DOI:
10.1039/D1CE01366A
(Communication)
CrystEngComm, 2022,
24, 52-56
Shock wave induced phase transition from crystalline to the amorphous state of lead nitrate crystals†
Received
9th October 2021
, Accepted 16th November 2021
First published on 17th November 2021
Abstract
The promising prospects arising out of the phase transitions of materials under shock conditions are attracting great attention in emerging fields at the frontier of structural science. In this communication, a dynamic shock wave induced phase transition from crystalline to the amorphous state of lead nitrate crystals is reported and the discussion is based on the results obtained from diffraction, spectroscopic and microscopic techniques.
Introduction
Shock waves genarated from tabletop shock tubes have profound potential to unlock the properties of a wide range of feasible materials of all kinds ranging from nano to bulk scales which could possess peculiar properties to be unearthed such that identifying phase change materials is on the surge due to the impending possible scope for a variety of applications. Shock wave impact analysis on materials has the prospective potential of rekindling innovation for the state-of-the-art modern technology which maneuvers the researchers to take up the task of unearthing the hidden wonders of functional materials as there is a lot of scope for the enrichment of multidimensional materials science research.1–3 In recent years, shock wave-induced endeavors have been witnessed which show the pathway to find materials that can undergo reversible phase transformation such that they are found to be suitable for a wide range of applications such as molecular switching, dielectric switching, magnetic sensors, phase shifters, resistive memories, etc.4–8 However, reports on dynamic shock wave-driven assessment of crystallographic phase transitions revealing high shock-resistant materials are much scarcer than static high-pressure studies. As known from the literature, reports on laser shock-induced phase transition from crystalline to the amorphous state are very few and these involve germanium,9 ZIF-8 MOFs,10 silicon carbide,11 boron carbide,12 and mullite.12 The amorphous to the crystalline phase transition of multi-wall carbon nanotubes has been demonstrated by the impulsion of dynamic shock waves.14 Furthermore, crystalline to crystalline phase transitions are exhibited in materials such as titanium oxide15 and zirconium oxide16 by dynamic shock waves, while reversible crystallographic phase transitions,4,17,18 reversible magnetic phase transitions and molecular5,7 phase transitions have also been reported very recently. But among these possible crystallographic phase transitions, crystalline to amorphous transitions and vice versa are still not explored much due to lack of data so systematic analysis of such phase transitions is of prime necessity in order to have a better understating of dynamic shock wave physics in condensed matter. Moreover, there are a number of important questions being raised on available literature reports.9–13 Why and how does an ordered crystal become a disordered crystal under shock conditions and vice versa? Moreover, why do some of the materials undergo polymorphic phase transitions under shock conditions? The answers to these questions lie in the secret of how one might design functional materials by controlling the disordered crystalline nature under extreme conditions. A literature survey reveals that there are no reports available on the ionic crystals of a family of nitrates under shock conditions. Lead nitrate Pb(NO3)2 single crystals have been established as potential candidates for ferroelectric applications and several researchers have examined their physical and chemical properties over the years and found several interesting results with respect to the temperature which influences changes in the internal free rotation of the nitrate groups within the crystal lattice.19–22 Research on the shock wave impact of isomorphic nitrates such as lead, barium, and strontium may provide a fruitful understanding of their structural concepts which may provide a direct path to the discovery of ferroelectric activity of novel materials associated with shock conditions.
In this communication, we report the direct phase transition of lead nitrate crystals from crystalline to the amorphous state via an unknown high-pressure phase under dynamic shock conditions which is then discussed on the basis of the results obtained from X-ray diffraction, Raman spectroscopy and microscopy techniques.
Experimental section
For the present experiment, the required optically transparent crystals of Pb(NO3)2 have been grown by a slow evaporation technique at room temperature and harvested after 25 days. The comprehensive details of the crystal growth of the title crystal and a shock wave loading (Fig. S1†) methodology are provided in the ESI† data. In the present framework, shock waves of Mach number 1.7 have been utilized with the transient pressure of 1.0 MPa. Four test crystals of equal dimensions (5 × 5 × 1mm3) have been identified such that one crystal has been utilized as the control crystal and the remaining three crystals have been utilized for the shock wave impact study. Subsequently, one, two and three shock pulses have been loaded on the respective crystals in sequential order.
Results and discussion
X-ray diffraction analysis
X-ray diffraction (Rigaku – SmartLab X-ray diffractometer) has been adopted to characterize the structural characteristics of the control and shocked crystals and the obtained XRD profiles are presented in Fig. 1 wherein the control crystal has three diffraction peaks at 19.81, 39.86 and 61.54°, respectively, and the corresponding diffraction planes are (111), (222) and (511). Moreover, the observed diffraction peak positions are well-matched with the simulated structure of the title crystal with the Pa
space group (COD = 5
910
007). Under shock conditions, interesting results have been observed and the XRD profiles of the title crystal with respect to the number of shock pulses are presented in Fig. 1a. Under the first shock condition, the complete disappearance of the control crystal's diffraction peaks indexed to (111), (222) and (511) is observed whereas a new diffraction peak appears at 72.36° and this diffraction angle position belongs to the (600) plane with the space lattice symmetry of Pa
. The generation of the new diffraction peak is due to the dynamic re-orientation of the oxyanion nitrate groups since, in the case of the metal carbonate group materials, the materials have free internal rotation of the nitrate groups and stable metal cations. The research interest in such materials at high-pressure and high-temperature is still growing due to the creation of new configuration materials and the availability of new theories on the phase transition of nitrate group materials of which KNO3 crystals are the most extensively studied materials. As per the crystallographic point of view, each Pb atom is connected to the oxygen atoms of different planar anionic NO3 units so as to build the cuboctahedron and the anions are bound to the lead cation by the ionic bond. In the case of the (111) plane of the title crystal, of the two nitrate groups, one lies above and the other lies below the lead atoms at the same distance. The plane orientations of the (111) and (600) planes are presented in Fig. 1b. Under shock conditions, significant migration of the cationic atoms and rotational disorder of the NO3 units occur because of the impact of shock waves and hence the re-orientational effect occur and similar results have been observed for the SO4 and PO4 anionic materials.4,23 Note that, under the first shock condition, no crystallographic phase transition is observed. Under the second shock condition, a new strong diffraction peak appears again at 43.36° and astonishingly, this diffraction peak does not belong to the space group Pa
of the title crystal and the corresponding XRD pattern is presented in Fig. 1a. Moreover, to date, there are no high-pressure or high-temperature experimental data that have been reported for the title crystal. The obtained XRD results could be considered as a high-pressure phase such that this finding would be a beacon for the crystallographic researchers so as to solve this structure of the new phase of lead nitrate crystal. So far, there is no definitive agreement among researchers on the number of known crystalline phases of these types of salts and there is no disagreement either regarding the existence or non-existence of new crystalline phases when working under certain external conditions such as pressure, temperature and shock waves. On this note, the shock wave-induced high-pressure crystallographic phase of the title crystal could be a fruitful subject to the researchers as this may lead to the discovery of the ferroelectric activity of the title crystal. Moreover, the dynamic migration of the cationic atoms and the rotational disorder of the free NO3 units have a major contribution to the formation of such a high-pressure phase under shock conditions.24,25
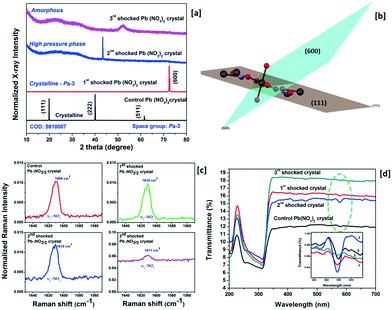 |
| Fig. 1 (a) XRD patterns of the control and shocked crystals; (b) configurations of the (111) and (600) planes; (c) υ2-Raman spectra of the control and shocked crystals; (d) optical transmittance spectra of the control and shocked crystals (inset: normalized transmittance data showing the disappearance of the absorption band under the 3rd shock condition). | |
Under the 3rd shock conditions, there is no signature of the diffraction planes, not even low-intensity peaks so that it could be considered an amorphous state in which a complete phase transition from crystalline to the amorphous state has occurred making it the first observation, to date, made on the title sample in the amorphous state. Under the 3rd shock conditions, the rotational disorder of the NO3 units, the positional disorder of the cations and non-planarity of the NO3 ions significantly increase and hence the disappearance of the crystalline state has been observed wherein the resultant test crystal turns into an amorphous state.26–29 Under shock conditions, no physical breakage of the test crystal has been noticed. Another interesting behaviour is noted as shown in the XRD profiles of the test crystal with respect to the number of shock pulses (Fig. 1a) in which the baseline of the control crystal's XRD pattern is a straight line indicating the good crystalline nature; while increasing the number of shock pulses (1, 2 and 3), the line width considerably increases with respect to the number of shock pulses such that it authenticates the shock wave induced structural disorder (defects) with respect to the ordered NO3 groups in the lattice and the space group allows the formation of a thermal population of upper vibrational states associated with anharmonicity in the crystal and the corresponding figure is presented in ESI† S2. A Raman spectroscopy study for the control and shocked crystals has been performed to better understand the rotational disorder of the NO3 units and the recorded Raman spectra of the control and shocked crystals are presented in ESI† S3 wherein the observed Raman bands are found to be in good agreement with previous reports.30,31 The important characteristic Raman band represented by the υ2-NO3 Raman region is shown in Fig. 1c and the υ1-NO3 Raman region is presented in ESI† S4. Numerous researchers have reported the Raman spectral characteristic behavior and the respective signature of the occurrence of the disorder of NO3 units in the unit cells which have been clearly discussed for a similar group of crystals.32–34 In general, broadening of NO3 Raman lines gives disordered structures of the crystals as observed in this case. As seen in Fig. 1c, the υ2-NO3 Raman band has a strong and high intensity that is located at 1609 cm−1 for the control crystal and the Raman position and Raman shapes remain the same for the 1st and 2nd shock conditions, in which, under the 2nd shock condition, the normalized intensity of the υ2-NO3 Raman band considerably increases such that this may be the possible indication of the formation of a new phase in the title crystal. But in the case of the 3rd shock condition, the normalized intensity of the υ2-NO3 Raman band is almost a flat line with respect to the baseline and this is the definitive proof for the formation of the amorphous state of lead nitrate. Similarly, in the case of υ1-NO3, the Raman band at 1024 cm−1 has completely disappeared. Note that the nitrate ions are located at the C1 site symmetry possessing the crystalline state of the Pa
space group. Moreover, under the 3rd shock condition, the positional coordinates of the nitrates have moved off the C1 site symmetry and induced the lattice disorder of the unit cell and as a result, we have observed the amorphous state at 3rd shock condition.30,31,34 In addition to that, the rotational moment of inertia of the NO3 group is expected to change with respect to the number of shock pulses. Generally, the rotational inertia of NO3 units in molten salts increases with respect to temperature due to the increase in the rotational kinetic energy.35 On the same footing, from the XRD and Raman measurements, it is clear that the degree of rotational disorder of NO3 units has increased with respect to the number of shock pulses such that the amorphous state is attained under the 3rd shock condition because of a significant enhancement of the rotational disorder of NO3 in the crystal lattice. Based on a previous report,35 the amorphous state of the PbNO3 sample has higher rotational inertia of NO3 than that of the high-pressure phase and ambient phase. The obtained optical transmittance spectra of the control and shocked crystals with respect to the number of shock pulses over the wavelength region between 200 and 700 nm using a UV-DRS Shimadzu UV-3600 plus spectrometer are presented in Fig. 1d. The nitrate ion's primary characteristic absorption band is located at 228 nm and the observed wavelength region is found to be well-matched with previous reports.36,37 In addition to that, for the control crystal, one more absorption band of NO3 units is located at 577 nm. Under shock conditions, there is no major change that has been noticed with respect to the phase in the primary NO3 absorption band region. But the secondary NO3 absorption band shows a few changes under the shock conditions. Remarkably, the secondary NO3 absorption band intensity reduces considerably under the 3rd shock condition and may be the possible signature of the formation of the amorphous state as reflected in optical absorption spectroscopy. In the case of the amorphous state, a few of the absorption energy bands might have collapsed which could be the reflection in the form of the disappearance of the molecular absorption bands and similar results have been reported for the crystalline and amorphous states of antimony trisulphide.38,39
Morphological studies
Field emission scanning electron microscopy (FE-SEM) has been adopted to evaluate the microstructure and surface morphology of the title crystal under shock conditions and the obtained micrographs are presented in Fig. 2. As seen in Fig. 2a, the control crystal exhibits ferroelectric surface grains which may be due to the growth characteristic of the title crystal possessing a cubic crystal system. Under the 1st shock condition, the surface grains completely turn into a rectangular plate-like morphology which may be due to the re-orientation of NO3 units and the corresponding micrograph is presented in Fig. 2b and the results are well corroborated with the XRD results. Note that the shear deformation and fragmentation are on a very minimum level under the first shock condition. Under the 2nd shock condition, there is no clear shape that could be noticed on the top surface of the crystal which may be due to the formation of the high-pressure phase of the crystal and the corresponding micrograph is shown in Fig. 2c.
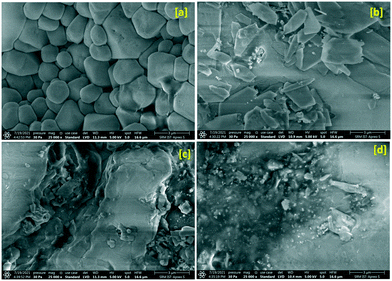 |
| Fig. 2 FE-SEM images of the control and shocked crystals: (a) the control, (b) 1st shocked, (c) 2nd shocked and (d) 3rd shocked crystals. | |
The shapeless surface morphology and agglomeration morphology clearly show a considerable increment of the shear deformation and fragmentation along with the rotational disorder of the NO3 units. Interestingly, under the 3rd shock condition, a complete collapse of the well-shaped surface morphology is observed which may be due to the massive increment of the shear deformation and fragmentation of the crystalline blocks and similar results have been observed for ZIF-8 crystals.10
Conclusions
In the present work, the crystallographic phase stability of lead nitrate crystals under dynamic shock wave loaded conditions at Mach number 2.2 has been investigated and the phase transition is observed from crystalline to the amorphous state via an unknown high-pressure phase and the shock phase profile with respect to the number of shock pulses is Pa
–Pa
– high-pressure phase – amorphous for the 0, 1, 2 and 3rd shocked conditions, respectively. The observed re-orientational changes of the crystallographic planes and the amorphous state observed for the test samples are due to the occurrence of rotational disorder and the formation of non-planar NO3 groups under shock conditions. XRD profiles provide evidence for the formation of the amorphous state under the 3rd shock condition and the results obtained from Raman spectroscopy, UV-DRS and SEM are in excellent agreement with the XRD results. Note that this could be the first report, to date, for the formation of an unknown high-pressure phase for lead nitrate crystals under shock conditions and such kinds of new high-pressure phase and amorphous structured materials may contribute towards ferroelectric applications.
Author contributions
A. Sivakumar: validation, formal analysis, resources and writing – original draft. P. Eniya: sample preparation. S. Sahaya Jude Dhas: review & editing. Raju Suresh Kumar: resources. Abdulrahman I. Almansour: formal analysis. Kundan Sivashanmugan: formal analysis. J. Kalyana Sundar: formal analysis. S. A. Martin Britto Dhas: supervision, writing – review & editing.
Conflicts of interest
The authors declare that they have no conflict of interest.
Acknowledgements
The authors thank the Department of Science and Technology (DST), India for funding this research through the DST-FIST programme (SR/FST/College-2017/130 (c)).
Notes and references
- Z. Su and W. L. Shaw,
et al.
, J. Am. Chem. Soc., 2017, 139, 4619–4622 CrossRef CAS PubMed.
- J. M. Winey, K. Zimmerman, Z. A. Dreger and Y. M. Gupta, J. Phys. Chem. A, 2020, 124, 6521–6527 CrossRef CAS PubMed.
- T. De Rességuier, O. O. Kurakevych, A. Chabot, J. P. Petitet and V. L. Solozhenko, J. Appl. Phys., 2017, 108, 083522 CrossRef.
- A. Sivakumar and S. Reena Devi,
et al.
, Cryst. Growth Des., 2020, 20, 7111–7119 CrossRef CAS.
- A. Sivakumar and S. Soundarya,
et al.
, J. Phys. Chem. C, 2020, 124, 10755–10763 CrossRef CAS.
- V. Mowlika and A. Sivakumar,
et al.
, J. Nanostruct. Chem., 2020, 10, 203–209 CrossRef CAS.
- A. Rita and A. Sivakumar,
et al.
, J. Mater. Sci.: Mater. Electron., 2020, 31, 20360–20367 CrossRef.
- J. M. Winey, K. Zimmerman, Z. A. Dreger and Y. M. Gupta, J. Phys. Chem. A, 2020, 124, 6521–6527 CrossRef CAS PubMed.
- S. Zhao, B. Kad, C. E. Wehrenberg, B. A. Remington, E. N. Hahn, K. L. More and M. A. Meyers, Proc. Natl. Acad. Sci. U. S. A., 2017, 114, 9791–9796 CrossRef CAS PubMed.
- X. Zhou, Y.-R. Miao, W. L. Shaw, K. S. Suslick and D. D. Dlott, J. Am. Chem. Soc., 2019, 141, 2220–2223 CrossRef CAS PubMed.
- S. Zhao and R. Flanagan,
et al.
, Acta Mater., 2018, 158, 206–213 CrossRef CAS.
- S. Zhao, B. Kad, B. A. Remington, J. C. LaSalvia, C. E. Wehrenberg, K. D. Behler and M. A. Meyers, Proc. Natl. Acad. Sci. U. S. A., 2016, 113, 12088–12093 CrossRef CAS PubMed.
- W. Braue, B. Hildmann, H. Schneider and U. Hornemann, J. Eur. Ceram. Soc., 2009, 29, 3135–3146 CrossRef CAS.
- A. Sivakumar and S. S. J. Dhas,
et al.
, Cryst. Growth Des., 2021, 21, 1617–1624 CrossRef CAS.
- S. Kalaiarasi, A. Sivakumar, S. A. M. B. Dhas and M. Jose, Mater. Lett., 2018, 219, 72–75 CrossRef CAS.
- V. Jayaram and K. P. J. Reddy, Adv. Mater. Lett., 2016, 7, 100–150 Search PubMed.
- A. Sivakumar and S. S. J. Dhas,
et al.
, New J. Chem., 2021, 45, 16529 RSC.
- A. Sivakumara and S. S. Jude,
et al.
, CrystEngComm, 2021, 23, 7044 RSC.
- G. K. Bichile and R. G. Kulkarni, Acta Crystallogr., Sect. A: Cryst. Phys., Diffr., Theor. Gen. Crystallogr., 1975, 31, 446 CrossRef.
- H. Isoda and R. Kawashima, J. Phys. Chem. Solids, 2007, 68, 561–563 CrossRef CAS.
- F. Michard, F. Plicque and A. Zarembowitch, J. Appl. Phys., 1973, 44, 572 CrossRef CAS.
- K. Sai Babu and T. Chiranjivi, Solid State Ionics, 1982, 6, 155–157 CrossRef CAS.
- A. Sivakumar, S. S. J. Dhas, S. Balachandar and S. A. M. B. Dhas, J. Electron. Mater., 2019, 48, 7868–7873 CrossRef CAS.
- M. K. Teng, M. Balkanski and J. F. Mourey, Solid State Commun., 1971, 9, 465–469 CrossRef.
- T. G. Worlton, D. L. Decker, J. D. Jorgensen and B. Kleb, Phys. B, 1986, 136, 503–506 CrossRef CAS.
- A. Lunden, Z. Naturforsch., 1995, 50, 1067–1076 CrossRef CAS.
- H. Stephen, Rep. Prog. Phys., 2004, 67, 1233–1314 CrossRef.
- K. Raul, L. Nilsson, N. Hessel Andersen, A. Lundent and O. T. John, J. Phys.: Condens. Matter, 1992, 4, 1925–1933 CrossRef.
- E. Cazzanelli and R. Frech, Raman spectra of 7Li2SO4 and 6Li2SO4, J. Chem. Phys., 1983, 79, 2615 CrossRef CAS.
- H. Isoda, A. Sakal and R. Kawashima, J. Phys. Soc. Jpn., 2007, 76, 065001 CrossRef.
- M. H. Brooker, J. Solid State Chem., 1979, 28, 29–39 CrossRef CAS.
- R. Murugan, P. Jane Huang, A. Ghule and H. Chang, Thermochim. Acta, 2000, 346, 83–90 CrossRef CAS.
- M. H. Brwker, J. Phys. Chem. Solids, 1978, 39, 657–667 CrossRef.
- J. W. Singer, A. Özgür Yazaydin, R. James Kirkpatrick and G. M. Bowers, Chem. Mater., 2012, 24, 1828–1836 CrossRef CAS.
- M. Hafez, I. S. Yahia and S. Taha, Acta Phys. Pol., A, 2015, 127, 734–740 CrossRef.
- K. S. Krishnan and A. C. Guha, Proc. Indian Natl. Sci. Acad., 1934, 1, 242–249 Search PubMed.
- C. Ghosh and B. P. Varma, Thin Solid Films, 1979, 60, 61–65 CrossRef CAS.
- C. Ghosh and B. P. Varma, Solid State Commun., 1979, 31, 683–686 CrossRef CAS.
- T. Kota and T. Takenaka, Mol. Phys., 1985, 54, 1393 CrossRef.
Footnote |
† Electronic supplementary information (ESI) available. See DOI: 10.1039/d1ce01366a |
|
This journal is © The Royal Society of Chemistry 2022 |
Click here to see how this site uses Cookies. View our privacy policy here.