in diseases involving changes in copper trafficking, using 64Cu, and the potential is now clear for similar progression to in vivo studies with radioisotopes of zinc, manganese and other essential or toxic elements and metallodrugs. We hope that metallomics researchers will be encouraged to consider making use of these molecular imaging tools to complement elemental analytical tools, to study in vivo, and ultimately in humans, the effects of genetic manipulation, disease and therapy on metal trafficking.
Author contributions
G. F. and P. J. B. were responsible for the concept of this article. Sections on metals within individual groups were distributed among all the authors, G. F. orchestrated the individual sections into a coherent manuscript. G. F., J. E. B., J. J. B. and P. J. B. contributed to editing and reviewing the manuscript. All authors approved the final version of the manuscript.
Conflicts of interest
There are no conflicts to declare.
Acknowledgements
This work was supported by the Centre of Excellence in Medical Engineering funded by the Wellcome Trust and the Engineering and Physical Sciences Research Council (EPSRC) (grant number WT 203148/Z/16/Z); the National Institute for Health Research Biomedical Research Centre based at Guy's and St. Thomas’ NHS Foundation Trust and King's College London (KCL); EPSRC Programme Grant (EP/S032789/1 “MITHRAS”); and the EPSRC Centre for Doctoral Training in Medical Imaging (EP/L015226/1).
References
- T. J. Stewart, Metallomics, 2019, 11, 29–49 CrossRef PubMed.
- R. D. Badawi, H. Shi, P. Hu, S. Chen, T. Xu, P. M. Price, Y. Ding, B. A. Spencer, L. Nardo, W. Liu, J. Bao, T. Jones, H. Li and S. R. Cherry, J. Nucl. Med., 2019, 60, 299–303 CrossRef CAS PubMed.
- E. C. Dijkers, T. H. Oude Munnink, J. G. Kosterink, A. H. Brouwers, P. L. Jager, J. R. De Jong, G. A. Van Dongen, C. P. Schröder, M. N. Lub-De Hooge and E. G. De Vries, Clin. Pharmacol. Ther., 2010, 87, 586–592 CrossRef CAS PubMed.
- A. Afshar-Oromieh, U. Haberkorn, M. Eder, M. Eisenhut and C. M. Zechmann, Eur. J. Nucl. Med. Mol. Imaging, 2012, 39, 1085–1086 CrossRef CAS PubMed.
- J. D. Young, V. Abbate, C. Imberti, L. K. Meszaros, M. T. Ma, S. Y. A. Terry, R. C. Hider, G. E. Mullen and P. J. Blower, J. Nucl. Med., 2017, 58, 1270–1277 CrossRef CAS PubMed.
- J. J. Bartnicka and P. J. Blower, J. Nucl. Med., 2018, 59, 1355–1359 CrossRef CAS PubMed.
- B. F. Palmer, Clin. J. Am. Soc. Nephrol., 2014, 10, 1050–1060 CrossRef PubMed.
- P. Strazzullo and C. Leclercq, Adv. Nutr., 2014, 5, 188–190 CrossRef PubMed.
- M. Zacchia, M. L. Abategiovanni, S. Stratigis and G. Capasso, Kidney Dis., 2016, 2, 72–79 CrossRef PubMed.
- A. G. Therien and R. Blostein, Am. J. Physiol., 2000, 279, C541–C566 CrossRef CAS PubMed.
- S. Fujimaki, T. Maruyama, N. Suzui, N. Kawachi, E. Miwa and K. Higuchi, Plant Cell Physiol., 2015, 56, 943–950 CrossRef CAS PubMed.
- G. Ariño-Estrada, G. S. Mitchell, P. Saha, A. Arzani, S. R. Cherry, E. Blumwald and A. Z. Kyme, Sci. Rep., 2019, 9, 1–9 Search PubMed.
- R. Williams, G. Ruwanpathirana, C. Davey, L. Johnston and D. Plett, J. Nucl. Med., 2020, 61, 3102 Search PubMed.
- J. F. Chatal, F. Rouzet, F. Haddad, C. Bourdeau, C. Mathieu and D. Le Guludec, Front. Med., 2015, 2, 1–7 Search PubMed.
- W. G. Myers, R. E. Bigler and M. C. Graham, Eur. J. Nucl. Med., 1984, 9, 272–277 CrossRef CAS PubMed.
- A. Takami, K. Yoshida, H. Tadokoro, S. Kitsukawa, K. Shimada, M. Sato, K. Suzuki, Y. Masuda and S. Tanada, J. Nucl. Med., 2000, 41, 763–769 CAS.
- P. G. Melon, C. Brihaye, C. Degueldre, M. Guillaume, R. Czichosz, P. Rigo, H. E. Kulbertus, D. Comar, C. J. Anderson and S. R. Bergmann, J. Nucl. Med., 1994, 35, 1116–1224 CAS.
- E. Kazakauskaitė, D. Žaliaduonytė-Pekšienė, E. Rumbinaitė, J. Keršulis, I. Kulakienė and R. Jurkevičius, Medicina, 2018, 54, 1–14 CrossRef PubMed.
- M. R. Jochumsen, J. Sörensen, B. G. Pedersen, J. R. Nyengaard, S. R. P. Krag, J. Frøkiær, M. Borre, K. Bouchelouche and L. P. Tolbod, Eur. J. Nucl. Med. Mol. Imaging, 2021, 48, 532–542 CrossRef CAS PubMed.
- S. Wan, M. Speechly-Dick, L. Menezes, R. Endozo, R. Bell, M. Walker, B. Ganeshan, J. Dickson, I. Kayani and A. Groves, Eur. Hear. J. – Cardiovasc. Imaging, 2021, 22, jeab111.067 CrossRef.
- S. Steien and J. Aaseth, Analyst, 1995, 120, 779–781 RSC.
- H. L. Atkins, T. F. Budinger, E. Lebowitz, A. N. Ansari, M. W. Greene, R. G. Fairchild and K. J. Ellis, J. Nucl. Med., 1977, 18, 133–140 CAS.
- M. Gómez-Río, D. M. Del Valle Torres, A. Rodríguez-Fernández, J. M. Llamas-Elvira, S. O. Lozano, C. R. Font, E. L. Ramírez and M. Katati, Eur. J. Nucl. Med. Mol. Imaging, 2004, 31, 1237–1243 CrossRef PubMed.
- A. M. Sehweil, J. H. McKillop, R. Milroy, R. Wilson, H. M. Abdel-Dayem and Y. T. Omar, Eur. J. Nucl. Med., 1989, 15, 376–379 CrossRef CAS PubMed.
-
D. V. Rao, V. B. Mylavarapu, K. S. R. Sastry and R. S. Howell, DNA Damage by Auger Emitters, Taylor and Francis, London, 1987, pp. 15–26 Search PubMed.
- J. S. Nettleton, R. S. Lawson, M. C. Prescott and I. D. Morris, J. Nucl. Med., 2004, 45, 138–146 CAS.
- F. J. T. Wackers, R. C. Fetterman, J. A. Mattera and J. P. Clements, Semin. Nucl. Med., 1985, 15, 46–66 CrossRef CAS PubMed.
- K. M. Osytek, P. J. Blower, I. M. Costa, G. E. Smith, V. Abbate and S. Y. A. Terry, EJNMMI Res., 2021, 11, 63 CrossRef CAS PubMed.
- A. Rigby, J. E. Blower, P. J. Blower, S. Y. A. Terry and V. Abbate, Nucl. Med. Biol., 2021, 98–99, 1–7 CrossRef CAS PubMed.
- C. Hindorf, S. Chittenden, A. K. Aksnes, C. Parker and G. D. Flux, Nucl. Med. Commun., 2012, 33, 726–732 CrossRef CAS PubMed.
- H. M. L. Jansen, J. Pruim, A. M. V. D. Vliet, A. M. J. Paans, J. M. Hew, E. J. F. Franssen, B. M. De Jong, J. G. W. Kosterink, R. Haaxma and J. Korf, J. Nucl. Med., 1994, 35, 456–460 CAS.
- J. De Reuck, P. Santens, K. Strijckmans and I. Lemahieu, J. Neurol. Sci., 2001, 193, 1–6 CrossRef CAS PubMed.
- R. Hernandez, S. A. Graves, T. Gregg, H. R. VanDeusen, R. J. Fenske, H. N. Wienkes, C. G. England, H. F. Valdovinos, J. J. Jeffery, T. E. Barnhart, G. W. Severin, R. J. Nickles, M. E. Kimple, M. J. Merrins and W. Cai, Diabetes, 2017, 66, 2163–2174 CrossRef CAS PubMed.
- J. A. O’Donoghue, J. S. Lewis, N. Pandit-Taskar, S. E. Fleming, H. Schöder, S. M. Larson, V. Beylergil, S. Ruan, S. K. Lyashchenko, P. B. Zanzonico, W. A. Weber, J. A. Carrasquillo and Y. Y. Janjigian, J. Nucl. Med., 2018, 59, 161–166 CrossRef PubMed.
- F. Man, L. Lim, A. Volpe, A. Gabizon, H. Shmeeda, B. Draper, A. C. Parente-Pereira, J. Maher, P. J. Blower, G. O. Fruhwirth and R. T. M. de Rosales, Mol. Ther., 2019, 27, 219–229 CrossRef CAS PubMed.
- D. Rehder, Metallomics, 2015, 7, 730–742 CrossRef CAS PubMed.
- K. Saatchi, K. H. Thompson, B. O. Patrick, M. Pink, V. G. Yuen, J. H. McNeill and C. Orvig, Inorg. Chem., 2005, 44, 2689–2697 CrossRef CAS PubMed.
- I. F. Chaple and S. E. Lapi, J. Nucl. Med., 2018, 59, 1655–1659 CrossRef CAS PubMed.
- K. T. Kim, M. Y. Eo, T. T. H. Nguyen and S. M. Kim, Int. J. Implant Dent., 2019, 5, 10 CrossRef PubMed.
- D. D. D. Hepburn and J. B. Vincent, J. Inorg. Biochem., 2003, 94, 86–93 CrossRef CAS PubMed.
- W. Maret, Met. Ions Life Sci., 2019, 19, 231–251 CAS.
- R. B. Costello, J. T. Dwyer and R. L. Bailey, Nutr. Rev., 2016, 74, 455–468 CrossRef PubMed.
-
S. Wilbur, H. Abadin, M. Fay, D. Yu, B. Tencza, J. Ingerman, L. Klotzbach and S. James, Toxicological Profile for Chromium, Agency for Toxic Substances and Disease Registry (US), 2012 Search PubMed.
-
K. S. Nijran, Dacie and Lewis Practical Haematology, Elsevier, 12 edn, 2017, pp. 350–365 Search PubMed.
-
L. B. Maia, I. Moura and J. J. G. Moura, in Molybdenum and Tungsten Enzymes: Biochemistry, ed. R. Hille, C. Schulzke and M. L. Kirk, Royal Society of Chemistry, 2017, vol. 2017-Janua, pp. 1–80 Search PubMed.
- R. W. Leggett, Sci. Total Environ., 1997, 206, 147–165 CrossRef CAS PubMed.
- J. M. Régis, A. Esmaylzadeh, J. Jolie, V. Karayonchev, L. Knafla, U. Köster, Y. H. Kim and E. Strub, Nucl. Instrum. Methods Phys. Res., Sect. A, 2020, 955, 163258 CrossRef.
- F. S. Archibald and I. Fridovich, Arch. Biochem. Biophys., 1982, 214, 452–463 CrossRef CAS PubMed.
- E. R. Stadtman, B. S. Berlett and P. B. Chock, Proc. Natl. Acad. Sci. U. S. A., 1990, 87, 384–388 CrossRef CAS PubMed.
- K. Barnese, E. B. Gralla, D. E. Cabelli and J. S. Valentine, J. Am. Chem. Soc., 2008, 130, 4604–4606 CrossRef CAS PubMed.
- P. A. Doble and G. L. G. Miklos, Metallomics, 2018, 10, 1191–1210 CrossRef CAS PubMed.
- H. Yang, J. Wang, X. Yang, F. Wu, Z. Qi, B. Xu, W. Liu and Y. Deng, Toxicol. Ind. Health, 2019, 35, 53–62 CrossRef CAS PubMed.
- L. Strause and P. Saltman, ACS Symp. Ser., 1987, 354, 46–55 CrossRef CAS.
- T. J. V. Bichell, M. Wegrzynowicz, K. G. Tipps, E. M. Bradley, M. A. Uhouse, M. Bryan, K. Horning, N. Fisher, K. Dudek, T. Halbesma, P. Umashanker, A. D. Stubbs, H. K. Holt, G. F. Kwakye, A. M. Tidball, R. J. Colbran, M. Aschner, M. D. Neely, A. Di Di Pardo, V. Maglione, A. Osmand and A. B. Bowman, Biochim. Biophys. Acta, Mol. Basis Dis., 2017, 1863, 1596–1604 CrossRef CAS PubMed.
- T. E. Kehl-Fie and E. P. Skaar, Curr. Opin. Chem. Biol., 2010, 14, 218–224 CrossRef CAS PubMed.
- T. G. Kazi, H. I. Afridi, N. Kazi, M. K. Jamali, M. B. Arain, N. Jalbani and G. A. Kandhro, Biol. Trace Elem. Res., 2008, 122, 1–18 CrossRef CAS PubMed.
- J. A. Roth, NeuroMol. Med., 2009, 11, 281–296 CrossRef CAS PubMed.
- K. Tuschl, E. Meyer, L. E. Valdivia, N. Zhao, C. Dadswell, A. Abdul-Sada, C. Y. Hung, M. A. Simpson, W. K. Chong, T. S. Jacques, R. L. Woltjer, S. Eaton, A. Gregory, L. Sanford, E. Kara, H. Houlden, S. M. Cuno, H. Prokisch, L. Valletta, V. Tiranti, R. Younis, E. R. Maher, J. Spencer, A. Straatman-Iwanowska, P. Gissen, L. A. M. Selim, G. Pintos-Morell, W. Coroleu-Lletget, S. S. Mohammad, S. Yoganathan, R. C. Dale, M. Thomas, J. Rihel, O. A. Bodamer, C. A. Enns, S. J. Hayflick, P. T. Clayton, P. B. Mills, M. A. Kurian and S. W. Wilson, Nat. Commun., 2016, 7, 11601 CrossRef CAS PubMed.
- L. H. Rodan, M. Hauptman, A. M. D’Gama, A. E. Qualls, S. Cao, K. Tuschl, F. Al-Jasmi, J. Hertecant, S. J. Hayflick, M. Wessling-Resnick, E. T. Yang, G. T. Berry, A. Gropman, A. D. Woolf and P. B. Agrawal, Mol. Genet. Metab., 2018, 124, 161–167 CrossRef CAS PubMed.
- M. S. Madejczyk, J. L. Boyer and N. Ballatori, Comp. Biochem. Physiol., Part C: Toxicol. Pharmacol., 2009, 149, 566–571 Search PubMed.
- J. Jankovic, Neurology, 2005, 64, 2021–2028 CrossRef CAS PubMed.
- Y. Jiang, W. Zheng, L. Long, W. Zhao, X. Li, X. Mo, J. Lu, X. Fu, W. Li, S. Liu, Q. Long, J. Huang and E. Pira, Neurotoxicology, 2007, 28, 126–135 CrossRef CAS PubMed.
- R. M. Bowler, S. Nakagawa, M. Drezgic, H. A. Roels, R. M. Park, E. Diamond, D. Mergler, M. Bouchard, R. P. Bowler and W. Koller, Neurotoxicology, 2007, 28, 298–311 CrossRef CAS PubMed.
- R. C. Harris, J. I. Lundin, S. R. Criswell, A. Hobson, L. M. Swisher, B. A. Evanoff, H. Checkoway and B. A. Racette, Parkinsonism Relat. Disord., 2011, 17, 672–676 CrossRef PubMed.
- B. A. Racette, M. Aschner, T. R. Guilarte, U. Dydak, S. R. Criswell and W. Zheng, Neurotoxicology, 2012, 33, 881–886 CrossRef CAS PubMed.
- C. C. Huang, N. S. Chu, C. S. Lu, J. Der Wang, J. L. Tsai, J. L. Tzeng, E. C. Wolters and D. B. Calne, Arch. Neurol., 1989, 46, 1104–1106 CrossRef CAS PubMed.
- J. A. Chalela, L. Bonillha, R. Neyens and A. Hays, Neurocrit. Care, 2011, 14, 456–458 CrossRef PubMed.
- R. F. Butterworth, Neurotoxic. Res., 2010, 18, 100–105 CrossRef PubMed.
- Y. Sanotsky, R. Lesyk, L. Fedoryshyn, I. Komnatska, Y. Matviyenko and S. Fahn, Mov. Disord., 2007, 22, 1337–1343 CrossRef PubMed.
- A. Stepens, I. Logina, V. Liguts, P. Aldiņš, I. Ekšteina, A. Platkajis, I. Martiņsone, E. Terauds, B. Rozentale and M. Donaghy, N. Engl. J. Med., 2008, 358, 1009–1017 CrossRef CAS PubMed.
- R. G. Pautler, A. C. Silva and A. P. Koretsky, Magn. Reson. Med., 1998, 40, 740–748 CrossRef CAS PubMed.
- B. T. Bedenk, S. Almeida-Corrêa, A. Jurik, N. Dedic, B. Grünecker, A. J. Genewsky, S. F. Kaltwasser, C. J. Riebe, J. M. Deussing, M. Czisch and C. T. Wotjak, NeuroImage, 2018, 169, 374–382 CrossRef CAS PubMed.
- W. Deng, M. A. Faiq, C. Liu, V. Adi and K. C. Chan, Front. Neural Circuits, 2019, 13, 35 CrossRef CAS PubMed.
- P. Gawne, F. Man, J. Fonslet, R. Radia, J. Bordoloi, M. Cleveland, P. Jimenez-Royo, A. Gabizon, P. J. Blower, N. Long and R. T. M. De Rosales, Dalton Trans., 2018, 47, 9283–9293 RSC.
- S. A. Graves, R. Hernandez, J. Fonslet, C. G. England, H. F. Valdovinos, P. A. Ellison, T. E. Barnhart, D. R. Elema, C. P. Theuer, W. Cai, R. J. Nickles and G. W. Severin, Bioconjugate Chem., 2015, 26, 2118–2124 CrossRef CAS PubMed.
- J. Fonslet, S. Tietze, A. I. Jensen, S. A. Graves and G. W. Severin, Appl. Radiat. Isot., 2017, 121, 38–43 CrossRef CAS PubMed.
- G. J. Topping, P. Schaffer, C. Hoehr, T. J. Ruth and V. Sossi, Med. Phys., 2013, 40, 1–8 CrossRef PubMed.
- S. A. Graves, R. Hernandez, H. F. Valdovinos, P. A. Ellison, J. W. Engle, T. E. Barnhart, W. Cai and R. J. Nickles, Sci. Rep., 2017, 7, 3033 CrossRef PubMed.
- A. L. Wooten, T. A. Aweda, B. C. Lewis, R. B. Gross and S. E. Lapi, PLoS One, 2017, 12, 1–14 CrossRef PubMed.
- G. Saar, C. M. Millo, L. P. Szajek, J. Bacon, P. Herscovitch and A. P. Koretsky, Mol. Imaging Biol., 2018, 20, 562–574 CrossRef CAS PubMed.
- A. H. Dhyani, X. Fan, L. Leoni, M. Haque and B. B. Roman, Magn. Reson. Imaging, 2013, 31, 508–514 CrossRef CAS PubMed.
- F. C. Michelotti, G. Bowden, A. Küppers, L. Joosten, J. Maczewsky, V. Nischwitz, G. Drews, A. Maurer, M. Gotthardt, A. M. Schmid and B. J. Pichler, Theranostics, 2020, 10, 398–410 CrossRef CAS PubMed.
- H. Napieczynska, G. W. Severin, J. Fonslet, S. Wiehr, A. Menegakis, B. J. Pichler and C. Calaminus, NeuroImage, 2017, 158, 112–125 CrossRef CAS PubMed.
- H. L. Atkins, P. Som, R. G. Fairchild, J. Hui, E. Schachner, A. Goldman and T. Ku, Radiology, 1979, 133, 769–774 CrossRef CAS PubMed.
- D. J. R. Lane, A. M. Merlot, M. L. H. Huang, D. H. Bae, P. J. Jansson, S. Sahni, D. S. Kalinowski and D. R. Richardson, Biochim. Biophys. Acta, Mol. Cell Res., 2015, 1853, 1130–1144 CrossRef CAS PubMed.
- R. Evstatiev and C. Gasche, Gut, 2012, 61, 933–952 CrossRef CAS PubMed.
- K. E. Finberg, Curr. Opin. Hematol., 2013, 20, 208–214 CrossRef CAS PubMed.
- D. R. Richardson and P. Ponka, Biochim. Biophys. Acta, Rev. Biomembr., 1997, 1331, 1–40 CrossRef CAS.
- M. W. Hentze, M. U. Muckenthaler, B. Galy and C. Camaschella, Cell, 2010, 142, 24–38 CrossRef CAS PubMed.
- D. A. Loeffler, J. R. Connor, P. L. Juneau, B. S. Snyder, L. Kanaley, A. J. DeMaggio, H. Nguyen, C. M. Brickman and P. A. LeWitt, J. Neurochem., 1995, 65, 710–716 CrossRef CAS PubMed.
- J. Stankiewicz, S. S. Panter, M. Neema, A. Arora, C. E. Batt and R. Bakshi, Neurotherapeutics, 2007, 4, 371–386 CrossRef CAS PubMed.
- M. Lubberink, V. Tolmachev, S. Beshara and H. Lundqvist, Appl. Radiat. Isot., 1999, 51, 707–715 CrossRef CAS PubMed.
- M. Bruehlmeier, K. L. Leenders, P. Vontobel, C. Calonder, A. Antonini and A. Weindl, J. Nucl. Med., 2000, 41, 781–787 CAS.
- S. Beshara, J. Sorensen, M. Lubberink, V. Tolmachev, B. Langstrom, G. Antoni, B. G. Danielson and H. Lundqvist, Br. J. Haematol., 2003, 120, 853–859 CrossRef CAS PubMed.
- E. J. Freireich, J. F. Ross, T. B. Bayles, C. P. Emerson and S. C. Finch, J. Clin. Invest., 1957, 36, 1043–1058 CrossRef CAS PubMed.
- H. O. Anger and D. C. Van Dyke, Science, 1964, 144, 1587–1589 CrossRef CAS PubMed.
- S. Beshara, H. Lundqvtst, J. Sundin, M. Lubberink, V. Tolmachev, S. Valind, G. Antoni, B. Långström and B. G. Danielson, Br. J. Haematol., 1999, 104, 288–295 CrossRef CAS PubMed.
- R. D. Shannon, Acta Crystallogr., Sect. A: Cryst. Phys., Diffr., Theor. Gen. Crystallogr., 1976, 32, 751–767 CrossRef.
- M. Hofman, P. Eu, P. Jackson, E. Hong, D. Binns, A. Iravani, D. Murphy, C. Mitchell, S. Siva, R. J. Hicks, J. D. Young, P. J. Blower and G. E. Mullen, J. Nucl. Med., 2018, 59, 625–631 CrossRef CAS PubMed.
- M. Kulkarni, S. Hughes, A. Mallia, V. Gibson, J. Young, A. Aggarwal, S. Morris, B. Challacombe, R. Popert, C. Brown, P. Cathcart, P. Dasgupta, V. S. Warbey and G. J. R. Cook, Eur. J. Nucl. Med. Mol. Imaging, 2020, 47, 674–686 CrossRef CAS PubMed.
- A. Afaq, H. Payne, R. Davda, J. Hines, G. J. R. Cook, M. Meagher, D. Priftakis, V. S. Warbey, A. Kelkar, C. Orczyk, A. Mitra, S. Needleman, M. Ferris, G. Mullen and J. Bomanji, J. Nucl. Med., 2021, 62, 1727–1734 CrossRef CAS PubMed.
- M. F. bin Othman, N. R. Mitry, V. J. Lewington, P. J. Blower and S. Y. A. Terry, Nucl. Med. Biol., 2017, 46, 12–18 CrossRef PubMed.
- M. F. bin Othman, E. Verger, I. Costa, M. Tanapirakgul, M. S. Cooper, C. Imberti, V. J. Lewington, P. J. Blower and S. Y. A. Terry, Nucl. Med. Biol., 2020, 80–81, 57–64 CrossRef CAS PubMed.
- D. Front, R. Bar-Shalom, R. Epelbaum, N. Haim, M. W. Ben-Arush, M. Ben-Shahar, M. Gorenberg, U. Kleinhaus, S. Parmett, G. M. Kolodny and O. Israel, J. Nucl. Med., 1993, 34, 2101–2104 CAS.
- E. Even-Sapir and O. Israel, Eur. J. Nucl. Med. Mol. Imaging, 2003, 30, S65–S81 CrossRef CAS PubMed.
- A. Ando, K. Nitta, I. Ando, S. Sanada, S. Katsuda, N. Tonami, T. Hiraki, K. Hisada and H. Ogawa, Eur. J. Nucl. Med., 1990, 17, 21–27 CrossRef CAS PubMed.
- J. A. Ioppolo, D. Caldwell, O. Beiraghi, L. Llano, M. Blacker, J. F. Valliant and P. J. Berti, Nucl. Med. Biol., 2017, 52, 32–41 CrossRef CAS PubMed.
- W. R. Harris, Biochemistry, 1986, 25, 803–808 CrossRef CAS PubMed.
- K.-Y. Tzen, Z. H. Oster, H. N. Wagner and M.-F. Tsan, J. Nucl. Med., 1980, 21, 31–35 CAS.
- M. F. Tsan and U. Scheffel, J. Nucl. Med., 1986, 27, 1215–1219 CAS.
- H. Kohno, K. Ochiai, Y. Ohkubo and A. Kubodera, Radioisotopes, 1987, 36, 261–264 CrossRef CAS PubMed.
- M. Petrik, H. Haas, G. Dobrozemsky, C. Lass-Flörl, A. Helbok, M. Blatzer, H. Dietrich and C. Decristoforo, J. Nucl. Med., 2010, 51, 639–645 CrossRef CAS PubMed.
- M. Petrik, A. Vlckova, Z. Novy, L. Urbanek, H. Haas and C. Decristoforo, Biomed. Pap. Med. Fac. Univ. Palacky. Olomouc. Czech. Repub., 2015, 159, 60–66 CrossRef PubMed.
- M. Petrik, E. Umlaufova, V. Raclavsky, A. Palyzova, V. Havlicek, H. Haas, Z. Novy, D. Dolezal, M. Hajduch and C. Decristoforo, Sci. Rep., 2018, 8, 1–9 CAS.
- A. Pandey, C. Savino, S. H. Ahn, Z. Yang, S. G. Van Lanen and E. Boros, J. Med. Chem., 2019, 62, 9947–9960 CrossRef CAS PubMed.
- F. Mota, A. A. Ordonez, G. Firth, C. A. Ruiz-Bedoya, M. T. Ma and S. K. Jain, J. Med. Chem., 2020, 63, 1964–1977 CrossRef CAS PubMed.
- C. R. Chitambar, Biochim. Biophys. Acta, Mol. Cell Res., 2016, 1863, 2044–2053 CrossRef CAS PubMed.
- R. G. Sephton, G. S. Hodgson, S. De Abrew and A. W. Harris, J. Nucl. Med., 1978, 19, 930–935 CAS.
- A. Autio, H. Virtanen, T. Tolvanen, H. Liljenbäck, V. Oikonen, T. Saanijoki, R. Siitonen, M. Käkelä, A. Schüssele, M. Teräs and A. Roivainen, EJNMMI Res., 2015, 5, 117 Search PubMed.
- T. Ujula, S. Salomäki, A. Autio, P. Luoto, T. Tolvanen, P. Lehikoinen, T. Viljanen, H. Sipilä, P. Härkönen and A. Roivainen, Mol. Imaging Biol., 2010, 12, 259–268 CrossRef PubMed.
- H. Flodh and S. Ullberg, Int. J. Cancer, 1968, 3, 694–699 CrossRef CAS PubMed.
- C. Snow, Arch. Intern. Med., 1999, 159, 2746–2747 CrossRef PubMed.
- T. Mastren, B. V. Marquez, D. E. Sultan, E. Bollinger, P. Eisenbeis, T. Voller and S. E. Lapi, Mol. Imaging, 2015, 14, 526–532 CrossRef CAS PubMed.
- P. E. N. Braad, S. B. Hansen, H. Thisgaard and P. F. Høilund-Carlsen, Phys. Med. Biol., 2015, 60, 3479–3497 CrossRef CAS PubMed.
- H. F. Valdovinos, R. Hernandez, S. Graves, P. A. Ellison, T. E. Barnhart, C. P. Theuer, J. W. Engle, W. Cai and R. J. Nickles, Appl. Radiat. Isot., 2017, 130, 90–101 CrossRef CAS PubMed.
- H. Thisgaard, M. L. Olesen and J. H. Dam, J. Labelled Compd. Radiopharm., 2011, 54, 758–762 CrossRef CAS.
- L. L. Radford, S. Fernandez, R. Beacham, R. El Sayed, R. Farkas, M. Benešová, C. Müller and S. E. Lapi, Pharmaceuticals, 2019, 12, 166 CrossRef CAS PubMed.
- P. Goethals, A. Volkaert, C. Vandewielle, R. Dierckx and N. Lameire, Nucl. Med. Biol., 2000, 27, 77–81 CrossRef CAS PubMed.
- H. M. L. Jansen, A. T. M. Willemsen, L. G. F. Sinnige, A. M. J. Paans, J. M. Hew, E. J. F. Franssen, A. M. Zorgdrager, J. Pruim, J. M. Minderhoud and J. Korf, J. Neurol. Sci., 1995, 132, 139–145 CrossRef CAS PubMed.
- J. De Reuck, K. Vonck, P. Santens, P. Boon, J. De Bleecker, K. Strijckmans and I. Lemahieu, J. Neurol. Sci., 2000, 181, 13–18 CrossRef CAS PubMed.
- H. Stevens, H. M. L. Jansen, J. De Reuck, M. Lemmerling, K. Strijckmans, P. Goethals, I. Lemahieu, B. M. De Jong, A. T. M. Willemsen and J. Korf, J. Neurol. Sci., 1999, 171, 11–18 CrossRef CAS PubMed.
- J. De Reuck, P. Santens, J. Keppens, J. De Bleecker, K. Strijckmans, P. Goethals, I. Lemahieu and J. Korf, Clin. Neurol. Neurosurg., 1999, 101, 15–18 CrossRef CAS PubMed.
- H. M. L. Jansen, S. Knollema, L. V. Van Der Duin, A. T. M. Willemsen, A. Wiersma, E. J. F. Franssen, F. G. M. Russel, J. Korf and A. M. J. Paans, J. Nucl. Med., 1996, 37, 2082–2086 CAS.
- J. Zweit, P. Carnochan, R. Goodall and R. Ott, J. Nucl. Biol. Med., 1994, 38, 18–21 CAS.
- G. D. Nielsen, O. Andersen and M. Jensen, Toxicol. Sci., 1993, 21, 236–243 CrossRef CAS.
- M. S. Uddin, A. Hermanne, B. Scholten, S. Spellerberg, H. H. Coenen and S. M. Qaim, Radiochim. Acta, 2011, 99, 131–135 CrossRef CAS.
- R. A. Nadar, K. Farbod, K. C. van der Schilden, L. Schlatt, B. Crone, N. Asokan, A. Curci, M. Brand, M. Bornhaeuser, M. Iafisco, N. Margiotta, U. Karst, S. Heskamp, O. C. Boerman, J. J. J. P. van den Beucken and S. C. G. Leeuwenburgh, Sci. Rep., 2020, 10, 1–12 CrossRef PubMed.
- R. A. Nadar, G. M. Franssen, N. W. M. Van Dijk, K. Codee-van der Schilden, M. de Weijert, E. Oosterwijk, M. Iafisco, N. Margiotta, S. Heskamp, J. J. J. P. van den Beucken and S. C. G. Leeuwenburgh, Mater. Today Bio., 2020, 9, 100088 CrossRef PubMed.
- M. S. Cooper, M. T. Ma, K. Sunassee, K. P. Shaw, J. D. Williams, R. L. Paul, P. S. Donnelly and P. J. Blower, Bioconjugate Chem., 2012, 23, 1029–1039 CrossRef CAS PubMed.
- T. C. Castle, R. I. Maurer, F. E. Sowrey, M. J. Went, C. A. Reynolds, E. J. L. McInnes and P. J. Blower, J. Am. Chem. Soc., 2003, 125, 10040–10049 CrossRef CAS PubMed.
- Y. Fujibayashi, H. Taniuchi, Y. Yonekura, H. Ohtani, J. Konishi and A. Yokoyama, J. Nucl. Med., 1997, 38, 1155–1160 CAS.
- J. S. Lewis, D. W. McCarthy, T. J. McCarthy, Y. Fujibayashi and M. J. Welch, J. Nucl. Med., 1999, 40, 177–183 CAS.
- N. G. Haynes, J. L. Lacy, N. Nayak, C. S. Martin, D. Dai, C. J. Mathias and M. A. Green, J. Nucl. Med., 2000, 41, 309–314 CAS.
- J. L. J. Dearling, J. S. Lewis, G. E. D. Mullen, M. J. Welch and P. J. Blower, J. Biol. Inorg. Chem., 2002, 7, 249–259 CrossRef CAS PubMed.
- N. Takahashi, Y. Fujibayashi, Y. Yonekura, M. J. Welch, A. Waki, T. Tsuchida, N. Sadato, K. Sugimoto, A. Nakano, J. D. Lee and H. Itoh, Ann. Nucl. Med., 2001, 15, 293–296 CrossRef CAS PubMed.
- T. Z. Wong, J. L. Lacy, N. A. Petry, T. C. Hawk, T. A. Sporn, M. W. Dewhirst and G. Vlahovic, Am. J. Roentgenol., 2008, 190, 427–432 CrossRef PubMed.
- C. J. Anderson, F. Dehdashti, P. D. Cutler, S. W. Schwarz, R. Laforest, L. A. Bass, J. S. Lewis and D. W. McCarthy, J. Nucl. Med., 2001, 42, 213–221 CAS.
- J. E. Mortimer, J. R. Bading, D. M. Colcher, P. S. Conti, P. H. Frankel, M. I. Carroll, S. Tong, E. Poku, J. K. Miles, J. E. Shively and A. A. Raubitschek, J. Nucl. Med., 2014, 55, 23–29 CrossRef CAS PubMed.
- M. Persson, D. Skovgaard, M. Brandt-Larsen, C. Christensen, J. Madsen, C. H. Nielsen, T. Thurison, T. L. Klausen, S. Holm, A. Loft, A. K. Berthelsen, M. Ploug, H. Pappot, K. Brasso, N. Kroman, L. Højgaard and A. Kjaer, Theranostics, 2015, 5, 1303–1316 CrossRef CAS PubMed.
- A. Pfeifer, U. Knigge, T. Binderup, J. Mortensen, P. Oturai, A. Loft, A. K. Berthelsen, S. W. Langer, P. Rasmussen, D. Elema, E. Von Benzon, L. Hjgaard and A. Kjaer, J. Nucl. Med., 2015, 56, 847–854 CrossRef CAS PubMed.
- B. Grubmüller, R. P. Baum, E. Capasso, A. Singh, Y. Ahmadi, P. Knoll, A. Floth, S. Righi, S. Zandieh, C. Meleddu, S. F. Shariat, H. C. Klingler and S. Mirzaei, Cancer Biother. Radiopharm., 2016, 31, 277–286 CrossRef PubMed.
- C. B. Johnbeck, U. Knigge, A. Loft, A. K. Berthelsen, J. Mortensen, P. Oturai, S. W. Langer, D. R. Elema and A. Kjaer, J. Nucl. Med., 2017, 58, 451–457 CrossRef CAS PubMed.
- J. M. Connett, C. J. Anderson, L. W. Guo, S. W. Schwarz, K. R. Zinn, B. E. Rogers, B. A. Siegel, G. W. Philpott and M. J. Welch, Proc. Natl. Acad. Sci. U. S. A., 1996, 93, 6814–6818 CrossRef CAS PubMed.
- J. S. Lewis, M. R. Lewis, P. D. Cutler, A. Srinivasan, M. A. Schmidt, S. W. Schwarz, M. M. Morris, J. P. Miller and C. J. Anderson, Clin. Cancer Res., 1999, 5, 3608–3616 CAS.
- K. Knogler, J. Grünberg, K. Zimmermann, S. Cohrs, M. Honer, S. Ametamey, P. Altevogt, M. Fogel, P. A. Schubiger and I. Novak-Hofer, Clin. Cancer Res., 2007, 13, 603–611 CrossRef CAS PubMed.
- G. Hao, T. Mastren, G. Hassan, W. Silvers, O. Oz and X. Sun, J. Nucl. Med., 2017, 58, 940 Search PubMed.
- C. Cullinane, C. M. Jeffery, P. D. Roselt, E. M. van Dam, S. Jackson, K. Kuan, P. Jackson, D. Binns, J. van Zuylekom, M. J. Harris, R. J. Hicks and P. S. Donnelly, J. Nucl. Med., 2020, 61, 1800–1805 CrossRef CAS PubMed.
- L. McInnes, N. Zia, C. Cullinane, J. V. Z. S. Jackson, J. Stoner, M. Haskali, P. Roselt, E. Van Dam, M. Harris, R. Hicks and P. Donnelly, J. Nucl. Med., 2020, 61, 1215 Search PubMed.
- C. Qin, H. Liu, K. Chen, X. Hu, X. Ma, X. Lan, Y. Zhang and Z. Cheng, J. Nucl. Med., 2014, 55, 812–817 CrossRef CAS PubMed.
- C. Ferrari, A. Niccoli Asabella, C. Villano, B. Giacobbi, D. Coccetti, P. Panichelli and G. Rubini, BioMed Res. Int., 2015, 129764 Search PubMed.
- R. J. Hicks, P. Jackson, G. Kong, R. E. Ware, M. S. Hofman, D. A. Pattison, T. A. Akhurst, E. Drummond, P. Roselt, J. Callahan, R. Price, C. M. Jeffery, E. Hong, W. Noonan, A. Herschtal, L. J. Hicks, A. Hedt, M. Harris, B. M. Paterson and P. S. Donnelly, J. Nucl. Med., 2019, 60, 777–785 CrossRef CAS PubMed.
- S. J. DeNardo, G. L. DeNardo, D. L. Kukis, S. Shen, L. A. Kroger, D. A. Denardo, D. S. Goldstein, G. R. Mirick, Q. Salako, L. F. Mausner, S. C. Srivastava and C. F. Meares, J. Nucl. Med., 1999, 40, 302–310 CAS.
- R. T. O’Donnell, G. L. DeNardo, D. L. Kukis, K. R. Lamborn, S. Shen, A. Yuan, D. S. Goldstein, C. E. Carr, G. R. Mirick and S. J. DeNardo, J. Nucl. Med., 1999, 40, 2014–2020 Search PubMed.
- A. Piccardo, F. Paparo, M. Puntoni, S. Righi, G. Bottoni, L. Bacigalupo, S. Zanardi, A. DeCensi, G. Ferrarazzo, M. Gambaro, F. G. Ruggieri, F. Campodonico, L. Tomasello, L. Timossi, S. Sola, E. Lopci and M. Cabria, J. Nucl. Med., 2018, 59, 444–451 CrossRef CAS PubMed.
-
M. C. Linder, Biochemistry of Copper, Springer US, Boston, MA, 1991 Search PubMed.
- A. G. Bearn and H. G. Kunkel, J. Lab. Clin. Med., 1955, 45, 623–631 CAS.
- J. A. Bush, J. P. Mahoney, H. Markowitz, C. J. Gubler, G. E. Cartwright and M. M. Wintrobe, J. Clin. Invest., 1955, 34, 1766–1778 CrossRef CAS PubMed.
- W. N. Jensen and H. Kamin, J. Lab. Clin. Med., 1957, 49, 200–210 CAS.
- A. Członkowska, M. Rodo, A. Wierzchowska-Ciok, L. Smolinski and T. Litwin, Liver Int., 2018, 38, 1860–1866 CrossRef PubMed.
- K. Kjærgaard, T. D. Sandahl, K. Frisch, K. H. Vase, S. Keiding, H. Vilstrup, P. Ott, L. C. Gormsen and O. L. Munk, EJNMMI Radiopharm. Chem., 2020, 5, 1–12 CrossRef PubMed.
- C. Raynaud, D. Comar, M. Dutheil, P. Blanchon, O. Monod, R. Parrot and M. Rymer, J. Nucl. Med., 1973, 14, 947–950 CAS.
- R. Bahde, S. Kapoor, K. K. Bhargava, M. L. Schilsky, C. J. Palestro and S. Gupta, J. Nucl. Med., 2012, 53, 961–968 CrossRef CAS PubMed.
- S. H. Lee, R. Lancey, A. Montaser, N. Madani and M. C. Linder, Proc. Soc. Exp. Biol. Med., 1993, 203, 428–439 CrossRef CAS PubMed.
- M. C. Linder, Metallomics, 2016, 8, 887–905 CrossRef CAS PubMed.
- D. Denoyer, S. Masaldan, S. La Fontaine and M. A. Cater, Metallomics, 2015, 7, 1459–1476 CrossRef CAS PubMed.
- F. Peng, J. Liu, J. S. Wu, X. Lu and O. Muzik, Mol. Imaging Biol., 2005, 7, 325–329 CrossRef PubMed.
- H. Zhang, H. Cai, X. Lu, O. Muzik and F. Peng, Acad. Radiol., 2011, 18, 1561–1568 CrossRef PubMed.
- L. Jiang, Y. Tu, X. Hu, A. Bao, H. Chen, X. Ma, T. Doyle, H. Shi and Z. Cheng, Sci. Rep., 2017, 7, 1–10 CrossRef PubMed.
- J. T. Jørgensen, M. Persson, J. Madsen and A. Kjær, Nucl. Med. Biol., 2013, 40, 345–350 CrossRef PubMed.
- A. Parmar, G. Pascali, F. Voli, L. Lerra, E. Yee, A. Ahmed-Cox, K. Kimpton, G. Cirillo, A. Arthur, D. Zahra, G. Rahardjo, G. J. Liu, N. Lengkeek, F. Saletta, A. Charil, M. Kavallaris and O. Vittorio, Theranostics, 2018, 8, 5645–5659 CrossRef CAS PubMed.
- F. Peng, X. Lu, J. Janisse, O. Muzik and A. F. Shields, J. Nucl. Med., 2006, 47, 1649–1652 CAS.
- H. Cai, J. S. Wu, O. Muzik, J. T. Hsieh, R. J. Lee and F. Peng, J. Nucl. Med., 2014, 55, 622–628 CrossRef CAS PubMed.
- K. Il Kim, S. J. Jang, J. H. Park, Y. J. Lee, T. S. Lee, K. S. Woo, H. Park, J. G. Choe, G. Il An and J. H. Kang, J. Nucl. Med., 2014, 55, 1692–1698 CrossRef PubMed.
- E. Capasso, S. Durzu, S. Piras, S. Zandieh, P. Knoll, A. Haug, M. Hacker, C. Meleddu and S. Mirzaei, Ann. Nucl. Med., 2015, 29, 482–488 CrossRef CAS PubMed.
- P. Panichelli, C. Villano, A. Cistaro, A. Bruno, F. Barbato, A. Piccardo and A. Duatti, Cancer Biother. Radiopharm., 2016, 31, 159–167 CrossRef CAS PubMed.
- L. G. Dubois, L. Campanati, C. Righy, I. D’Andrea-Meira, T. C. L. D. S. E. Spohr, I. Porto-Carreiro, C. M. Pereira, J. Balça-Silva, S. A. Kahn, M. F. DosSantos, M. D. A. R. Oliveira, A. Ximenes-da-Silva, M. C. Lopes, E. Faveret, E. L. Gasparetto and V. Moura-Neto, Front. Cell. Neurosci., 2014, 8, 418 Search PubMed.
- H. Maeda, H. Nakamura and J. Fang, Adv. Drug Delivery Rev., 2013, 65, 71–79 CrossRef CAS PubMed.
- F. Peng, O. Muzik, J. Gatson, S. G. Kernie and R. Diaz-Arrastia, J. Nucl. Med., 2015, 56, 1252–1257 CrossRef CAS PubMed.
- F. Xie, H. Cai and F. Peng, Am. J. Nucl. Med. Mol. Imaging, 2017, 7, 33–39 CAS.
- S. J. O’Day, A. M. M. Eggermont, V. Chiarion-Sileni, R. Kefford, J. J. Grob, L. Mortier, C. Robert, J. Schachter, A. Testori, J. Mackiewicz, P. Friedlander, C. Garbe, S. Ugurel, F. Collichio, W. Guo, J. Lufkin, S. Bahcall, V. Vukovic and A. Hauschild, J. Clin. Oncol., 2013, 31, 1211–1218 CrossRef PubMed.
-
D. George, A Phase Ib Study of Intravenous Copper Loading With Oral Disulfiram in Metastatic, Castration Resistant Prostate Cancer, https://clinicaltrials.gov/show/NCT02963051, accessed 28 July 2020.
- S. Baldari, G. Di Rocco and G. Toietta, Int. J. Mol. Sci., 2020, 21, 1–20 Search PubMed.
- T. Zhang, J. Kephart, E. Bronson, M. Anand, C. Daly, I. Spasojevic, H. Berg, O. G. James, P. Healy, S. Halabi, M. R. Harrison, A. J. Armstrong and D. J. George, J. Clin. Oncol., 2020, 38, 96 Search PubMed.
- A. Czlonkowska, T. Litwin, P. Dusek, P. Ferenci, S. Lutsenko, V. Medici, J. K. Rybakowski, K. H. Weiss and M. L. Schilsky, Nat. Rev. Dis. Prim., 2018, 4, 21 CrossRef PubMed.
- A. Ryan, S. J. Nevitt, O. Tuohy and P. Cook, Cochrane Database Syst. Rev., 2019, 2019, CD012267 Search PubMed.
- E. A. Roberts and M. L. Schilsky, Hepatology, 2008, 47, 2089–2111 CrossRef CAS PubMed.
- P. Ferenci, A. Czlonkowska, W. Stremmel, R. Houwen, W. Rosenberg, M. Schilsky, P. Jansen, D. Moradpour and J. Gitlin, J. Hepatol., 2012, 56, 671–685 CrossRef PubMed.
- F. Peng, S. Lutsenko, I. Sun and O. Muzik, Mol. Imaging Biol., 2012, 14, 70–78 CrossRef PubMed.
- F. Peng, S. Lutsenko, X. Sun and O. Muzik, Mol. Imaging Biol., 2012, 14, 600–607 CrossRef PubMed.
- L. W. Gray, F. Peng, S. A. Molloy, V. S. Pendyala, A. Muchenditsi, O. Muzik, J. Lee, J. H. Kaplan and S. Lutsenko, PLoS One, 2012, 7, e38327 CrossRef CAS PubMed.
- F. Xie, Y. Xi, J. M. Pascual, O. Muzik and F. Peng, Metab. Brain Dis., 2017, 32, 717–726 CrossRef CAS PubMed.
- T. D. Sandahl, L. C. Gormsen, K. Kjærgaard, M. H. Vendelbo, D. E. Munk, O. L. Munk, D. Bender, S. Keiding, K. H. Vase, K. Frisch, H. Vilstrup and P. Ott, Hepatology, 2022, 1–10 Search PubMed.
- S. Nomura, S. Nozaki, T. Hamazaki, T. Takeda, E. Ninomiya, S. Kudo, E. Hayashinaka, Y. Wada, T. Hiroki, C. Fujisawa, H. Kodama, H. Shintaku and Y. Watanabe, J. Nucl. Med., 2014, 55, 845–851 CrossRef CAS PubMed.
- P. Thomas, M. Mathew and P. R. Roshni, Natl. J. Physiol., Pharm. Pharmacol., 2017, 7, 1447–1448 Search PubMed.
- I. Mohr and K. H. Weiss, Ann. Transl. Med., 2019, 7, S69–S69 CrossRef CAS PubMed.
- S. R. Cherry, T. Jones, J. S. Karp, J. Qi, W. W. Moses and R. D. Badawi, J. Nucl. Med., 2018, 59, 3–12 CrossRef CAS PubMed.
- T. Lech and J. K. Sadlik, Biol. Trace Elem. Res., 2007, 118, 10–15 CrossRef CAS PubMed.
- D. Bousquet-Moore, R. E. Mains and B. A. Eipper, J. Neuroci. Res., 2010, 88, 2535–2545 CrossRef CAS PubMed.
- T. V. Vendelboe, P. Harris, Y. Zhao, T. S. Walter, K. Harlos, K. El Omari and H. E. M. Christensen, Sci. Adv., 2016, 2, 1–10 Search PubMed.
- N. D’Ambrosi and L. Rossi, Neurochem. Int., 2015, 90, 36–45 CrossRef PubMed.
- S. Takikita, T. Takano, T. Narita and Y. Maruo, Mol. Genet. Metab. Reports, 2015, 4, 25–29 CrossRef CAS PubMed.
- C. A. Owen, Am. J. Physiol., 1971, 221, 1722–1727 CrossRef CAS PubMed.
- A. I. Bush and R. E. Tanzi, Neurotherapeutics, 2008, 5, 421–432 CrossRef CAS PubMed.
- O. Bandmann, K. H. Weiss and S. G. Kaler, Lancet
Neurol., 2015, 14, 103–113 CrossRef CAS PubMed.
- F. Peng, F. Xie and O. Muzik, Aging Dis., 2018, 9, 109 CrossRef PubMed.
- Z. Xiao, P. S. Donnelly, M. Zimmermann and A. G. Wedd, Inorg. Chem., 2008, 47, 4338–4347 CrossRef CAS PubMed.
- M. T. Fodero-Tavoletti, V. L. Villemagne, B. M. Paterson, A. R. White, Q. X. Li, J. Camakaris, G. O’keefe, R. Cappai, K. J. Barnham and P. S. Donnelly, J. Alzheimer's Dis., 2010, 20, 49–55 CAS.
- J. B. Torres, E. M. Andreozzi, J. T. Dunn, M. Siddique, I. Szanda, D. R. Howlett, K. Sunassee and P. J. Blower, J. Nucl. Med., 2016, 57, 109–114 CrossRef CAS PubMed.
- J. Baguña Torres, Z. Yu, J. Bordoloi, K. Sunassee, D. Smith, C. Smith, O. Chen, R. Purchase, K. Tuschl, J. Spencer, F. Platt and P. J. Blower, Biometals, 2019, 32, 293–306 CrossRef PubMed.
- H. D. Betts, C. Whitehead and H. H. Harris, Metallomics, 2021, 13, 1–12 CrossRef PubMed.
- A. Balfourier, J. Kolosnjaj-Tabi, N. Luciani, F. Carn, F. Gazeau and C. J. Murphy, Proc. Natl. Acad. Sci. U. S. A., 2020, 117, 22639–22648 CrossRef CAS PubMed.
- J. Pellico, P. J. Gawne and R. T. M. De Rosales, Chem. Soc. Rev., 2021, 50, 3355–3423 RSC.
- R. B. Franklin, P. Feng, B. Milon, M. M. Desouki, K. K. Singh, A. Kajdacsy-Balla, O. Bagasra and L. C. Costello, Mol. Cancer, 2005, 4, 1–13 CrossRef PubMed.
- L. C. Costello and R. B. Franklin, Mol. Cancer, 2006, 5, 17 CrossRef PubMed.
- L. C. Costello and R. B. Franklin, J. Biol. Inorg. Chem., 2011, 16, 3–8 CrossRef CAS PubMed.
- V. Kolenko, E. Teper, A. Kutikov and R. Uzzo, Nat. Rev. Urol., 2013, 10, 219–226 CrossRef CAS PubMed.
- B. X. Hoang, B. Han, D. G. Shaw and M. Nimni, Eur. J. Cancer Prev., 2016, 25, 457–461 CrossRef CAS PubMed.
- M. A. Lovell, J. D. Robertson, W. J. Teesdale, J. L. Campbell and W. R. Markesbery, J. Neurol. Sci., 1998, 158, 47–52 CrossRef CAS PubMed.
- T. J. A. Craddock, J. A. Tuszynski, D. Chopra, N. Casey, L. E. Goldstein, S. R. Hameroff and R. E. Tanzi, PLoS One, 2012, 7, e33552 CrossRef CAS PubMed.
- X. Miao, W. Sun, Y. Fu, L. Miao and L. Cai, Front. Med., 2013, 7, 31–52 CrossRef PubMed.
- R. K. Mitchell, M. Hu, P. L. Chabosseau, M. C. Cane, G. Meur, E. A. Bellomo, R. Carzaniga, L. M. Collinson, W.-H. Li, D. J. Hodson and G. A. Rutter, Mol. Endocrinol., 2016, 30, 77–91 CrossRef CAS PubMed.
- S. Kleiner, D. Gomez, B. Megra, E. Na, R. Bhavsar, K. Cavino, Y. Xin, J. Rojas, G. Dominguez-Gutierrez, B. Zambrowicz, G. Carrat, P. Chabosseau, M. Hu, A. J. Murphy, G. D. Yancopoulos, G. A. Rutter and J. Gromada, Proc. Natl. Acad. Sci. U. S. A., 2018, 115, E7642–E7649 CAS.
- Y. Wu, X. Peng, B. Guo, J. Fan, Z. Zhang, J. Wang, A. Cui and Y. Gao, Org. Biomol. Chem., 2005, 3, 1387–1392 RSC.
- E. M. Nolan, J. Jaworski, K. I. Okamoto, Y. Hayashi, M. Sheng and S. J. Lippard, J. Am. Chem. Soc., 2005, 127, 16812–16823 CrossRef CAS PubMed.
- Y. Mikata, M. Wakamatsu, A. Kawamura, N. Yamanaka, S. Yano, A. Odani, K. Morihiro and S. Tamotsu, Inorg. Chem., 2006, 45, 9262–9268 CrossRef CAS PubMed.
- Y. Mikata, A. Yamanaka, A. Yamashita and S. Yano, Inorg. Chem., 2008, 47, 7295–7301 CrossRef CAS PubMed.
- H. Wang, Q. Gan, X. Wang, L. Xue and S. Liu, Org. Lett., 2007, 9, 4995–4998 CrossRef CAS PubMed.
- B. A. Wong, S. Friedle and S. J. Lippard, Inorg. Chem., 2009, 48, 7009–7011 CrossRef CAS PubMed.
- Y. Zhang, X. Guo, W. Si, L. Jia and X. Qian, Org. Lett., 2008, 10, 473–476 CrossRef CAS PubMed.
- Y. Zhang, X. Guo, L. Jia, S. Xu, Z. Xu, L. Zheng and X. Qian, Dalton Trans., 2012, 41, 11776–11782 RSC.
- G. J. Stasiuk, F. Minuzzi, M. Sae-Heng, C. Rivas, H.-P. Juretschke, L. Piemonti, P. R. Allegrini, D. Laurent, A. R. Duckworth, A. Beeby, G. A. Rutter and N. J. Long, Chem. – Eur. J., 2015, 21, 5023–5033 CrossRef CAS PubMed.
- T. W. Price, G. Firth, C. J. Eling, M. Kinnon, N. J. Long, J. Sturge and G. J. Stasiuk, Chem. Commun., 2018, 54, 3227–3230 RSC.
- T. R. DeGrado, M. K. Pandey, J. F. Byrne, H. P. Engelbrecht, H. Jiang, A. B. Packard, K. A. Thomas, M. S. Jacobson, G. L. Curran and V. J. Lowe, J. Nucl. Med., 2014, 55, 1348–1354 CrossRef CAS PubMed.
- T. R. DeGrado, B. J. Kemp, M. K. Pandey, H. Jiang, T. M. Gunderson, L. R. Linscheid, A. R. Woodwick, D. M. McConnell, J. G. Fletcher, G. B. Johnson, R. C. Petersen, D. S. Knopman and V. J. Lowe, Mol. Imaging, 2016, 15, 1–10 CrossRef CAS PubMed.
- J. Yu, A. F. Martins, C. Preihs, V. C. Jordan, S. Chirayil and P. Zhao, J. Am. Chem. Soc., 2015, 137, 14173–14179 CrossRef CAS PubMed.
- A. F. Martins, V. Clavijo Jordan, F. Bochner, S. Chirayil, N. Paranawithana, S. Zhang, S. T. Lo, X. Wen, P. Zhao, M. Neeman and A. D. Sherry, J. Am. Chem. Soc., 2018, 140, 17456–17464 CrossRef CAS PubMed.
- S. Chirayil, V. C. Jordan, A. F. Martins, N. Paranawithana, S. J. Ratnakar and A. D. Sherry, Inorg. Chem., 2021, 60, 2168–2177 CrossRef CAS PubMed.
- S. A. Gunn, T. C. Gould, S. S. Ginori and J. G. Morse, Proc. Soc. Exp. Biol. Med., 1955, 88, 556–558 CrossRef CAS PubMed.
-
J. E. Ballou, HW-SA [reports]. U.S. At. Energy Comm., 1960, HW-63047, pp. 1–23.
- H. Spencer, B. Rosoff, A. Feldstein, S. H. Cohn and E. Gusmano, Radiat. Res., 1965, 24, 432 CrossRef.
- D. C. Barber, N. J. Logie and J. R. Mallard, Br. J. Urol., 1969, 41, 474 CrossRef CAS PubMed.
- N. Suzui, Y. G. Yin, S. Ishii, H. Sekimoto and N. Kawachi, Plant Methods, 2017, 13, 1–6 CrossRef PubMed.
-
G. Firth, PhD thesis, King's College London, 2022.
- E. M. Andreozzi, J. B. Torres, K. Sunassee, J. Dunn, S. Walker-Samuel, I. Szanda and P. J. Blower, Metallomics, 2017, 9, 1622–1633 CrossRef CAS PubMed.
- Y. Fujibayashi, H. Saji, K. Kawai, Y. Unuma, S. Miyata, T. Okuno, R. Hosotani, K. Inoue, H. Adachi, K. Horiuchi, K. Torizuka and A. Yokoyama, Int. J. Nucl. Med. Biol., 1986, 12, 447–451 CrossRef CAS PubMed.
- A. R. Jalilian, B. Fateh, M. Ghergherehchi, A. Karimian, S. Moradkhani, M. Kamali-Dehghan and F. Tabeie, Nukleonika, 2005, 50, 143–148 CAS.
- M. Tamura, H. Matsui, S. Hirohara, K. Kakiuchi, M. Tanihara, N. Takahashi, K. Nakai, Y. Kanai, H. Watabe and J. Hatazawa, Bioorg. Med. Chem., 2014, 22, 2563–2570 CrossRef CAS PubMed.
- L. Järup and A. Åkesson, Toxicol. Appl. Pharmacol., 2009, 238, 201–208 CrossRef PubMed.
- G. J. Ehrhardt, W. Volkert, W. F. Goeckeler and D. N. Kapsch, J. Nucl. Med., 1983, 24, 349–352 CAS.
- J. J. Walsh and G. E. Burch, Exp. Biol. Med., 1959, 102, 348–349 CrossRef CAS PubMed.
- P. R. Flanagan, J. S. McLellan, J. Haist, G. Cherian, M. J. Chamberlain and L. S. Valberg, Gastroenterology, 1978, 74, 841–846 CrossRef CAS.
- P. Grandjean and P. Landrigan, Lancet, 2006, 368, 2167–2178 CrossRef CAS.
- H. J. Gibb, A. Barchowsky, D. Bellinger, P. M. Bolger, C. Carrington, A. H. Havelaar, S. Oberoi, Y. Zang, K. O’Leary and B. Devleesschauwer, Environ. Res., 2019, 174, 188–194 CrossRef CAS PubMed.
- R. H. Greenlaw and M. Quaife, Radiology, 1962, 78, 970–973 CrossRef CAS PubMed.
- M. K. Loken, L. O. Wigdahl, J. M. Gilson and E. V. Staab, J. Nucl. Med., 1966, 7, 209–218 CAS.
- B. Aberg, L. Ekman, R. Falk, U. Greitz, G. Persson and J. Snihs, Arch. Environ. Heal. An Int. J., 1969, 19, 478–484 CrossRef CAS PubMed.
- M. Aschner and T. W. Clarkson, Teratology, 1988, 38, 145–155 CrossRef CAS PubMed.
- C. Taddei and A. D. Gee, J. Labelled Compd. Radiopharm., 2018, 61, 237–251 CrossRef CAS PubMed.
- J. E. Blower, S. F. Cousin and A. D. Gee, EJNMMI Radiopharm. Chem., 2017, 2, 16 CrossRef PubMed.
- J. E. Blower, M. T. Ma, F. A. I. Al-Salemee and A. D. Gee, Chem. Commun., 2021, 57, 4962–4965 RSC.
- O. Jacobson, D. O. Kiesewetter and X. Chen, Bioconjugate Chem., 2015, 26, 1–18 CrossRef CAS PubMed.
- A. B. Apolo, L. Lindenberg, J. H. Shih, E. Mena, J. W. Kim, J. C. Park, A. Alikhani, Y. Y. McKinney, J. Weaver, B. Turkbey, H. L. Parnes, L. V. Wood, R. A. Madan, J. L. Gulley, W. L. Dahut, K. A. Kurdziel and P. L. Choyke, J. Nucl. Med., 2016, 57, 886–892 CrossRef CAS PubMed.
- M. R. Dweck, C. Jones, N. V. Joshi, A. M. Fletcher, H. Richardson, A. White, M. Marsden, R. Pessotto, J. C. Clark, W. A. Wallace, D. M. Salter, G. McKillop, E. J. R. Van Beek, N. A. Boon, J. H. F. Rudd and D. E. Newby, Circulation, 2012, 125, 76–86 CrossRef CAS PubMed.
- C. Portulano, M. Paroder-Belenitsky and N. Carrasco, Endocr. Rev., 2014, 35, 106–149 CrossRef CAS PubMed.
- T. A. R. Stallons, A. Saidi, I. Tworowska, E. S. Delpassand and J. J. Torgue, Mol. Cancer Ther., 2019, 18, 1012–1021 CrossRef CAS PubMed.
- I. Palma-Lara, M. Martínez-Castillo, J. C. Quintana-Pérez, M. G. Arellano-Mendoza, F. Tamay-Cach, O. L. Valenzuela-Limón, E. A. García-Montalvo and A. Hernández-Zavala, Regul. Toxicol. Pharmacol., 2020, 110, 104539 CrossRef CAS PubMed.
- C. Niu, H. Yan, T. Yu, H. P. Sun, J. X. Liu, L. Xiu-Song, W. Wu, F. Q. Zhang, Y. Chen, L. Zhou, J. M. Li, X. Y. Zeng, R. R. O. Yang, M. M. Yuan, M. Y. Ren, F. Y. Gu, Q. Cao, B. W. Gu, X. Y. Su, G. Q. Chen, S. M. Xiong, T. D. Zhang, S. Waxman, Z. Y. Wang, Z. Chen, J. Hu, Z. X. Shen and S. J. Chen, Blood, 1999, 94, 3315–3324 CrossRef CAS PubMed.
- M. Jennewein, M. A. Lewis, D. Zhao, E. Tsyganov, N. Slavine, J. He, L. Watkins, V. D. Kodibagkar, S. O’Kelly, P. Kulkarni, P. P. Antich, A. Hermanne, F. Rösch, R. P. Mason and P. E. Thorpe, Clin. Cancer Res., 2008, 14, 1377–1385 CrossRef CAS PubMed.
- P. A. Ellison, T. E. Barnhart, F. Chen, H. Hong, Y. Zhang, C. P. Theuer, W. Cai, R. J. Nickles and O. T. Dejesus, Bioconjugate Chem., 2016, 27, 179–188 CrossRef CAS PubMed.
- S. H. Al-Kouraishi and G. G. J. Boswell, Int. J. Appl. Radiat. Isot., 1978, 29, 607–609 CrossRef CAS.
- M. Jennewein, S. M. Qaim, P. V. Kulkarni, R. P. Mason, A. Hermanne and F. Rösch, Radiochim. Acta, 2005, 93, 579–583 CrossRef CAS.
|
This journal is © The Royal Society of Chemistry 2022 |
Click here to see how this site uses Cookies. View our privacy policy here.