DOI:
10.1039/D2DT01873J
(Paper)
Dalton Trans., 2022,
51, 14228-14242
Single component white-OLEDs derived from tris(β-diketonato) europium(III) complexes bearing the large bite angle N^N 2-(4-thiazolyl)benzimidazole ligand†
Received
13th June 2022
, Accepted 21st August 2022
First published on 30th August 2022
Abstract
Two new organo-europium complexes (OEuCs) [Eu(tfac)3(TB-Im)] (Eu1) [Eu(hfac)3(TB-Im)] (Eu2) incorporating fluorinated (hexafluoroacetylacetone; Hhfaa) or hemi-fluorinated (trifluoroacetylacetone; Htfac) β-diketones together with the large bite angle N^N ligand (2-(4-thiazolyl)benzimidazole; TB-Im) have been synthesized and characterized. The structure of the complexes has been established by single crystal X-ray diffraction (SC-XRD) analysis and shows that the coordination sphere is composed of a EuO6N2 core (octacoordinated). Continuous shape measures (CShMs) revealed that the geometry around Eu(III) is trigonal dodecahedral with approximate D2d-symmetry. Efficient red emission is observed for both the complexes in solution with a fairly large photoluminescence quantum yield (PLQY (QLEu) = 39.00–47.00%). Furthermore, by utilizing the experimental photoluminescence (PL) data and theoretical modelling employing density functional theory (DFT) in conjunction with LUMPAC, energy transfer (ET) and back energy transfer rates were calculated, and an ET mechanism for the sensitized PL is proposed and discussed in detail. Finally, the complexes were used as an emitting layer (EML) to fabricate 20 organic light emitting diodes (OLEDs) by varying the doping concentration. Interestingly, both the complex-based OLEDs at 4 wt% doping concentration display white electroluminescence (EL) with the brightness (B) = 100.5–364.1 cd m−2 at very low turn-on voltage (Vturn-on) = 3.9–4.6 V. The overall electroluminescence performance of Eu1 and Eu2 is higher than that of the reported europium based single component white-OLEDs.
1. Introduction
Research into the design and development of coordination complexes capable of panchromatic emission either in their molecular forms or in devices has received considerable attention.1 This is because of their wide application in full-colour flat-panel displays and solid-state lighting for the benefit of energy conservation.2 One of the promising classes of complexes for further development is the class of efficient organo-europium complexes (OEuCs) because of their exemplary photophysical properties leading to many fascinating applications such as sensors3 and thermometers,4 and in anti-counterfeiting applications.5 To develop efficient OEuCs, the organic ligand(s) acting either as the primary antenna or as the ancillary ligands must have strong light absorption capabilities between 250 and 450 nm with a compatible triplet state (3ππ*) leading to sufficiently separated 3ππ* and Eu(III) emitting levels.6 This allows efficient harvesting of the absorbed energy by the organic ligand(s) through the well-known antenna effect.7 Besides, the intrinsic monochromatic red emission due to the electric-dipole (ED) 5D0 → 7F2 transition of Eu(III)8 between 608 nm and 620 nm makes these complexes potential candidates as the red component9 to fabricate tricolour RGB-based white organic light emitting diodes (white-OLEDs) given that the OEuCs exhibit efficient PL properties.
As simple as it sounds, the generation of white light i.e., panchromatic emission in any phase (crystal/solution/film) remains challenging if a three-component approach is used and has some serious drawbacks e.g., colour impurity as the device ages, manufacturing cost as well as labour to fabricate them, thereby impeding their real-life and industrial applications.1b,10 To overcome these issues, the development of energy-efficient white-OLEDs from a single molecular platform could provide an elegant solution.1b Among the different strategies, an appropriate way to achieve white light emission from a single compound is the generation of blue and reddish-orange emissions concomitantly.10 Interestingly, OEuCs could be employed as EMLs to fabricate white-OLEDs particularly because they display three emission transitions in the reddish-orange region also because they have microsecond to millisecond excited state lifetimes. It is well known that as the current density increases, the longer excited lifetime decays non-radiatively due to triplet–triplet annihilation11 leading to emissions in the region between 400 and 500 nm.
Keeping this idea in mind, in the present work, we synthesized two new OEuCs complexes (Chart 1) by employing hemi-fluorinated (Htfac) and fluorinated (Hhfac) acetylacetones as the primary antenna ligands in conjunction with TB-Im as an ancillary ligand. It is well established that both the β-diketones act as efficient antenna ligands to generate highly luminescent OEuCs,1b,d,12 which is attributed to the well-placed 3ππ* (3ππ* = 22
720 cm−1 for tfac and 21
930 cm−1 for hfac) transitions fulfilling Latva's empirical rule.6b The bidentate ancillary TB-Im ligand was chosen because of the asymmetrical N^N-chelating coordination mode via the five-membered imidazolyl ring fused with phenyl ring (benzoimidazole ring C–N = 1.393 Å) and five-membered thiazolyl ring (C–N = 1.295 Å). As noted by us and others, large bite angle coordinating ligands have a profound effect in distorting the coordination geometry around the Ln(IIII) centre, which is beneficial for enhancing the PL properties.9a,c,13 Besides, the NH proton of the imidazolyl ring is often engaged in the formation of intermolecular hydrogen bonds leading to supramolecular self-assembly, that result in fascinating structural arrangements in the solid-state.1a We now present the details of the synthesis of Eu1 and Eu2, (Chart 1), their structural characterisation, and their photophysical properties. The results are supported by theoretical studies and an ET mechanism of the sensitized emission for the OEuCs is proposed. Finally, we have employed Eu1 and Eu2 as an emitter to fabricate single- and double-EML OLEDs.
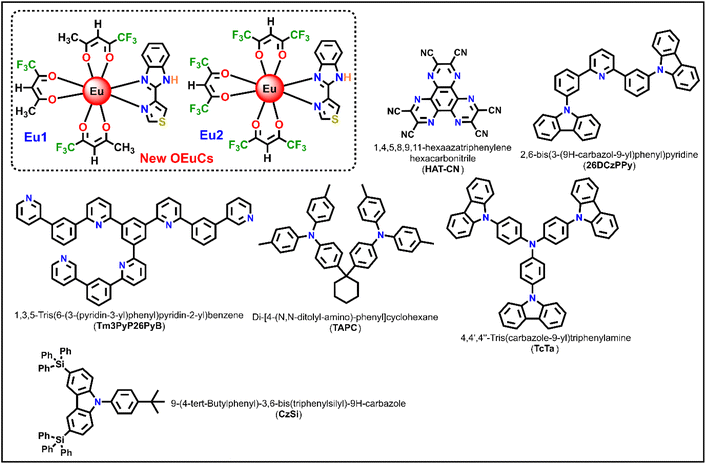 |
| Chart 1 Chemical structures of new OEuCs and organic compounds employed in the OLEDs fabrication. | |
2. Experimental
2.1. Synthesis of the complexes
Eu(III) chloride was purchased from Strem Chemicals, Inc. Htfac, Hhfac and TB-Im were obtained from Tokyo Chemical Industry (TCI) and were used as received. Solvents were pre-dried and distilled before use according to standard procedures. All organic compounds employed in OLED fabrication were procured from commercial sources and used without further purification unless otherwise specified (Chart 1). Elemental analysis was performed on an Euro EA – CHN in the Department of Chemistry, Sultan Qaboos University. Attenuated total-reflectance (ATR) infrared (IR) spectra were recorded on pure samples on diamond using a Cary 630 F T-IR spectrometer. Mass spectra were obtained using LCMS-8040, Shimazdu-Japan coupled to a triple quadruple tandem mass spectrometer equipped with electrospray ionization (ESI). The thermal stability of the complexes was determined by thermogravimetric analysis (TGA) and differential thermogravimetric analysis (DTA) in the temperature range between 50 and 700 °C under a dinitrogen (N2) atmosphere and recorded on TA instrument model SDTQ600.
2.1.1. [Eu(tfac)3(TB-Im)] (Eu1).
Eu-1 was synthesized by reacting equimolar quantities of [Eu(tfac)3(H2O)2]1b (0.5 g; 0.771 mmol) and TB-Im (0.155 g; 0.771 mmol) in 1
:
1 mixture of dichloromethane (DCM) and ethanol (EtOH). The reaction mixture was stirred overnight at room temperature and left for slow solvent evaporation. After a week crystals suitable for single-crystal X-ray analysis were obtained. Colour: white; yield (70%); microanalysis calculated for C25H19EuF9N3O6S: C, 36.96; H, 2.36; N, 5.17; found: C, 36.98; H, 2.34; N, 5.16; FTIR (solid; cm−1)- ν(N–H) 3251 cm−1; ν(C
O)st 1617 cm−1; ν(C
N)st 1524 cm−1; out-of plane asymmetric ν(C–F)st 1177 cm−1; in-plane ν(C–H)bend. 1124 cm−1, (Fig. S1, ESI†); ESI-MS (m/z) = 812.1 [M + H] (Fig. S2, ESI†) melting temperature (Tm) = 228.9 °C decomposition temperature (Td) with 5% weight loss = 241 °C.
2.1.2. [Eu(hfac)3(TB-Im)] (Eu2).
Eu2 was synthesized using a one-pot method reported earlier for the synthesis of related complexes.1d Colour: white; yield (68%); microanalysis calculated for C25H10EuF18N3O6S: C, 30.82; H, 1.03; N, 4.31; found: C, 30.84; H, 0.99; N, 4.29; FT-IR (solid; cm−1)- ν(N–H) 3297 cm−1; ν(C–O)st 1642 cm−1; ν(C
N)st 1531 cm−1; out-of plane asymmetric ν(C–F)st 1194 cm−1; in-plane ν(C–H)bend. 1137 cm−1; (Fig. S3, ESI†); ESI-MS (m/z) = 1013.20 [M + K − 2H] (Fig. S4, ESI†); Tm = 202 °C, Td with 5% weight loss = 239 °C.
2.2. Single crystal X-ray structure determination
Single crystals of Eu1 and Eu2 suitable for single-crystal X-ray analysis were grown by the slow solvent (EtOH) evaporation method. The structure determination was performed at room temperature on a Stoe IPS II diffractometer using monochromatic Mo-Kα radiation (λ = 0.71073 Å). A multi-scan absorption correction was applied. The data reduction, including an empirical absorption correction using spherical harmonics, was implemented in LANA. The crystal structure was solved by direct methods using the online version of WinGX14 and then refined by full-matrix least-squares (SHELXL-2018) on F2.15 The non-hydrogen atoms were refined anisotropically. All of the hydrogen atoms were positioned geometrically in idealized positions and refined with the riding model approximation, with Uiso(H) = 1.2Ueq(C). The displacement ellipsoids particularly on the fluorine atoms of the CF3 groups were elongated because of disorder however they could be modelled appropriately using a single atom position for each fluorine atom. Crystal data and structure refinement for europium complexes are detailed in Table S2, ESI.† The molecular graphics were produced using the program MERCURY from the CSD package.16
2.3. Spectroscopic measurements and OLED fabrication process
Spectroscopic measurements of the complexes that include optical absorption, excitation, emission spectra, decay profiles and absolute PLQY values were performed at room temperature; the methodology for the measurements have been reported previously.9c Optical absorption spectra were obtained using Varian Cary 5000 UV-Visible-NIR spectrophotometer while excitation, emission spectra and decay profiles were recorded on an Edinburgh FS5 fluorimeter. The absolute PLQY were determined using a calibrated integrating sphere on a C-9920-02 from the Hamamatsu Photonic instrument. Theoretical methodology details (section 1, ESI†) involving the calculation of ground-state geometry, ET rates, radiative emission rate (ARad) and theoretical PLQY are detailed in the ESI.† Important experimental photophysical parameters were calculated by the following equations as detailed in our previous reports:1d,17 | 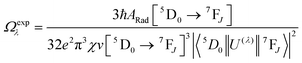 | (1) |
| 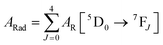 | (2) |
|  | (3) |
| 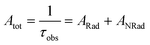 | (4) |
|  | (5) |
| 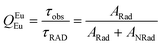 | (6) |
|  | (7) |
ITO coated glass with the sheet resistance of 10 Ω sq−1 was used as the anode substrate. Prior to film deposition, patterned ITO substrates were cleaned with detergent, rinsed in de-ionized water, and finally dried in an oven. All organic layers were deposited at a rate of 0.1 nm s−1 under a high vacuum (≤3.0 × 10−5 Pa). The doped EMLs were prepared by co-evaporating dopant and host material from two sources, and the doping concentration was modulated by controlling the evaporation rate of the dopant. LiF and Al were deposited in another vacuum chamber (≤8.0 × 10−5 Pa) at rates of 0.01 and 1.0 nm s−1, respectively, without being exposed to the atmosphere. The thicknesses of these deposited layers and the evaporation rate of individual materials were monitored in vacuum with quartz crystal monitors. A shadow mask was used to define the cathode and make eight emitting dots with an active area of 9 mm2 on each substrate. Current density (J)–brightness (B)–voltage (V) characteristics were measured by using a programmable brightness light distribution characteristics measurement system C9920-11 from the Hamamatsu Photonic instrument. PL and EL spectra were measured with a calibrated Hitachi F-7000 fluorescence spectrophotometer and an Ocean Optics spectrophotometer.
3. Results and discussion
3.1. Synthesis, characterization and X-ray diffraction studies
The new complexes were synthesized by the method reported earlier1b,d and characterized by elemental analysis, mass spectrometry and FT-IR spectroscopy. The results suggested the formation of the complexes with the formulae [Eu(tfac)3(TB-Im)] (Eu1) [Eu(hfac)3(TB-Im)] (Eu2). These results were confirmed by the single-crystal X-ray diffraction studies. The X-ray crystallographic data for Eu1 and Eu2 are given in Table S2, ESI.† The complex Eu1 crystallizes in the triclinic space group P
while Eu2 in monoclinic space group P21/n. The asymmetric unit in both cases consists of a single mononuclear complex in which the Eu(III) ion is eight coordinate comprising six oxygen (O) atoms from the primary β-diketone ligands and two nitrogen (N) atoms of ancillary ligands i.e., N2O6, Fig. 1. The bond distances (Table 1) are comparable to the analogous OEuCs reported by us.1d,9b The Eu–N bond distances [(2.560(3) Å)avg. for Eu1 and (2.554(4) Å)avg. for Eu2] are, in both cases, longer than Eu–O bond distances [(2.365(3) Å)avg for Eu1 and (2.382(3) Å)avg. for Eu2] (Table 1). The coordination geometry and assignment of symmetry around the Eu(III) centres of the complexes were determined by the SHAPE software package which calculates continuous shape measures (CShMs) of a set of atomic positions relative to the vertices of ideal reference polyhedra.18 The EuN2O6 coordination polyhedron can be assigned as a distorted triangular dodecahedron, with idealized D2d symmetry around the metal centre (Fig. 1c). Comparison between the two structures revealed that the distortion is similar with CShM values 0.629 and 0.695 for Eu1 and Eu2 (Table S3, ESI†), respectively, despite the primary antenna ligand (hfac) in the latter being symmetrical.
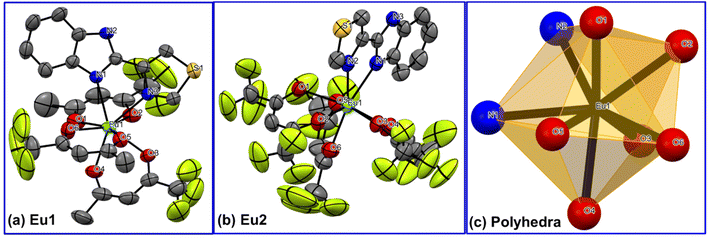 |
| Fig. 1 Single-crystal X-ray structures of (a) Eu1 and (b) Eu2. The displacement ellipsoids have been drawn with 50% probability (c) trigonal dodecahedral coordination polyhedron. Hydrogen atoms are omitted for clarity. | |
Table 1 Selected bond lengths [Å] and angles [°] for Eu1 and Eu2
Eu1
|
Eu(1)–O(6) |
2.353(3) |
Eu(1)–O(3) |
2.386(3) |
Eu(1)–O(4) |
2.354(3) |
Eu(1)–O(1) |
2.392(3) |
Eu(1)–O(5) |
2.357(3) |
Eu(1)–N(1) |
2.542(3) |
Eu(1)–O(2) |
2.385(3) |
Eu(1)–N(3) |
2.606(3) |
O(6)–Eu(1)–O(4) |
76.76(14) |
O(3)–Eu(1)–O(1) |
124.98(13) |
O(6)–Eu(1)–O(5) |
72.10(12) |
O(6)–Eu(1)–N(1) |
80.85(12) |
O(4)–Eu(1)–O(5) |
90.55(12) |
O(4)–Eu(1)–N(1) |
146.82(12) |
O(6)–Eu(1)–O(2) |
149.60(12) |
O(5)–Eu(1)–N(1) |
105.55(11) |
O(4)–Eu(1)–O(2) |
101.95(13) |
O(1)–Eu(1)–N(3) |
127.79(12) |
O(5)–Eu(1)–O(2) |
138.07(12) |
N(1)–Eu(1)–N(3) |
64.26(11) |
Eu2
|
Eu(1)–O(3) |
2.320(3) |
Eu(1)–O(2) |
2.413(3) |
Eu(1)–O(6) |
2.345(4) |
Eu(1)–O(1) |
2.444(3) |
Eu(1)–O(4) |
2.381(3) |
Eu(1)–N(1) |
2.508(4) |
Eu(1)–O(5) |
2.394(4) |
Eu(1)–N(2) |
2.601(4) |
O(3)–Eu(1)–O(6) |
93.87(14) |
O(2)–Eu(1)–O(1) |
69.50(11) |
O(3)–Eu(1)–O(4) |
71.04(11) |
O(3)–Eu(1)–N(1) |
100.59(12) |
O(6)–Eu(1)–O(4) |
77.08(15) |
O(6)–Eu(1)–N(1) |
145.19(13) |
O(3)–Eu(1)–O(5) |
147.45(12) |
O(2)–Eu(1)–N(2) |
75.86(13) |
O(4)–Eu(1)–O(2) |
130.60(13) |
O(1)–Eu(1)–N(2) |
81.27(12) |
O(5)–Eu(1)–O(2) |
128.52(13) |
N(1)–Eu(1)–N(2) |
64.98(11) |
To underpin the analysis of the photophysical properties through theoretical calculations, it is necessary to determine the ground state geometry of the complex in question. The ground state geometry of the complexes (Eu1 and Eu2) was optimized from the crystallographic coordinates (details are included in the ESI† and the optimized structure is shown in Fig. S5, ESI†). The comparison involving the crystallographic geometry and the geometries calculated by different DFT methods in terms of root mean square deviations (RMSD) is shown in Table S4, ESI.† Contrary to our previous work,9a,b the TZVPPD basis set (PBE1PBE/TZVPPD/MWB52) provided the best results for all the atoms in the complexes (Table S4, ESI†).
It is important to establish the solid-state packing in a given complex since it helps to understand the thermal and optical properties. The packing diagram of Eu2 exhibits an extensive set of π⋯π stacking interactions of 3.411 Å and 3.781 Å between the aromatic rings of adjacent ligands to generate a one-dimensional (I-D) chain structure along the a-axis (Fig. 2b). Interestingly, Eu1 does not show any sign of π⋯π interactions; however, a π⋯F (2.417 Å) interaction is observed between the aromatic ring of the TB-Im ligand and tfac ligand within the crystal lattice that generates a long chain of stacked molecules along the crystallographic a-axis (Fig. 2a). For Eu1 there is an intermolecular N–H⋯O hydrogen bond involving the N–H unit of the TB-Im ligand (N(2)–H(2)⋯O(2i), H(2)⋯O(2i) 2.24 Å, N(2)⋯O(2i) 3.028(5) Å, N(2)–H(2)⋯O(2i) 153°; symmetry code (i) ½ − x, −½ + y, ½ − z). There is also a relatively short C–H⋯F intermolecular interaction (C(11)–H(11b)⋯F(6ii), H(11b)⋯F(6ii) 2.54 Å, C(11)⋯F(6ii), 3.461(10) Å, C(11)–H(11b)⋯F(6ii) 161°; symmetry code (ii) ½ + x, 3/2 − y, −½ + z). The N–H unit in Eu2 also participates in a hydrogen bond (N(3)–H(3)⋯O(1iii), H(3)⋯O(1iii) 2.16 Å, N(3)⋯O(1iii) 2.915(6) Å, N(3)–H(3)⋯O(1iii) 147°; symmetry code (iii) 1 − x, 1 − y, 1 − z), there is also a NH⋯F (2.586 Å) interaction with one of the hfac ligands, and F⋯F interactions (2.938 Å) between the molecules.
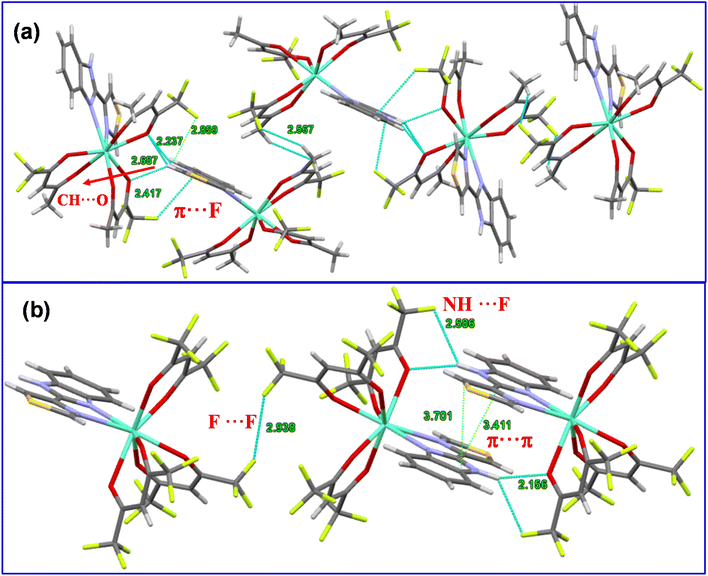 |
| Fig. 2 Packing diagram of the complexes displaying different interactions (a) Eu1 and (b) Eu2. | |
3.2. Photophysical studies
Optical absorption spectroscopy was used to evaluate the light absorbing capability of the complexes. The complexes displayed broad spectra in the region between 250 and 350 nm with λmaxabs at 287 nm (ε = 8041 M−1 cm−1) and 293 nm (ε = 11
375 M−1 cm−1) for Eu1 and Eu2, respectively, attributed to a composite of π–π* transitions of both primary antenna and ancillary ligands (Fig. 3a). Moreover, the spectrum of Eu2 displayed a minor (6 nm) but meaningful redshift in λmaxabs compared to that of Eu1. This could be attributed to the greater number of fluorine (F) atoms on the ligands in Eu2 which is favourable for inducing increased intramolecular charge transfer (ICT).19
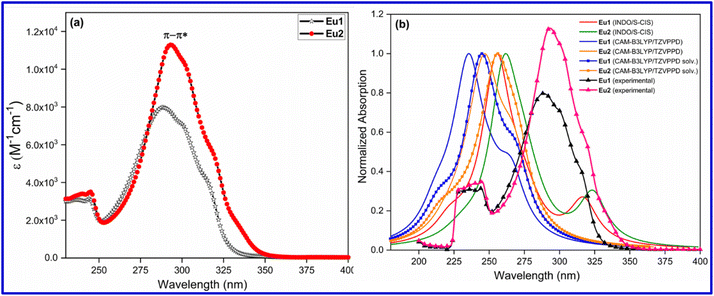 |
| Fig. 3 (a) Optical absorption spectra of Eu1 and Eu2 in DCM solution (1 × 10−5 M). (b) Overlapped experimental and theoretical absorption spectra calculated by the TD-DFT (with and without the effect of solvent) and INDO/S-CIS methods for Eu1 and Eu2 using the geometry optimized at the PBE1PBE/TZVPPD/MWB52 level of theory. | |
To rationalize the experimental optical absorption spectroscopy results, we calculated the theoretical absorption spectra by the TD-DFT and INDO/S-CIS methods (Fig. 3b) employing the optimized geometry with the PBE1PBE/TZVPPD/MWB52 level of theory. As can be seen from Fig. 3b, the λabsmax value is marginally under calculated which is similar to our recent study, where different TD-DFT methods (CAM-B3LYP, M06-2X, PBE1PBE and ωB97X-D3BJ) were used.9b However, the band shape and redshift (ca. 9 nm) of spectra obtained by the TD-DFT methods are in qualitative agreement with the experimental spectra of Eu1 and Eu2 suggesting that the TD-DFT approach could be an important tool to provide the most relevant electronic transitions to the most intense band.
The electronic transitions calculated at the CAM-B3LYP/TZVPPD/MWB52 level of theory, considering the effect of the DCM solvent, are presented in Table 2. It is possible to observe that the most important molecular orbitals (MOs) involved in the most intense band range from HOMO−4 to LUMO+4. The most intense bands of both complexes are due to electronic transitions involving MOs centred both in the primary β-diketonate and ancillary ligands (Fig. 4). The natural transition orbitals (NTOs) analysis performed at the same level of theory (Fig. S6, ESI†) further corroborated this observation. This analysis provides a simple representation of the transition density between the ground and the excited state20 and reveals additionally that the longest wavelength band of Eu1 is due to the electronic transitions involving MOs centred on the ancillary ligand. It is important to mention that the ligand containing a greater number of F-atoms provides a larger electron density resulting in a larger contribution to the band. Similar conclusions are obtained from the analysis of the TD-DFT results without considering the implicit effect of the DCM solvent (Table S5 and Fig. S7, ESI†).
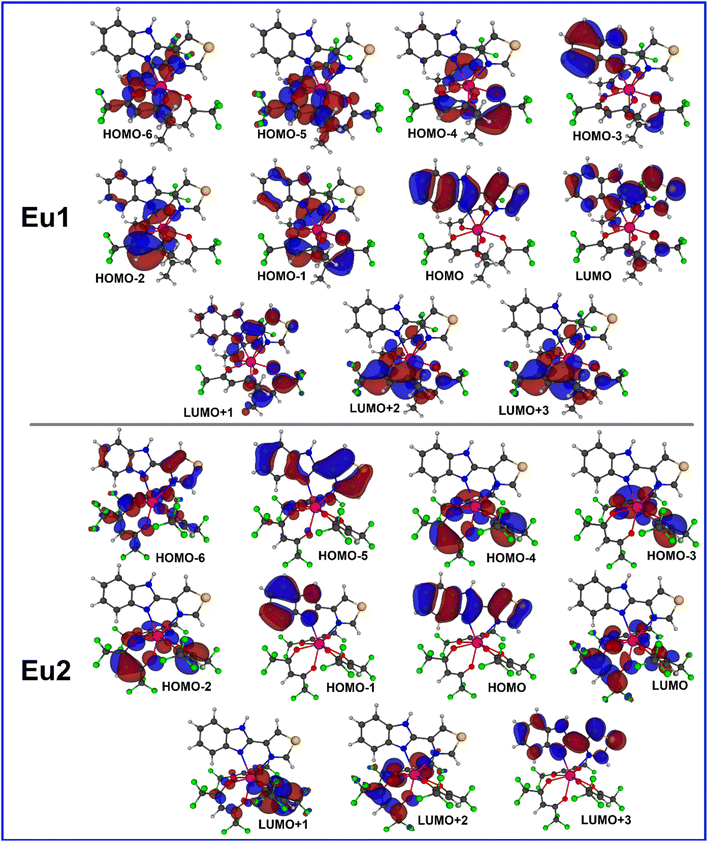 |
| Fig. 4 Most relevant MOs calculated at the TD-DFT CAM-BLYP level of theory that explains the main electronic transitions. The implicit effect of the DCM solvent was considered in the calculation. | |
Table 2 Electronic transitions calculated at the CAM-B3LYP/TZVPPD/MWB52 level of theory for the most intense bands of Eu1 and Eu2 considering the effect of the DCM solvent
|
λ, nm/oscillator strength |
Major contribution |
Total |
MOs centred on the ancillary ligand are highlighted in bold. |
Eu1
|
246.3/0.7912 |
HOMO−1 → LUMO+3 (17.88%) |
68.90% |
HOMO−4 → LUMO+1 (13.62%) |
HOMO−3 → LUMO (13.43%) |
HOMO−2 → LUMO+2 (11.59%) |
HOMO−1 → LUMO+1 (6.31%) |
HOMO−2 → LUMO (6.07%) |
269.0/0.5553 |
HOMO → LUMO (60.81%) |
83.78% |
HOMO → LUMO+1 (17.46%) |
HOMO → LUMO+4 (5.50%) |
Eu2
|
254.4/0.9220 |
HOMO → LUMO+1 (28.41%) |
72.19% |
HOMO → LUMO (21.12%) |
HOMO → LUMO+2 (10.90%) |
HOMO−2 → LUMO (6.64%) |
HOMO−4 → LUMO+1 (5.12%) |
270.7/0.4056 |
HOMO → LUMO+3 (60.77%) |
76.92% |
HOMO−2 → LUMO (10.59%) |
HOMO → LUMO+4 (5.55%) |
Exciting the complexes at their λmaxabs exhibited five typical well-resolved emission transitions a–e (Table 3) in the region between 550–750 nm (Fig. 5) without any residual ligand fluorescence (RFL) between 380–500 nm (Fig. S8 and S9, ESI†) implying good energy transfer ET. Table 3 shows the barycentre of the emission transitions (a–e) and % contribution of the transition intensities relative to the magnetic dipole (MD) 5D0 → 7F1. The intensity of the emission spectra follow a similar trend as the molar absorptivity values i.e., Eu2 > Eu1. The spectra of both complexes are dominated by the narrow (FWHM < 4 nm) electric dipole (ED) 5D0 → 7F2 transition with a contribution greater than 78% of the total emission intensity. Moreover, the superiority of the ED transition over the MD transition indicates dynamic coupling (DC) as the dominant mechanism in the emission process.21 From the emission spectra of the complexes, we further calculated the CIE coordinates of the emitted colour (Table 3). As can be seen from the inset of Fig. 5 (Fig. S10 and S11, ESI†) both the complexes displayed pure red emission with CIE coordinates very close to recommended CIE by NTSC (x, 0.67; y, 0.33) for red emission.
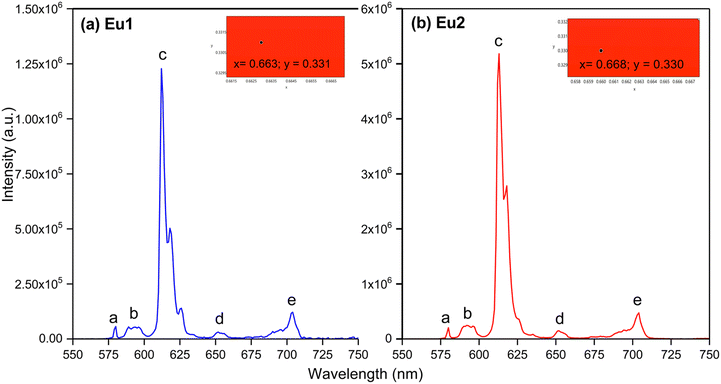 |
| Fig. 5 PL spectra of (a) Eu1 and (b) Eu2 in DCM solution. Inset showing the magnified view of the CIE colour with their coordinates calculated from the emission spectra. | |
Table 3 Experimental and theoretical photophysical properties of Eu1 and Eu2 in DCM
Photophysical parameters |
Eu1
|
Eu2
|
Values in the bracket are % contribution relative to MD transition; values in square parentheses are theoretically calculated; Ω2 and Ω4 were calculated by applying eqn (1) and (2); ARad and ANRad were calculated by applying eqn (2) and (4); QEuEu and ηSen were calculated by applying eqn (6) and (7). |
5D0 → 7F0 (a) |
17 261.32 cm−1 (1.09%) |
17 247.68 cm−1 (0.86%) |
5D0 → 7F1 (b) |
16 880.18 cm−1 |
16 863.62 cm−1 |
5D0 → 7F2 (c) |
16 237.29 cm−1 (78.63%) |
16 236.99 cm−1 (80.36%) |
5D0 → 7F3 (d) |
15 298.74 cm−1 (2.73%) |
15 324.53 cm−1 [2.61%] |
5D0 → 7F4 (e) |
14 286.42 cm−1 (11.35%) |
14 308.93 cm−1 (10.33%) |
FWHM of 5D0 → 7F2 |
3.76 nm |
3.36 nm |
Intensity ratio (R21) |
12.88 |
14.02 |
CIE color coordinates |
x = 0.663; y = 0.331 |
x = 0.668; y = 0.330 |
τ
obs
|
885 ± 2.37 μs (χ2 = 1.002) |
977± 1.82 μs (χ2 = 1.021) |
Ω
2 (× 10−20 cm2) |
22.66 × 10−20 cm2 |
24.57 × 10−20 cm2 |
[22.61 × 10−20 cm2] |
[24.57 × 10−20 cm2] |
Ω
4 (× 10−20 cm2) |
7.62 × 10−20 cm2 |
7.25 × 10−20 cm2 |
[7.81 × 10−20 cm2] |
[7.24 × 10−20 cm2] |
A
Rad
|
744.01 s−1 [721.90 s−1] |
786.18 s−1 [764.37 s−1] |
A
NRad
|
385.93 s−1 [408.04 s−1] |
236.52 s−1 [259.17 s−1] |
Q
Eu
Eu
|
65.84% [63.89%] |
76.87% [74.68%] |
Q
Eu
L
|
39.33% [38.32%] |
47.00% [45.97%] |
η
Sen
|
59.73% [59.98%] |
61.14% [61.56%] |
Apart from the steady-state emission studies, it is of importance to establish other potential experimental photophysical parameters to fully understand the emission phenomena in a given complex before their potential use in optoelectronic devices such as OLEDs can be established. In this regard, we first determined the excited lifetime (τobs) of the 5D0 emitting state of Eu1 and Eu2 in solution. The τobs was calculated by the fitting of the PL decay curve (Fig. S12, ESI†) as shown in Fig. S13 and S14, ESI.† The PL decay profiles for both the complexes reveal monoexponential behaviour and confirm the presence of single emitting species. This is supported by the crystal structure determinations of the complexes and further corroborates the steady-state emission spectra where a single well-resolved emission peak is observed for 5D0 → 7F0. The τobs values in the microsecond timescale regime (Eu1 = 885 ± 2.37 μs and Eu2 = 977± 1.82 μs, Table 3) are well within the range of typical europium β-diketonate N^N complexes. The absolute PLQY (QLEu) of the complexes follows a similar trend as noted for the steady-state emissions and the excited lifetimes. The complexes displayed fairly large values of QLEu1 = 39.33% and QLEu2 = 47.00% in solution. To understand it better, we have calculated ARad and ANRad rates for the complexes by applying a set of eqn (2)–(4), which are presented in Table 3. It is clear from Table 3 that the large QLEu2 value of Eu2 compared to Eu1 is due to the lower ANRad = 236.52 s−1 of Eu2, which is further reflected in its QEuEu = 76.87% leading to sensitization efficiency of ηSen = 61.14%. Finally, J–O intensity parameter (Ω2 and Ω4) was calculated (Table 3). In each case Ω2 displayed a large value implying that the symmetry around the Eu(III) is distorted which indeed is the case as determined by the single-crystal structure determinations (distorted triangular dodecahedron). As expected, Ω2 value of Eu2 (24.57 × 10−20 cm2) is larger than that of Eu1 (22.66 × 10−20 cm2), which is due to more distorted coordination geometry at the Eu(III) centre; in Eu2 (CShM = 0.695) compared to Eu1 (CShM = 0.629). The Ω4 parameter is less sensitive to the coordination sphere; however, it is related to long-range effects (hydrogen bonding and π–π stacking).21 The significantly large value of the Ω4 = 7.62 × 10−20 cm2 and 7.25 × 10−20 cm2 for Eu1 and Eu2, respectively, points to the presence of these effects. This is further supported by the single crystal X-ray structure where these long-range effects are encountered. The theoretically calculated Ω2 and Ω4 (Table 3) using the QDC model22 (Table S6, ESI†) and ARad compare well with the experimental data (Table 3). Moreover, we further calculated the contribution of the forced electric dipole intensity parameters ΩFEDλ. The low values of ΩFEDλ (Table S6, ESI†) attest that the emission for the present complexes is dominated by the dynamic coupling (DC) mechanism, a commonly observed phenomenon in these types of complexes. Furthermore, a low value of ΩFEDλ indicates that Ωλ is strongly dependent on the polarizabilites of the ligand atoms of the complexes. It is worth mentioning that ΩFEDλ plays an important role in estimating the ET rates from the direct coulombic interaction (CI) mechanism which is operative when the J quantum number of the states of the Eu(III) ion involved in the electronic excitations satisfy the |ΔJ| = 2, 4, and 6 selection rule.
3.3. Intermolecular energy transfer (IET)
TD-DFT calculations were also performed to evaluate the impact of the geometry on the lowest energy singlet (S1) and triplet states (T1). The TD-DFT CAM-B3LYP/TZVP/MWB52 approach was applied considering all the optimized geometries by the different DFT methods (Table S7, ESI†). It is important to emphasize that RL parameter and energy of S1 and T1 varied modestly which could be attributed to the similar RMSD values in each structure (Table S4, ESI†). Moreover, a careful analysis of Table S6, ESI,† further revealed that the effect of solvent on the T1 of Eu2 is more prominent than that on Eu1. The solvent caused an average stabilization of 285 cm−1 in the T1 state of Eu2 while this is only 25 cm−1 for Eu1. An explanation for this is that the electron density due to the various peripheral F atoms of hfac in Eu2 interacts more strongly with the electric field produced by the solvent compared to tfac of Eu1. Using the default setting of LUMPAC23 for INDO/S-CIS model, we found a much lower value for T1 for both the complexes (14
550.3 and 14
424.1 cm−1 for Eu1 and Eu2, respectively, Table S7, ESI†) which is in contrast to our recent work.9a,c The analysis of the electronic transitions involved in T1 calculated by the INDO/S-CIS model showed that the most important MOs are situated on the TB-Im ligand. To confirm this behaviour, we further replaced the ancillary ligand by two water molecules and found the T1 was around 20
000 cm−1 thus confirming that the lowering is due to the TB-Im ligand.
To propose the ET mechanism of the complexes, the results obtained using the TD-DFT CAM-B3LYP/TZVPPD/MWB52 method (DCM solvent) with the geometry optimized at the PBE1PBE/TZVPPD/MWB52 level of theory were utilized. The ligand–metal ET rate for a given pathway depends on the energy and distance RL of the excited states involved in the ET process (see eqn (S2) and (S3), ESI†). The energy of the lowest S1 and T1 states and their corresponding RL value states are shown in Table 5. An analysis of the electronic transitions for S1 and T1 (Table 5) in conjunction with the MOs (Fig. 4) suggests that S1 and T1 involved MOs centred on the β-diketonate and ancillary ligands. In case of Eu2, 93.4% of the electronic transitions that compose T1 strongly depend on MOs centred only on the hfac ligands without any role of the TB-Im ligand. The analysis of MOs showed additionally that the lowest three triplet states of Eu2 had almost similar energies (T1 = 22
252.4 cm−1, T2 = 22
385.9 cm−1 and 22
622.0 cm−1) and involved only MOs centred on the different β-diketones ligands. A slightly different situation was observed for the degenerate T1 (24
326.3 cm−1), T2 (24
379.8 cm−1) and T3 (22 622.0 cm−1) states of Eu1, since these states had contribution from TB-Im mainly through HOMO−3, HOMO−1, LUMO, and LUMO. The T4 state (27
129.5 cm−1) of Eu2 is composed of MOs centred mainly on the TB-Im. The contribution of MOs centred on the TB-Im explains why the energy of T1 for Eu1 is higher than the corresponding state of Eu2.
Table 4 Energy of the S and T excited states, distance from energy donor to acceptor centre (RL), and electronic transitions for the corresponding excited states of the complexes calculated with the TD-DFT CAM-B3LYP/TZVPPD/MWB52 approach considering the effect of DCM
Compound |
State |
Energy/cm−1 |
R
L/Å |
Major contribution |
Total |
MOs highlighted in bold are those centred in the ancillary ligand. |
Eu1
|
S1 |
35 475.1 |
3.4485 |
HOMO−6 → LUMO+3 (32.83%) |
89.18% |
HOMO−6 → LUMO+1 (14.25%) |
HOMO−6 → LUMO+2 (9.80%) |
HOMO−5 → LUMO+3 (9.64%) |
HOMO−6 → LUMO (9.21%) |
HOMO−5 → LUMO+2 (7.15%) |
HOMO−5 → LUMO+1 (6.29%) |
T1 |
24 326.3 |
3.6026 |
HOMO−1 → LUMO+1 (27.66%) |
84.14% |
HOMO−4 → LUMO+3 (17.40%) |
HOMO−4 → LUMO+2 (11.48%) |
HOMO−1 → LUMO (9.89%) |
HOMO−4 → LUMO+1 (9.52%) |
HOMO−3 → LUMO+1 (8.19%) |
Eu2
|
S1 |
34 505.5 |
3.2805 |
HOMO−6 → LUMO+2 (26.04%) |
88.04% |
HOMO−6 → LUMO (17.21%) |
HOMO−7 → LUMO+2 (15.53%) |
HOMO−5 → LUMO+2 (9.19%) |
HOMO−8 → LUMO+2 (8.07%) |
HOMO−7 → LUMO (6.30%) |
HOMO−5 → LUMO (5.70%) |
T1 |
22 252.4 |
3.2957 |
HOMO−2 → LUMO (38.87%) |
93.40% |
HOMO−4 → LUMO (19.17%) |
HOMO−2 → LUMO+2 (16.89%) |
HOMO−4 → LUMO+2 (12.68%) |
HOMO−4 → LUMO+1 (5.79%) |
T4 |
27 129.5 |
3.1828 |
HOMO → LUMO+3 (78.57%) |
85.22% |
HOMO−5 → LUMO+3 (6.66%) |
Table 5 Key electroluminescent properties of the single-EML devices of Eu1 and Eu2 operating at J = 10 mA cm−2
Doping concentration (device) |
V
turn-on (V) |
B
(cd m−2) |
η
c b (cd A−1) |
η
p c (lm W−1) |
EQE |
CIEx,y d |
The data for maximum brightness (B).
Maximum current efficiency (ηc).
Maximum current efficiency (ηp).
CIE at J = 10 mA cm−2.
|
Eu1
|
2 wt% (Device 1) |
5.0 |
32.56 |
0.068 |
0.034 |
0.044% |
(0.284, 0.264) |
3 wt% (Device 2) |
4.6 |
55.11 |
0.079 |
0.046 |
0.049% |
(0.354, 0.288) |
4 wt% (Device 3) |
4.6 |
100.5 |
0.109 |
0.055 |
0.068% |
(0.329, 0.285) |
5 wt% (Device 4) |
4.6 |
29.47 |
0.049 |
0.027 |
0.035% |
(0.294, 0.243) |
6 wt% (Device 5) |
5.4 |
21.52 |
0.048 |
0.025 |
0.030% |
(0.285, 0.282) |
Eu2
|
2 wt% (Device 1) |
3.5 |
710.4 |
0.441 |
0.396 |
0.304% |
(0.283, 0.199) |
3 wt% (Device 2) |
3.5 |
736.1 |
0.524 |
0.461 |
0.327% |
(0.305, 0.220) |
4 wt% (Device 3) |
3.9 |
364.1 |
0.276 |
0.223 |
0.186% |
(0.333, 0.228) |
6 wt% (Device 4) |
4.1 |
238.8 |
0.229 |
0.176 |
0.150% |
(0.386, 0.259) |
8 wt% (Device 5) |
4.1 |
186.2 |
0.205 |
0.157 |
0.135% |
(0.386, 0.256) |
The calculated ET rates for the complexes employing Malta's model24 for different excited levels of Eu(III) and the excited states of the ligands are shown in Table 4 and Table S8, ESI.† ET channels that are governed by the CI and Ex. mechanisms are distinguished in Table S8† and the back ET rate (WBET) was estimated by using the sum where both mechanisms were operative. The largest value of ET rate (WET) observed for the S1 state of Eu1 is of the order of 105 s−1 and is related to the 7F1 → 5G2,3 acceptor levels of Eu(III). Because of the resonance condition involving S1 of Eu2 and the 5D4, 5D3 and 5G3 states, rates of this order were observed for 7F0 → 5D4, 7F1 → 5D3 and 7F1 → 5G3 acceptors, where all these rates are governed by the CI mechanism. A rate of the order of 106 was observed for the 7F1 → 5G2 acceptor for Eu2 and is dominated by the Ex. mechanism. Since S1 of both complexes were overestimated, WBET involving all the excited states considered for Eu(IIII) are practically negligible. When the ET pathways from T1 is analysed, it is noted that the 7F1 → 5D0 state has WET value of 108 and 109 s−1 for Eu1 and Eu2, respectively, revealing the importance of this channel in sensitizing the PL of the complexes. Table S8† shows large values of WBET for some pathways involving T1. In these cases, since the states of the Eu(III) ion are significantly above T1, the energy returns to T1 which can then be transferred to more resonant states of Eu(III) such as the 5D0 and 5D1 states. Moreover, ET from T4 state in Eu2 provides a significant value of rate (107 s−1) for the pathways related to 7F0 → 5D1 (3.24 × 107 s−1), 7F1 → 5D0 (2.79 × 107 s−1) and 7F1 → 5D2 (1.54 × 107 s−1). The RL value for T4 is sufficiently small (3.1828 Å) to favour the ligand-Eu(III) ET. A rate of 108 s−1 is noted for the T4 → 7G2 channel; however, a considerable WBET is obtained due to the good resonance condition between T4 and 5G2 (9.80 × 106 s−1; Table S8, ESI†).
The most important energy migration channels for the sensitized PL of the complexes are depicted as the Jablonski diagram (Fig. 6). Since the experimental ηSen for both the complex is ≈60%, a plausible explanation for these experimental observations could be due to the presence of pathways that eventually depopulates to T1. To reproduce the experimental results some rates involving ligand states were adjusted as already applied in previous work.9b As can be seen in Fig. 6, decay rates for Eu1 involving the S1 → T1 and T1 → S1 pathways of the order of 106 and 107 s−1, respectively, provided a theoretical ηSen = 60.0%, which agrees with the experimental ηSen = 59.73%. The energy-level diagram for Eu2 shows that rates of the order 105, 109 and 109 s−1 for S1 → T4, T4 → T1 and T1 → S0 provided a theoretical ηSen of 61.6%. It is important to mention that the other experimental photophysical parameters were also reproduced well for both complexes.
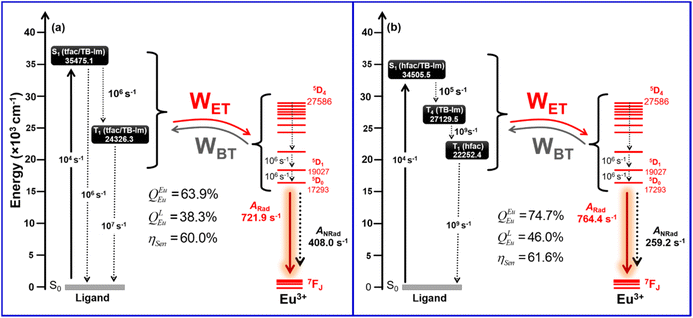 |
| Fig. 6 Schematic energy-level diagram for (a) Eu1 and (b) Eu2, showing the states considered in the modelling of the ET of both complexes. | |
3.4. Electroluminescence and electrophysical properties of Eu1 and Eu2 based OLEDs
The thermal stability of Eu1 and Eu2 is of critical importance since inferior thermal stability of the complex tends to reduce device stability, especially at the peak of its operation. This is due to the Joule heating when current flows through the organic layers.21 In view of this, the thermal stability of Eu1 and Eu2 in the temperature range between 50–800 °C under a dinitrogen (N2) atmosphere was determined. The thermogram of the complexes (Fig. 7 and Fig. S15, ESI†) does not exhibit any weight loss in the 50–150 °C region, implying that complexes have no lattice held/coordinated solvent/water molecules as is evident from the FT-IR and crystallographic studies. The DTA of the complexes displayed an endothermic peak at 228.9 and 202 °C for Eu1 and Eu2, respectively, representing the melting temperature (Tm). It is noteworthy that Tm of Eu1 is higher than Eu2 which possibly could be attributed to π⋯F (2.417 Å) interactions within the crystal lattice. The decomposition temperature (Td) with 5% weight loss of the complexes is 241 °C for Eu1 and 239 °C for Eu2, respectively. The high thermal stability of the complexes implies that they can easily be employed to fabricate OLEDs by the vacuum thermal evaporation method.
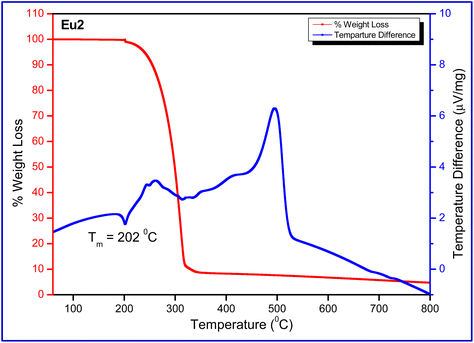 |
| Fig. 7 TGA and DTA profile of Eu2 under N2 atmosphere. | |
Realizing the good PL and thermal properties of Eu1 and Eu2 and to fully establish their potential as an active component in OLEDs, we finally fabricated OLEDs by the vacuum thermal evaporation method and evaluated their EL and electrophysical properties. To investigate EL performances of the complexes, multilayers OLEDs were fabricated (please see ESI† for device details). The doping concentrations of the active component (Eu1 and Eu2) were varied. Interestingly, as the doping concentration increased the temperature of evaporation (Tevp) increased simultaneously gradually [145–154 °C for Eu1 and 139–149 °C for Eu2]. However, it remained very low compared to Tm and Td [Tm = (228.9 °C)Eu-1 and (202 °C)Eu2 and Td = (241 °C)Eu-1 and (239 °C)Eu2] during the fabrication processes of the devices and implies that no decomposition and melting of the complexes occurred, thus EL arising in the OLEDs are due to complexes. The EL performance data obtained such as brightness (B), current efficiency (ηc), power efficiency (ηp), external quantum efficiency (EQE) and CIE colour coordinates for single-EML as well as double-EML OLEDs of Eu1 and Eu2 at J = 10 mA cm−2 are summarized in Table 5 and Table S9, ESI.†
As can be seen the EL spectra of the single- and double-EML devices of both Eu1 and Eu2 (Fig. 8) displayed emission transitions originating from the Eu(III) ion implying that both carrier-trapping and Förster ET are taking part in the device luminescent processes.9b–d,21 Moreover, EL spectra of Eu1 and single-EML Eu2 devices exhibited broad host emission in the region between 380–550 nm similar to the PL spectra of the doped films except the change in the intensity (Fig. S16–S19, ESI†). The presence of broad emission suggests that ET from host to Eu(III) ion is small. Moreover, the intensity of host emission for Eu1 based devices increased with the doping concentration except for the 6 wt% device, impling poor carrier-trapping on the Eu1 molecules and incomplete ET from the host to Eu(III) molecules. However, this trend is reversed for Eu2 based devices implying enhanced carrier-trapping and improved ET from host to Eu2 molecules.
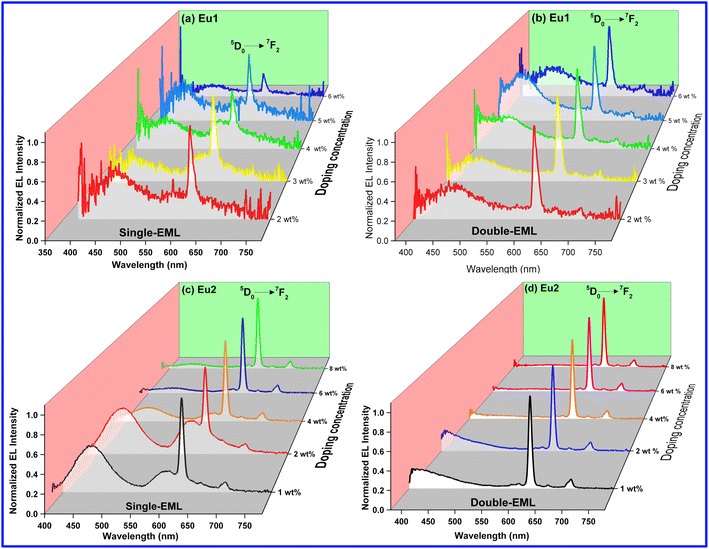 |
| Fig. 8 Normalized EL spectra of single- and double-EML devices of Eu1 and Eu2 at different doping concentrations operating at J = 10 mA cm−2. | |
The host emission in the region between 400–500 nm coupled (Fig. S20, ESI†) with the typical red emission of Eu(III) ion at 600–620 nm due to the electric dipole 5D0 → 7F2 transition prove beneficial in obtaining colour tunable OLEDs (Fig. 9, Fig. S21 and S22, ESI†). At the 4 wt% (Device 3) doping concentration, the emission of devices based on Eu1 and Eu2 fall in the white light emission region with CIE colour coordinates of (0.329, 0.285)Eu1 and (0.333, 0.228)Eu2 (Table 6), respectively. Furthermore, we have calculated the colour correlated temperature (CCT) of the emitted light that measures the coolness and warmness of the light by the McCamy method.25 The CCT values of Device 3 for Eu1 (5719 K) and Eu2 (5356 K) fall in the cool white light category and thus could be a potential candidate for lighting that will represent the real colour of the objects i.e., kitchen, garage, workshop, product displays, industrial applications, etc.
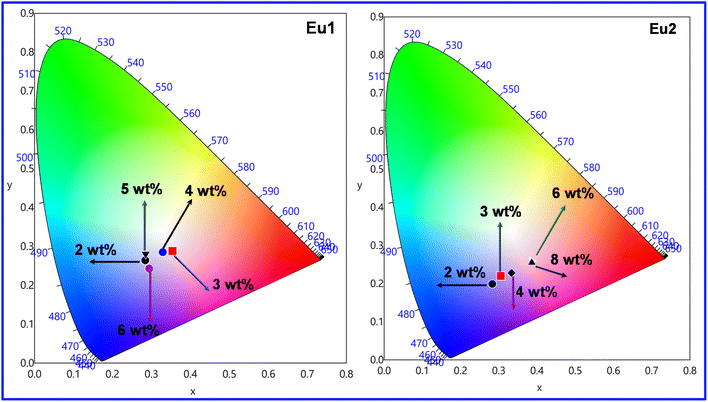 |
| Fig. 9 CIE 1931 chromaticity diagrams of single-EML Eu1 and Eu2 based devices at different doping concentrations operating at J = 10 mA cm−2. | |
Table 6 A comparative electroluminescent characteristic of single component W-OLEDs
Complex |
B
(cd m−2) |
V
turn-on (V) |
η
p (lm W−1) |
CIE |
Ref. |
Where TCPD = 1-[3,4,5-tris[4-(9H-carbazol-9-yl)butoxy]-phenyl]-3-phenylpropane-1,3-dione; Phen = 1,10-phenanthroline; tta = thenoyltrifluoroacetonate; L = 2-(3,5-dimethyl-2H-pyrrol-2-yl)-4-(3,5-dimethyl-3H-pyrrol-2-yl)-6-(4-(pentan-3-yl)phenyl)-1,3,5-triazine; bpm = 2,2′-bipyrimidine. The data for maximum brightness (B) and be placed after the definitions of the ligands on a new line. |
Eu1
|
55.11 |
4.6 |
0.034 |
0.354, 0.288 |
This work |
Eu2
|
736.1 |
3.5 |
0.461 |
0.305, 0.220 |
This work |
[Eu(TCPD)3Phen] |
229 |
20.5 |
0.2 (10.5 V) |
0.333; 0.348 |
26a
|
[Eu(tta)3L] |
945.1 |
16 |
— |
0.337; 0.362 |
27
|
[Eu2(tta)6]bpm |
19.7 |
7.6 |
— |
0.350; 0.330 |
26b
|
The current density (J)–voltage (V)–brightness (B) curves are shown in Fig. 10 and Fig. S23 & S24, ESI† for Eu1 and Eu2, respectively. The detailed EL performances such as B, ηc, ηp and EQE of single- as well as double-EML devices based on Eu1 and Eu2 are summarized in Table 5 & Table S9, ESI.† The white-OLED of Eu1 displayed B = 100.5 cd m−2, ηc = 0.109 cd A−1, ηp = 0.055 lm W−1, EQE = 0.068% with the turn-on voltage = 4.6 V, while Eu2 based W-OLED exhibited improved EL performance (B = 364.1 cd m−2, ηc = 0.276 cd A−1, ηp = 0.223 lm W−1, EQE = 0.186% with the very low turn-on voltage = 3.9 V). It is important to mention that the electroluminescent properties of the present single component white-OLEDs are higher than the reported ternary europium complexes.26
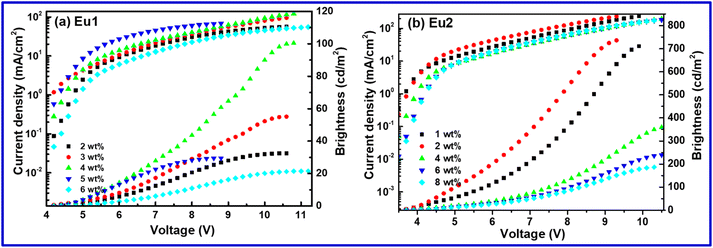 |
| Fig. 10 Current density (J)–voltage (V)–brightness (B) curve of the single component OLEDs of (a) Eu1 and (b) Eu2. | |
4. Conclusion
Two new OEuCs were succesfully synthesized and structurally charcaterized. Analysis of the crystallographically determined molecular structures through the CShMs analysis revealed that the geometry around the Eu(III) centre is distorted triangular dodecahedral, with approximate D2d symmetry’ in both structures. The complexes displayed fairly strong light absorbing capability and the most intense absorption transitions are a composite of MOs centred both on the primary β-diketonate and ancillary ligands. NTOs analysis also revealed that ligand with greater number of Fatoms provides a larger electron density and thus results in a larger contribution to the most intense transition. Exciting the complexes at their λmaxabs exhibited typical well-resolved red emission with reasonable QLEu value. A close scrutiny of the TD-DFT results suggest that electronic transitions that compose T1 in Eu2 (93.4%) exclusively rely on the MOs of hfac while for Eu1 both tfac and Tb-Im MOs are involved resulting in higher T1 state in Eu1 compared to Eu2. The 7F1 → 5D0 state have large WET values of 108 and 109 s−1 for Eu1 and Eu2, respectively, emphasising the significance of this channel in sensitizing the PL of the complexes. Finally, the complexes were successfully employed as EML to fabicate OLEDs. The emission of Device 3 of Eu1 and Eu2 falls in the white light emission region with superior EL performance to the few reported single component white-OLEDs of ternary europium complexes. Furthermore, calculated CCT values fall in the cool white light category and points to its potential for lighting that will represent the real colour of the objects i.e., kitchen, garage, workshop, product displays, industrial applications, etc.
Conflicts of interest
The authors declare no conflicts of interest.
Acknowledgements
MSK acknowledges His Majesty's Trust Fund for Strategic Research (Grant No. SR/SQU/SCI/CHEM/21/01) for funding. RI thanks HM's Trust Fund for a postdoctoral fellowship. JDLD appreciates the financial support from the Brazilian funding agencies: CAPES, CNPq (421733/2018-7), FACEPE (APQ - 0675-1.06/14) and FAPITEC-SE (Process N. 019.203.01074/2011-1). WFO thanks PIBIC/CNPq/UFS for a scientific initiation fellowship. The computing for this project was performed on the LCAD-UFS (UFS High Performance Computing Laboratory). LZ is grateful for the financial aid from the National Natural Science Foundation of China (62174160), Youth Innovation Promotion Association of the Chinese Academy of Sciences (2013150). WYW thanks the Hong Kong Research Grants Council (PolyU 153058/19P), Guangdong-Hong Kong-Macao Joint Laboratory of Optoelectronic and Magnetic Functional Materials (2019B121205002), the CAS-Croucher Funding Scheme for Joint Laboratories (ZH4A), Hong Kong Polytechnic University (1-ZE1C), Research Institute for Smart Energy (CDAQ) and the Endowed Professorship in Energy from Miss Clarea Au (847S) for the financial support. PRR is grateful to the Engineering and Physical Sciences Research Council (EPSRC) for funding (Grant EP/K004956/1).
References
-
(a) R. Ilmi, S. Kansız, N. K. Al-Rasbi, N. Dege, P. R. Raithby and M. S. Khan, New J. Chem., 2020, 44, 5673–5683 RSC;
(b) R. Ilmi, M. S. Khan, W. Sun, L. Zhou, W.-Y. Wong and P. R. Raithby, J. Mater. Chem. C, 2019, 7, 13966–13975 RSC;
(c) Y. He, L. Liu, G. Fu, W. Li, X. Lü, H. He and W.-Y. Wong, J. Mater. Chem. C, 2019, 7, 4800–4807 RSC;
(d) R. Ilmi, M. S. Khan, Z. Li, L. Zhou, W.-Y. Wong, F. Marken and P. R. Raithby, Inorg. Chem., 2019, 58, 8316–8331 CrossRef CAS PubMed;
(e) R. Ilmi, E. Tseriotou, P. Stylianou, Y. A. Christou, I. Ttofi, N. Dietis, C. Pitris, A. D. Odysseos and S. N. Georgiades, Mol. Pharm., 2019, 16, 4260–4273 CrossRef CAS PubMed.
- M. Pan, W.-M. Liao, S.-Y. Yin, S.-S. Sun and C.-Y. Su, Chem. Rev., 2018, 118, 8889–8935 CrossRef CAS PubMed.
-
(a) Y. Kitagawa, M. Tsurui and Y. Hasegawa, RSC Adv., 2022, 12, 810–821 RSC;
(b) A. N. Al-Khalili, I. J. Al-Busaidi, R. Ilmi, M. Al-Mandhary, M. S. Khan and N. K. Al-Rasbi, Inorg. Chim. Acta, 2020, 501, 119226 CrossRef CAS.
- K. Yanagisawa, Y. Kitagawa, T. Nakanishi, T. Seki, K. Fushimi, H. Ito and Y. Hasegawa, Chem. – Eur. J., 2018, 24, 1956–1961 CrossRef CAS PubMed.
- M. Rajendran, R. Devi, S. Mund, K. Singh and S. Vaidyanathan, J. Mater. Chem. C, 2021, 9, 15034–15046 RSC.
-
(a) S. Sato and M. Wada, Bull. Chem. Soc. Jpn., 1970, 43, 1955–1962 CrossRef CAS;
(b) M. Latva, H. Takalo, V. M. Mukkala, C. Matachescu, J. C. RodriguezUbis and J. Kankare, J. Lumin., 1997, 75, 149–169 CrossRef CAS.
-
(a) S. I. Weissman, J. Chem. Phys., 1942, 10, 214–217 CrossRef CAS;
(b)
N. Sabbatini, M. Guardigli and I. Manet, in Handbook on the Physics and Chemistry of Rare Earths, ed. K. A. Gschneidner Jr. and E. LeRoy, Elsevier, 1996, vol. 23, pp. 69–119 Search PubMed;
(c) R. Ilmi, N. Hasan, J. Liu, D. Mara, R. Van Deun and K. Iftikhar, J. Photochem. Photobiol., A, 2017, 347, 116–129 CrossRef CAS.
-
(a) R. Ilmi, A. Haque, I. J. Al-Busaidi, N. K. Al Rasbi and M. S. Khan, Dyes Pigm., 2019, 162, 59–66 CrossRef CAS;
(b) R. Ilmi, S. Anjum, A. Haque and M. S. Khan, J. Photochem. Photobiol., A, 2019, 383, 111968 CrossRef CAS.
-
(a) I. J. Al-Busaidi, R. Ilmi, D. Zhang, J. D. L. Dutra, W. F. Oliveira, N. K. Al Rasbi, L. Zhou, W.-Y. Wong, P. R. Raithby and M. S. Khan, Dyes Pigm., 2022, 197, 109879 CrossRef CAS;
(b) R. Ilmi, D. Zhang, J. D. L. Dutra, N. Dege, L. Zhou, W.-Y. Wong, P. R. Raithby and M. S. Khan, Org. Electron., 2021, 96, 106216 CrossRef CAS;
(c) M. S. Khan, R. Ilmi, W. Sun, J. D. L. Dutra, W. F. Oliveira, L. Zhou, W.-Y. Wong and P. R. Raithby, J. Mater. Chem. C, 2020, 8, 5600–5612 RSC;
(d) R. Ilmi, W. Sun, J. D. L. Dutra, N. K. Al-Rasbi, L. Zhou, P.-C. Qian, W.-Y. Wong, P. R. Raithby and M. S. Khan, J. Mater. Chem. C, 2020, 8, 9816–9827 RSC.
- G. M. Farinola and R. Ragni, Chem. Soc. Rev., 2011, 40, 3467–3482 RSC.
- T. N. Singh-Rachford and F. N. Castellano, Coord. Chem. Rev., 2010, 254, 2560–2573 CrossRef CAS.
- R. Ilmi, A. Haque and M. S. Khan, J. Photochem. Photobiol., A, 2019, 370, 135–144 CrossRef CAS.
- K. Yanagisawa, T. Nakanishi, Y. Kitagawa, T. Seki, T. Akama, M. Kobayashi, T. Taketsugu, H. Ito, K. Fushimi and Y. Hasegawa, Eur. J. Inorg. Chem., 2015, 2015, 4769–4774 CrossRef CAS.
- L. Farrugia, J. Appl. Crystallogr., 1999, 32, 837–838 CrossRef CAS.
-
(a)
G. Sheldrick, SADABS, Program for Empirical Absorption Correction of Area Detector Data, University of Göttingen, Göttingen, Germany, 1996 Search PubMed;
(b) G. Sheldrick, Acta Crystallogr., Sect. A: Found. Adv., 2015, 71, 3–8 CrossRef PubMed.
- C. F. Macrae, P. R. Edgington, P. McCabe, E. Pidcock, G. P. Shields, R. Taylor, M. Towler and J. van de Streek, J. Appl. Crystallogr., 2006, 39, 453–457 CrossRef CAS.
- R. Ilmi, S. Kansız, N. Dege and M. S. Khan, J. Photochem. Photobiol., A, 2019, 377, 268–281 CrossRef CAS.
-
(a) M. Pinsky and D. Avnir, Inorg. Chem., 1998, 37, 5575–5582 CrossRef CAS PubMed;
(b) D. Casanova, M. Llunell, P. Alemany and S. Alvarez, Chem. – Eur. J., 2005, 11, 1479–1494 CrossRef CAS PubMed.
- R. Ilmi, H. Al-Sharji and M. S. Khan, Top. Curr. Chem., 2022, 380, 18 CrossRef CAS PubMed.
- R. L. Martin, J. Chem. Phys., 2003, 118, 4775–4777 CrossRef CAS.
- R. Ilmi, D. Zhang, L. Tensi, H. Al-Sharji, N. K. Al Rasbi, A. Macchioni, L. Zhou, W.-Y. Wong, P. R. Raithby and M. S. Khan, Dyes Pigm., 2022, 203, 110300 CrossRef CAS.
- J. D. L. Dutra, N. B. D. Lima, R. O. Freire and A. M. Simas, Sci. Rep., 2015, 5, 13695 CrossRef.
- J. D. Dutra, T. D. Bispo and R. O. Freire, J. Comput. Chem., 2014, 35, 772–775 CrossRef CAS PubMed.
-
A. N. Carneiro Neto, E. E. S. Teotonio, G. F. de Sá, H. F. Brito, J. Legendziewicz, L. D. Carlos, M. C. F. C. Felinto, P. Gawryszewska, R. T. Moura, R. L. Longo, W. M. Faustino and O. L. Malta, in Handbook on the Physics and Chemistry of Rare Earths, ed. J.-C. G. Bünzli and V. K. Pecharsky, Elsevier, 2019, vol. 56, pp. 55–162 Search PubMed.
- C. S. McCamy, Color Res. Appl., 1992, 17, 142–144 CrossRef.
-
(a) S. Li, G. Zhong, W. Zhu, F. Li, J. Pan, W. Huang and H. Tian, J. Mater. Chem., 2005, 15, 3221–3228 RSC;
(b) G. Zucchi, T. Jeon, D. Tondelier, D. Aldakov, P. Thuery, M. Ephritikhine and B. Geffroy, J. Mater. Chem., 2010, 20, 2114–2120 RSC.
- G.-L. Law, K.-L. Wong, H.-L. Tam, K.-W. Cheah and W.-T. Wong, Inorg. Chem., 2009, 48, 10492–10494 CrossRef CAS PubMed.
|
This journal is © The Royal Society of Chemistry 2022 |
Click here to see how this site uses Cookies. View our privacy policy here.