DOI:
10.1039/D1EA00075F
(Communication)
Environ. Sci.: Atmos., 2022,
2, 128-136
Environmentally persistent free radicals in indoor particulate matter, dust, and on surfaces†
Received
21st September 2021
, Accepted 6th January 2022
First published on 7th January 2022
Abstract
Environmentally persistent free radicals (EPFR) are an emerging class of constituents in particulate matter (PM). They are relatively stable with lifetimes of days to years in the condensed phase, influence PM toxicity, and are involved in reactive oxygen species (ROS) generation and multiphase chemistry. The abundance of EPFR has been investigated in laboratory studies and outdoor locations globally, but their prevalence indoors was previously unstudied. In a case study home, EPFR were present at similar concentrations in indoor and outdoor PM, at 2.3 ± 2.8 × 1012 and 3.6 ± 3.1 × 1012 spins m−3, respectively. Indoor EPFR were observed at varying concentrations in airborne PM, dust, and surface samples, with the most concentrated surface loadings observed on windows. Overall, dust and indoor surfaces were estimated to be larger contributors to total in-home EPFR concentrations than PM. For comparison, combined estimates of total EPFR concentrations in indoor PM, dust, and on surfaces are similar to the range of typical indoor ozone concentrations. Due to the reactive nature of EPFR, they potentially undergo rapid cycling and may represent reservoirs of reactivity in these multiphase systems that influence the surface and multiphase chemistry of indoor environments. Here, the exposure of EPFR collected from some surfaces to nitrogen dioxide or ozone was shown to alter EPFR abundances and composition. In all, these results support further investigations of EPFR abundance, composition, and reactivity on indoor surfaces, including in accumulated organic coatings and dust.
Environmental significance
EPFR have been proposed as an indicator of outdoor PM toxicity but have not been examined indoors despite humans spending 90% of their time inside. Given that EPFR are long-lived, it is valuable to understand both their indoor airborne abundances and their accumulation on surfaces and in dust. Our observations of EPFR in indoor PM, surface, and total settled dust samples are directly relevant for human health, but EPFR may also influence indoor multiphase chemistry, especially given the lower concentrations of gas-phase radicals. The results demonstrate a diversity of EPFR reservoirs with varied composition and reactivity that warrant comparative studies across indoor environments, including locations with higher outdoor PM that could increase the penetration and accumulation of deposited outdoor EPFR.
|
Introduction
With people spending ∼90% of their time in enclosed spaces,1 indoor multiphase chemistry is a key consideration for human health.2 The indoor environment has an abundance of surfaces and other reservoirs for condensed and aqueous phase chemistry with greater total organic loadings than outdoors, thus playing a larger role in physical–chemical processes and human exposure.2–6 Films comprised of organic and inorganic compounds accumulate indoors on airborne particles, surfaces, and dust, including in air handling systems (e.g. ducts, filters). They include primary and secondary organic compounds, soot, and metals that originate from a mix of outdoor and indoor sources.3,7,8
These accumulated films represent a major part of indoor multiphase systems. Chemistry at aerosol surfaces is known to be important, but other surfaces in the built environment are relatively less studied. However, surfaces are known to be off-gassing sources as well as sinks for the deposition of aerosols and gases.3,9,10 Examples include multiphase processes important to urban HONO chemistry11,12 and reactive surfaces in rooms or air handling systems (e.g. filters) that are sources of oxidized species (e.g. carbonyls).11,13–15
Organic and aqueous surface layers represent potential reservoirs for radicals that initiate and propagate multiphase chemistry.16 Organic oxidation chemistry often regenerates oxidative radicals (e.g. HOx) and this process could play a larger role in indoor oxidant budgets considering the relative lack of photochemistry.17,18 A subset of oxidized organic compounds generated as byproducts of oxidation (i.e. reactive oxygen intermediates (ROI)) have been shown to survive in aerosols as environmentally persistent free radicals (EPFR).19–24 For example, they have been observed in layers of polycyclic aromatic compounds (i.e. PAHs) exposed to oxidants, as well as in combustion-related emissions (e.g. soot, cigarette smoke) and ambient PM.19,25,26 The long lifetimes of EPFR (∼days–years) make them able to retain and transport chemistry-propagating radicals beyond their initial formation.17,20 This presents concerns for human health through inhalation and associated oxidative stress,27,28 but also indicates their function as reservoirs of reactivity in the atmosphere.29–32 Their formation is highly dependent on oxidation conditions, metal content, and precursor types.33,34 EPFR previously identified in outdoor samples include oxygen- and carbon-centered organic radicals (e.g.
˙CnHmOp),29,35,36 but, the molecular identities of the oxidized organics linked to ambient EPFR are largely unknown, with the exception of quinones, semi-quinones and organic peroxides.20,37,38
Radical transport via EPFR and EPFR-converted reactive oxygen/nitrogen species (ROS/RNS) has been shown to be important for in-lung chemistry and climate-related cloud chemistry.21,29–32,39–41 While their importance as reservoirs of relatively stable, oxidation-initiating compounds may translate to indoor environments via airborne PM, on surfaces, and in dust, where they can accumulate over time, the abundance of EPFR indoors is largely unknown. Here, we present an exploratory investigation of the presence and potential implications of EPFR in suspended PM, dust, and on surfaces within a residence. Specifically, we (a) collected 20 long duration indoor PM samples over 2 months in fall 2019 with concurrent outdoor samples from a background site and examined their EPFR abundances via electron paramagnetic resonance spectroscopy (EPR); (b) compared airborne PM samples to both surface swabs and dust samples from the same home; (c) exposed a subset of samples to ppb-level ozone (O3) and nitrogen dioxide (NO2) concentrations to evaluate EPFR reactivity; and (d) estimated the relative contributions of indoor EPFR to both PM and total in-home reactive species to contextualize their importance for indoor multiphase chemistry.
Methods
To examine the airborne PM that can accumulate on indoor surfaces, total suspended particles were actively collected at ∼1 m3 h−1 onto PTFE filters (Pall Corp.) in PFA filter holders (Savillex Corp.) during Sept.–Nov. 2019 at two locations in Mainz, Germany: in an apartment and also outdoors at an established EPFR measurement site on the roof of the Max Planck Institute for Chemistry (49°59′28.6′′N 8°13′45.4′′E; see prior work29 for additional details on the outdoor site). PM collection was done in parallel at both sites with sampling times ranging 1–7 days with some variations in human occupancy (Tables S1, S2 and Fig. S1†). For comparison, we collected indoor surface swabs as well as total settled dust samples from the same apartment at the completion of the campaign (Table S3†). The total house dust samples were physically collected from under furniture without any solvent, pretreatment, or sieving, and was used (along with the surface swabs) as time-integrated samples of deposited analytes from indoor air, similar to prior work.42,43 To maintain consistency with the established EPR analytical methods for PM used in this and prior work29 and to reduce EPR artifacts from other sampling materials (e.g. quartz, cellulose), surfaces were swabbed with a PTFE filter to determine the relative composition of the accessible surface film and any settled PM/dust (i.e. EPFR concentration per sampled mass; EPFR spins μg−1). To facilitate collection of the deposited organic films, the filters/surfaces were slightly wetted with acetone immediately prior to sampling, and the impact of acetone wetting on EPFR abundances and composition was separately shown to be negligible in methods testing with dust. See ESI† for more detailed descriptions of the indoor site and additional details on sample collection methods.
A Bruker EMXplus X-Band EPR spectrometer was used for all sample analysis, with instrument configurations and filter sample handling/processing following prior work and detailed in the ESI.†29 To obtain consistent lattice conditions and prevent humidity-induced spin-lattice relaxation, filters were dried for 1+ hours in high purity N2 gas, which has no negative impact on EPFR recovery.44 All samples in filters were inserted into the EPR spectrometer (without solvent extraction) in 2 mm ID quartz tubes, which were continuously flushed with N2 at < 0.05 slpm. Quantification of EPFR spins used double integration of baseline corrected spectra, via Bruker Xenon software following established methods.29 The supplemental experiments with exposure of samples to 50–350 ppb ozone or NO2 were performed online by flowing each gas through the sample-containing EPR tube (Fig. S3†) at concentrations set to examine accelerated atmospheric aging over a shorter time period with real-time measurements in the EPR.
Results and discussion
EPFR observed in indoor PM, dust, and surface samples
Indoor PM at the residential apartment contained 0.2–7.1 × 1011 spins μg−1 across samples (N = 19; 51 days total) (Fig. S4a†) and had a similar range of volumetric EPFR concentrations (i.e., spins m−3) as the outdoor reference site (Fig. 1a), both of which varied over 1–2 orders of magnitude during the 2 month observation period (0.4–13.2 × 1012 spins m−3). While the sites were not immediately co-located, indoor and outdoor EPFR volumetric concentrations were often similar in the time-integrated samples (Fig. 1a) but were not always correlated (r = 0.83) and lower concentrations were sometimes observed at the indoor site (Fig. S1c†).
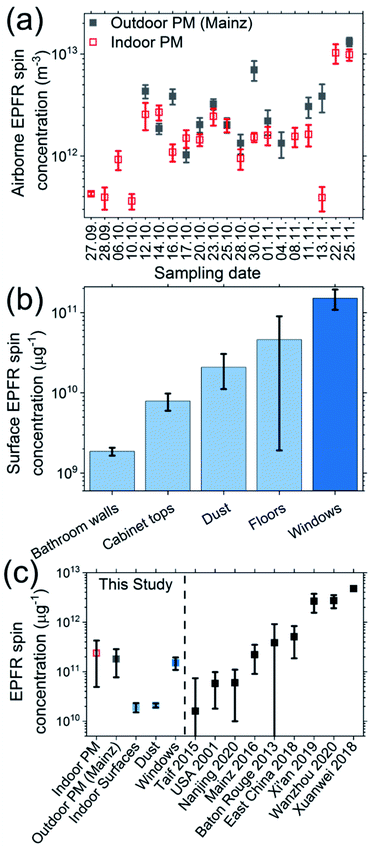 |
| Fig. 1 Concentrations of environmentally persistent free radicals (EPFR) in a home apartment compared to outdoor air and other locations. (a) Volumetric EPFR spin concentrations in total suspended particulate matter (PM) inside an apartment collected in 2019 compared to an outdoor sampling site in Mainz, Germany during the same time period. (b) Mass-normalized EPFR spin concentrations on surfaces and in dust inside the apartment (Nbathroom walls = 2; Ncabinet tops = 4; Ndust = 2; Nfloor surfaces = 2; Nwindows = 2). (c) Comparison of observed spin concentrations to prior outdoor studies on PM.19,26,36,45–48,50,51 Error bars in panel a indicate standard deviations based on measurement uncertainties; panel b's indicate the range of observations within each sample type, and panel c's indicate the range observed in each respective study. | |
On average, inhabited periods had somewhat higher indoor volumetric EFPR concentrations (2.7 × 1012 ± 3.1 × 1012 spins m−3) than largely uninhabited periods (9.7 × 1011 ± 6.7 × 1011 spins m−3; N ∼ 4; 19 days; p < 0.05) (Fig. S4b and Table S1†). While there was a limited number of uninhabited samples and considerable variance within each sample set, the results suggest that occupancy may influence EPFR concentrations. This could occur via increased indoor activities (e.g. cooking, cleaning) that may contribute to EPFR generation or resuspension, or through greater ventilation resulting in more outdoor EPFR intrusion. However, variations in indoor activities during inhabited periods did not exhibit obvious changes in EPFR concentrations over the multi-day timescales sampled here (Table S1†). In all, the results indicate similar indoor and outdoor EPFR concentrations, and that indoor airborne EPFR may vary with outdoor PM penetration, ventilation conditions, and/or indoor activities. Though further time-resolved samples are warranted to constrain the contributions of these various processes.
Surfaces, which comprise an outsize fraction of organic loading in indoor environments,6 exhibited significant EPFR concentrations that varied between surface types (Fig. 1b). The mass-specific EPFR concentrations (spins μg−1) on surfaces, resulting from the combination of condensed films and deposited particulates, were greatest on windows (15 × 1010 spins μg−1) followed by floors (4.6 × 1010 spins μg−1), cabinet tops (0.79 × 1010 spins μg−1), and tiled bathroom walls (0.19 × 1010 spins μg−1). The high concentrations on windows could be influenced by the increased photochemical EPFR production via transmitted solar radiation, which is highest on/near windows, and leads to greater rates of OH generation, photolysis of photolabile compounds, and possible photosensitized reactions.17,45–49
The total dust samples (i.e. loose dust under furniture) analyzed separately also had substantial EPFR (2.0 × 1010 spins μg−1). Given dust's prevalence in most indoor spaces and its high surface-to-volume ratio, it can be an efficient condensational sink and accessible interface for multiphase processes. While accumulated dust would have been incorporated with horizontal surface samples (e.g. floors, cabinet tops), it would have smaller contributions to vertical surfaces (e.g., windows, walls). Settled PM (and condensed organics) will make contributions to dust, but lower EPFR concentrations in dust vs. PM (Fig. 1c) are likely influenced by substantial contributions from other dust components (e.g., skin flakes, hair, fabric debris, microplastics), which may dilute EPFR concentrations.
The EPFR concentrations measured in this study fall within the range of outdoor PM2.5 EPFR observations in prior studies (Fig. 1c). Specifically, the outdoor PM results are similar to previous summertime EPFR measurements in Mainz29 and are comparable to spin concentrations found in Baton Rouge (US)20 and three different sites in eastern China.50 However, other studies found considerably higher51–53 or lower54–56 concentrations of EPFR in PM2.5. This highlights the variance in this new class of pollutants in different environments, which may be explained by different PM sources, chemistry, and/or PM2.5 concentrations.53,57,58 For example, the substantially elevated EPFR spin concentrations observed on window surfaces are most similar to the concentrations in outdoor and indoor PM in Mainz (Fig. 1c), which may indicate differences in composition and chemistry compared to other surfaces and greater similarity to outdoor PM due to photochemical aging.17,59
Electron paramagnetic resonance spectra reveal diverse and reactive EPFR content
The various indoor samples showed significant differences in indoor EPFR composition based on their EPR spectra (Fig. 2a–c), which indicates the occurrence of different carbonaceous species with unpaired electrons. In all samples, we found a single broad peak with a g-value of 2.002–2.004, which corresponds to the frequently described EPFR signal often associated with combustion processes (type 1).20 Additionally, on some surfaces (Fig. 2a) and to a smaller extent in dust (Fig. 2c), we found a signal constituting a triplet with 1
:
2
:
1 intensity distribution (44 G splitting), centered around 2.0120 (type 2). Such a pattern may be explained by two equivalent hydrogen atoms close to the unpaired electron and strong influences of heteroatoms potentially due to oxidation inducing a signal shift in the magnetic field possibly leading to an O-centered radical.19,60,61 This type 2 species is less-commonly described in the literature and the chemical structure may significantly differ compared to classical EPFR. In all, the results demonstrate two prominent, distinct molecular types with long-lived unpaired electrons on all indoor surfaces types, but ratios of the two observed radicals varied substantially in between surface types (see Fig. S2 and S5† for additional spectra and Table S3† for quantitative breakdown of type 1 vs. 2 EPFR in surface/dust samples).
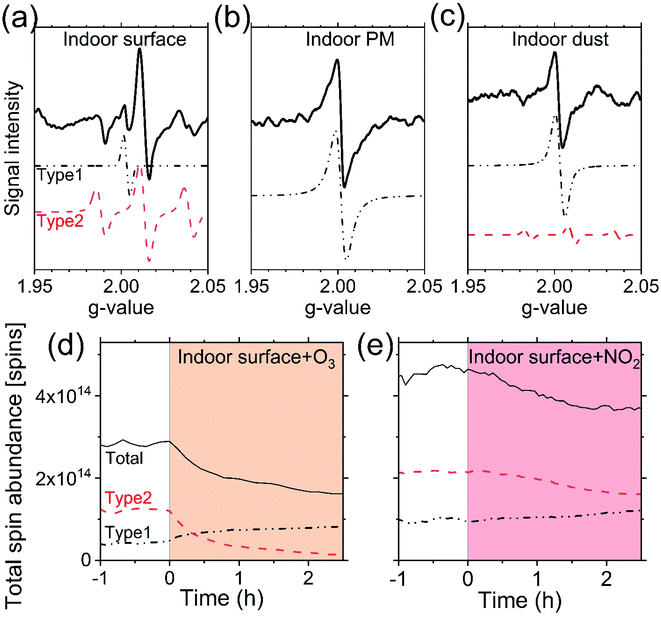 |
| Fig. 2 Examples of electron paramagnetic resonance (EPR) spectra of indoor EPFR in PM, dust, and surfaces and their reactivity with ozone and nitrogen dioxide. (a–c) EPR spectra of indoor surface (i.e. cabinet top), PM, and dust samples. Solid bold lines indicate the observed raw spectra, and dashed lines indicate type 1–2 radicals obtained by peak-fitting the raw spectra. (d and e) Changes in EPFR abundances for total observed radicals and type 1–2 radicals in indoor surface samples upon exposure to (d) ozone (cabinet top; sample #1) and (e) NO2 (cabinet top; sample #3) (with t = 0 occurring after being flushed with dry N2 gas prior to O3 or NO2 exposure), though real-world timescales will likely be longer dependent on in-room reactant concentrations. The total spin abundances shown in (d and e) are without normalization for sample mass or volume. Examples of exposure experiments for other samples are shown in Fig. S6 and S7.† See notes on spectra fitting and these specific samples in the text and ESI.† | |
We exposed a selection of samples to ozone or NO2 to evaluate EPFR evolution with typical reactive species (Fig. 2d, e, S6 and S7†). While EPFR in some PM samples were less responsive, both reactants induced an appreciable change in the EPR signal of the tested surface samples with total EPFR concentrations decreasing soon after ozone exposure, and more slowly for NO2. Type 1 and 2 EPFR signals responded differently; type 1 increased in intensity, while type 2 decreased, further supporting the observation of two distinct molecular EPFR types.
These observed changes may be attributed to the multiphase chemistry of EPFR and their derivatives. For example, Borrowman et al.19 reported EPFR formation upon exposing PAH-containing films to ozone, which then decayed via radical–radical recombination or interaction with ozone, and EPFR quenching by ambient ozone was also recently suggested.62 While similar formation processes may explain the type 1 EPFR increase (Fig. 2), the decay of type 2 EPFR suggests they are sensitive to ozone exposure. Interestingly, EPFR found in surface samples had significantly higher g-values compared to PM (Fig. S8†), which could be due to the prevalence of type 2 radicals and indicative of a higher degree of oxidation in the surface's organic phase.63 Given the observed EPFR variations between tested samples, these observations warrant further studies with larger sample sizes on EPFR composition and fate, including the intermediary products (e.g. ROI) resulting from slow reactions in surface films (e.g. aliphatic radicals or Criegee intermediates resonance-stabilized by transition metals) and the role of environmental conditions.
Conclusions and potential implications of EPFR reservoirs for indoor multiphase chemistry and air quality
The prominence of EPFR in this diverse range of surface, PM, and dust samples from this case study home and their observed and potential reactivity (Fig. 1 and 2) promotes the hypothesis that they may impact indoor aqueous- and condensed-phase systems, interact with other multiphase reactive species, and could act as a reservoir of oxidation-propagating radicals in indoor environments. Yet, the contributions of EPFR originating from indoor vs. outdoor sources, either directly-emitted or chemically-produced, are uncertain across all sample types. However, penetration of outdoor PM is one very likely source of airborne indoor EPFR, and regions with higher outdoor PM will likely lead to greater EPFR abundances in both airborne and deposited PM indoors.2
To explore the relevance of EPFR for multiphase chemistry indoors, we compared the concentrations measured in this study with estimates for typical gas-phase reactive species. EPFR concentrations within the condensed phase (i.e. EPFR per condensed phase volume) were estimated to be higher than those of O3 and NO2 (and thereby NO3) indoors based on their solubility in aerosols or surface films (Fig. 3a, shown per unit volume of air), and thus may enable more radical reactions than would be possible by the uptake of these reactive gases alone.
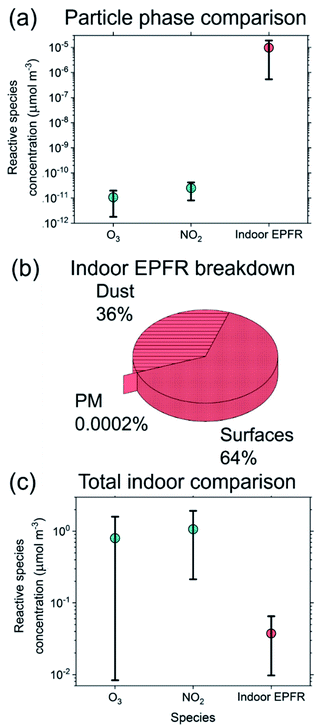 |
| Fig. 3 Potential EPFR contributions to indoor multiphase chemistry. (a) Contributions of EPFR to indoor particle-phase reactive species, shown with EPFR concentrations per unit volume of air compared to that of ozone and NO2 dissolved in suspended PM (according to Henry's law). (b) Distribution of total in-home EPFR based on estimates of the typical prevalence of surfaces and dust indoors. (c) Comparison of total in-home reactive species in both the gas- and condensed-phase (per unit volume of air) for a range of indoor ozone and NO2 concentrations compared to total indoor EPFR estimates including PM, surfaces, and dust. Note: the range presented for indoor EPFR in panel c reflects the range shown in Fig. 1. See ESI† for details about the ranges of air composition considered in panels a and c (0.2–39 ppb O3, 5–45 ppb NO2), as well as assumed room geometry, surface film loadings, and dust prevalence used in this comparative example, which vary across different indoor spaces and influence the in-home distribution of EPFR. | |
We can also use the survey of surface and dust samples to estimate EPFR contributions across indoor reservoirs in order to contextualize their potential relative importance, but note the uncertainties associated with the limited sample sizes. As suspended PM represents a small fraction of the condensed phase within indoor environments,2 estimated EPFR contributions from dust (36%) and surfaces (64%) far exceed contributions from PM (Fig. 3b) when considering their observed EPFR loadings and typical abundances in homes. However, the estimated contributions in Fig. 3b are sensitive to dust/film loadings and home characteristics, and do not include the less-accessible internal reservoirs below surfaces or gas-phase EPFR, the latter of which is expected to be relatively minimal.
We note that Fig. 3's comparisons between EPFR reservoirs (and to other reactive species) are not intended to be representative of human exposure or predictive of health outcomes across exposure routes. For example, EPFR in PM, along with metals, may still be important for in-lung ROS generation and damage.31 Furthermore, dust presents an additional EPFR inhalation route64 with dust resuspension resulting from occupant motion, air handling systems, or vacuuming.65 Yet, the large total in-home EPFR levels accumulated in dust (9.5 × 10−3 μmol m−3) and on surfaces (1.7 × 10−2 μmol m−3) may have additional implications for indoor multiphase chemistry, including in unseen, less-accessible indoor spaces.
These EPFR may be rapidly cycling in interaction with certain molecules (e.g. quinone-semiquinone-hydroquinone cycling).30 With lifetimes ranging days–years17,35 and possible production from indoor ozone reactions, we hypothesize that EPFR could be an appreciable source of condensed-phase radicals (Fig. 3a) and reservoirs of reactivity indoors where the lack of photochemical generation of radicals slows chemical oxidation. Additionally, organic surface films can uptake water during periods of higher indoor relative humidity, which can lead to glass-to-liquid phase transitions and significant increases in condensed-phase reactivity.66,67 Chemical reaction with water could also provide a source of aqueous-phase ROS, including ˙OH,63 O2˙−, or H2O2, when dissolved into aqueous systems (i.e. water-soluble redox activity).30,35,36,40,68–70 Similarly, recent findings have shown these radicals could originate from stable oxidized organic species,26,30 or from EPFR,30,62 in ambient PM.
A comparison of the estimated sum of total in-home EPFR across reservoirs to other indoor reactive species (e.g. O3, NO2; Fig. 3c) suggests that indoor EPFR abundances may fall roughly within the range of seasonally-varying indoor ozone concentrations, which lends support to the hypothesis that EPFR in the condensed phase may contribute appreciably to the total radical budget indoors and influence indoor multiphase chemistry (Fig. S9†). While the scope of this case study in one home was intended to be an exploratory survey of EPFR across PM, surfaces, and dust, the results support further consideration of EPFR reservoirs in indoor chemistry studies and models. We highlight several key areas for future exploration of these hypotheses and associated questions.
• What are the identities, reactions, rate coefficients, fates, and impacts of indoor EPFR; how do they vary among dust, surfaces, and PM; and how do they compare to the abundance of other oxidized non-EPFR organic compounds?
• How do indoor EPFR abundances vary as a function of location and time of year, and what is the influence of indoor EPFR sources?
• Though PM and dust are potentially lower total contributors to EPFR than surface films, how may the greater surface to volume ratio of PM and dust influence multiphase processes?
• How may EPFR participate in heterogeneous chemistry and influence equilibrium partitioning of oxidized gas-phase organics across the volatile-semivolatile range?
• Could the aqueous-phase dissolution of ROS-generating species contribute gas-phase radicals when produced in a small liquid volume and actively partitioned into the gas-phase owing to their Henry's Law coefficients of 10–1000 M atm−1?
• How do the effects of different indoor EPFR vary depending on their degree of oxidation, surrounding substrates, and associated transition metals, including variations between outdoor vs. indoor EPFR and their metal content that could influence radical-related chemistry?
• How can in-home EPFR levels be reduced and is physical cleaning via removal of dust and organic films in accessible areas an effective approach?
• A range of oxidant- (i.e. ˙OH, O3), UV-, ionization-, or chemical-based approaches have recently been increasingly considered for sanitation of indoor air and surfaces as mitigation measures for SARS-CoV-2 transmission. Given the emerging research on the unintended consequences of indoor air purifiers or cleaning products,71–75 studies should consider how an increase in the oxidative capacity of indoor air can induce chemical transformations occurring on PM, dust, and surfaces that may affect indoor EPFR levels and associated multiphase indoor air chemistry.
Finally, while outdoor surfaces are comparatively-less prevalent, surface-bound EPFR may be relevant to outdoor multiphase chemistry. Accordingly, integrating these budgets and constraining the frequency of interactions with other radicals may help improve knowledge on condensed-phase radical reactions.
Data availability
The observed EPFR abundances (and associated sampling details) and EPR spectra observed in all samples are included in the ESI† tables and figures. Raw EPR spectra files can be provided by the authors upon request.
Conflicts of interest
There are no conflicts of interest to declare.
Acknowledgements
D. R. G. is grateful for support from the Alexander von Humboldt Fellowship, which made this collaboration with MPIC possible. A. F. thanks the Max Planck Graduate Center for financial support. R. S. would like to acknowledge financial support from NSF GRFP (DGE1122492 and DGE1752134). H. T. thanks the support from MPIC and start-up fund of PolyU (P0036313). We also thank B. Dix for their help with sample collection.
References
- N. E. Klepeis, W. C. Nelson, W. R. Ott, J. P. Robinson, A. M. Tsang, P. Switzer, J. V. Behar, S. C. Hern and W. H. Engelmann, The National Human Activity Pattern Survey (NHAPS): a resource for assessing exposure to environmental pollutants, J. Exposure Sci. Environ. Epidemiol., 2001, 11, 231–252 CrossRef CAS PubMed.
- J. P. Abbatt and C. Wang, The atmospheric chemistry of indoor environments, Environ. Sci.: Processes Impacts, 2020, 22, 25–48 RSC.
- C. J. Weschler and W. W. Nazaroff, Growth of organic films on indoor surfaces, Indoor Air, 2017, 27, 1101–1112 CrossRef CAS PubMed.
- C. M. Eichler, J. Cao, G. Isaacman-VanWertz and J. C. Little, Modeling the formation and growth of organic films on indoor surfaces, Indoor Air, 2019, 29, 17–29 CrossRef CAS PubMed.
- J. M. Mattila, P. S. Lakey, M. Shiraiwa, C. Wang, J. P. Abbatt, C. Arata, A. H. Goldstein, L. Ampollini, E. F. Katz and P. F. DeCarlo, Multiphase chemistry controls inorganic chlorinated and nitrogenated compounds in indoor air during bleach cleaning, Environ. Sci. Technol., 2020, 54, 1730–1739 CrossRef CAS.
- A. P. Ault, V. H. Grassian, N. Carslaw, D. B. Collins, H. Destaillats, D. J. Donaldson, D. K. Farmer, J. L. Jimenez, V. F. McNeill and G. C. Morrison, Indoor Surface Chemistry: Developing a Molecular Picture of Reactions on Indoor Interfaces, Chem, 2020, 6(12), 3203–3218 CAS.
- V. W. Or, M. Wade, S. Patel, M. R. Alves, D. Kim, S. Schwab, H. Przelomski, R. O'Brien, D. Rim and R. L. Corsi, Glass surface evolution following gas adsorption and particle deposition from indoor cooking events as probed by microspectroscopic analysis, Environ. Sci.: Processes Impacts, 2020, 22, 1698–1709 RSC.
- C. Y. Lim and J. P. Abbatt, Chemical composition, spatial homogeneity, and growth of indoor surface films, Environ. Sci. Technol., 2020, 54, 14372–14379 CrossRef CAS PubMed.
- P. Khare and D. R. Gentner, Considering the future of anthropogenic gas-phase organic compound emissions and the increasing influence of non-combustion sources on urban air quality, Atmos. Chem. Phys., 2018, 18, 5391–5413 CrossRef CAS.
- R. Sheu, C. F. Fortenberry, M. J. Walker, A. Eftekhari, C. Stönner, A. Bakker, J. Peccia, J. Williams, G. C. Morrison and B. J. Williams, Evaluating Indoor Air Chemical Diversity, Indoor-to-Outdoor Emissions, and Surface Reservoirs Using High-Resolution Mass Spectrometry, Environ. Sci. Technol., 2021, 55, 10255–10267 CrossRef CAS PubMed.
- C. J. Weschler and W. W. Nazaroff, Semivolatile organic compounds in indoor environments, Atmos. Environ., 2008, 42, 9018–9040 CrossRef CAS.
- J. Stutz, B. Alicke, R. Ackermann, A. Geyer, S. Wang, A. B. White, E. J. Williams, C. W. Spicer and J. D. Fast, Relative humidity dependence of HONO chemistry in urban areas, J. Geophys. Res.: Atmos., 2004, 109, D03307, DOI:10.1029/2003jd004135.
- D. Poppendieck, H. Hubbard, C. J. Weschler and R. Corsi, Formation and emissions of carbonyls during and following gas-phase ozonation of indoor materials, Atmos. Environ., 2007, 41, 7614–7626 CrossRef CAS.
- G. C. Morrison and W. W. Nazaroff, Ozone interactions with carpet: secondary emissions of aldehydes, Environ. Sci. Technol., 2002, 36, 2185–2192 CrossRef CAS PubMed.
- D. Pagonis, D. J. Price, L. B. Algrim, D. A. Day, A. V. Handschy, H. Stark, S. L. Miller, J. de Gouw, J. L. Jimenez and P. J. Ziemann, Time-resolved measurements of indoor chemical emissions, deposition, and reactions in a university art museum, Environ. Sci. Technol., 2019, 53, 4794–4802 CrossRef CAS PubMed.
- H. Li, H. Guo, B. Pan, S. Liao, D. Zhang, X. Yang, C. Min and B. Xing, Catechol degradation on hematite/silica–gas interface as affected by gas composition and the formation of environmentally persistent free radicals, Sci. Rep., 2016, 6, 1–9 CrossRef PubMed.
- Q. Chen, H. Sun, M. Wang, Y. Wang, L. Zhang and Y. Han, Environmentally persistent free radical (EPFR) formation by visible-light illumination of the organic matter in atmospheric particles, Environ. Sci. Technol., 2019, 53, 10053–10061 CrossRef CAS PubMed.
-
J. H. Seinfeld and S. N. Pandis, Atmospheric Chemistry and Physics: from Air Pollution to Climate Change, John Wiley & Sons, 2016 Search PubMed.
- C. K. Borrowman, S. Zhou, T. E. Burrow and J. P. Abbatt, Formation of environmentally persistent free radicals from the heterogeneous reaction of ozone and polycyclic aromatic compounds, Phys. Chem. Chem. Phys., 2016, 18, 205–212 RSC.
- W. Gehling and B. Dellinger, Environmentally persistent free radicals and their lifetimes in PM2.5, Environ. Sci. Technol., 2013, 47, 8172–8178 CrossRef CAS PubMed.
- U. Poschl and M. Shiraiwa, Multiphase chemistry at the atmosphere-biosphere interface influencing climate and public health in the anthropocene, Chem. Rev., 2015, 115, 4440–4475 CrossRef PubMed.
- M. Shiraiwa, M. Ammann, T. Koop and U. Pöschl, Gas uptake and chemical aging of semisolid organic aerosol particles, Proc. Natl. Acad. Sci. U. S. A., 2011, 108, 11003–11008 CrossRef CAS PubMed.
- H. Jia, S. Zhao, Y. Shi, L. Zhu, C. Wang and V. K. Sharma, Transformation of polycyclic aromatic hydrocarbons and formation of environmentally persistent free radicals on modified montmorillonite: the role of surface metal ions and polycyclic aromatic hydrocarbon molecular properties, Environ. Sci. Technol., 2018, 52, 5725–5733 CrossRef CAS PubMed.
- R. Roy, R. Jan, S. Yadav, M. H. Vasave and P. G. Satsangi, Study of metals in radical-mediated toxicity of particulate matter in indoor environments of Pune, India, Air Qual., Atmos. Health, 2016, 9, 669–680 CrossRef CAS.
- B. Pan, H. Li, D. Lang and B. Xing, Environmentally persistent free radicals: Occurrence, formation mechanisms and implications, Environ. Pollut., 2019, 248, 320–331 CrossRef CAS PubMed.
- F. Hasan, L. Khachatryan and S. Lomnicki, Comparative studies of environmentally persistent free radicals on total particulate matter collected from electronic and tobacco cigarettes, Environ. Sci. Technol., 2020, 54, 5710–5718 CrossRef CAS PubMed.
- J. R. Reed, G. F. Cawley, T. G. Ardoin, B. Dellinger, S. M. Lomnicki, F. Hasan, L. W. Kiruri and W. L. Backes, Environmentally persistent free radicals inhibit cytochrome P450 activity in rat liver microsomes, Toxicol. Appl. Pharmacol., 2014, 277, 200–209 CrossRef CAS PubMed.
- A. C. Harmon, V. Y. Hebert, S. A. Cormier, B. Subramanian, J. R. Reed, W. L. Backes and T. R. Dugas, Particulate matter containing environmentally persistent free radicals induces AhR-dependent cytokine and reactive oxygen species production in human bronchial epithelial cells, PloS one, 2018, 13, e0205412 CrossRef PubMed.
- A. M. Arangio, H. Tong, J. Socorro, U. Pöschl and M. Shiraiwa, Quantification of environmentally persistent free radicals and reactive oxygen species in atmospheric aerosol particles, Atmos. Chem. Phys., 2016, 16, 13105–13119 CrossRef CAS.
- W. Gehling, L. Khachatryan and B. Dellinger, Hydroxyl radical generation from environmentally persistent free radicals (EPFRs) in PM2.5, Environ. Sci. Technol., 2014, 48, 4266–4272 CrossRef CAS PubMed.
- P. S. Lakey, T. Berkemeier, H. Tong, A. M. Arangio, K. Lucas, U. Poschl and M. Shiraiwa, Chemical exposure-response relationship between air pollutants and reactive oxygen species in the human respiratory tract, Sci. Rep., 2016, 6, 32916 CrossRef CAS PubMed.
- H. J. Tong, A. M. Arangio, P. S. J. Lakey, T. Berkemeier, F. B. Liu, C. J. Kampf, W. H. Brune, U. Poschl and M. Shiraiwa, Hydroxyl radicals from secondary organic aerosol decomposition in water, Atmos. Chem. Phys., 2016, 16, 1761–1771 CrossRef CAS.
- E. P. Vejerano, G. Rao, L. Khachatryan, S. A. Cormier and S. Lomnicki, Environmentally persistent free radicals: insights on a new class of pollutants, Environ. Sci. Technol., 2018, 52, 2468–2481 CrossRef CAS PubMed.
- H. Tong, P. S. Lakey, A. M. Arangio, J. Socorro, F. Shen, K. Lucas, W. H. Brune, U. Pöschl and M. Shiraiwa, Reactive oxygen species formed by secondary organic aerosols in water and surrogate lung fluid, Environ. Sci. Technol., 2018, 52, 11642–11651 CrossRef CAS PubMed.
- L. Khachatryan and B. Dellinger, Environmentally persistent free radicals (EPFRs)-2. Are free hydroxyl radicals generated in aqueous solutions?, Environ. Sci. Technol., 2011, 45, 9232–9239 CrossRef CAS PubMed.
- L. Khachatryan, E. Vejerano, S. Lomnicki and B. Dellinger, Environmentally persistent free radicals (EPFRs). 1. Generation of reactive oxygen species in aqueous solutions, Environ. Sci. Technol., 2011, 45, 8559–8566 CrossRef CAS PubMed.
- B. Dellinger, W. A. Pryor, R. Cueto, G. L. Squadrito, V. Hegde and W. A. Deutsch, Role of Free Radicals in the Toxicity of Airborne Fine Particulate Matter, Chem. Res. Toxicol., 2001, 14, 1371–1377 Search PubMed.
- M. Xu, T. Wu, Y.-T. Tang, T. Chen, L. Khachatryan, P. R. Iyer, D. Guo, A. Chen, M. Lyu and J. Li, Environmentally persistent free radicals in PM 2.5: a review, Waste Dispos. Sustain. Energy, 2019, 1, 177–197 Search PubMed.
- M. M. Shafer, J. D. Hemming, D. S. Antkiewicz and J. J. Schauer, Oxidative potential of size-fractionated atmospheric aerosol in urban and rural sites across Europe, Faraday Discuss., 2016, 189, 381–405 RSC.
- R. McWhinney, S. Zhou and J. Abbatt, Naphthalene SOA: redox activity and naphthoquinone gas–particle partitioning, Atmos. Chem. Phys., 2013, 13, 9731–9744 CrossRef.
- R. D. McWhinney, K. Badali, J. Liggio, S.-M. Li and J. P. Abbatt, Filterable redox cycling activity: a comparison between diesel exhaust particles and secondary organic aerosol constituents, Environ. Sci. Technol., 2013, 47, 3362–3369 CrossRef CAS PubMed.
- H. K. Leppänen, M. Täubel, B. Jayaprakash, A. Vepsäläinen, P. Pasanen and A. Hyvärinen, Quantitative assessment of microbes from samples of indoor air and dust, J. Exposure Sci. Environ. Epidemiol., 2018, 28, 231–241 CrossRef PubMed.
- A. Barberán, R. R. Dunn, B. J. Reich, K. Pacifici, E. B. Laber, H. L. Menninger, J. M. Morton, J. B. Henley, J. W. Leff and S. L. Miller, The ecology of microscopic life in household dust, Proc. R. Soc. B, 2015, 282, 20151139 CrossRef PubMed.
- U. Green, Y. Shenberger, Z. Aizenshtat, H. Cohen and S. Ruthstein, Exploring the radical nature of a carbon surface by electron paramagnetic resonance and a calibrated gas flow, Journal of visualized experiments, J. Vis. Exp., 2014, 86, 51548 Search PubMed.
- K. Zhu, H. Jia, S. Zhao, T. Xia, X. Guo, T. Wang and L. Zhu, Formation of environmentally persistent free radicals on microplastics under light irradiation, Environ. Sci. Technol., 2019, 53, 8177–8186 CrossRef CAS PubMed.
- L. Qin, L. Yang, J. Yang, R. Weber, K. Ranguelova, X. Liu, B. Lin, C. Li, M. Zheng and G. Liu, Photoinduced Formation
of Persistent Free Radicals, Hydrogen Radicals and Hydroxyl Radicals from Catechol on Atmospheric Particulate Matter, iScience, 2021, 3, 102193 CrossRef PubMed.
- S. Zhou and T. F. Kahan, Spatiotemporal characterization of irradiance and photolysis rate constants of indoor gas-phase species in the UTest house during HOMEChem, Indoor air, 2021 DOI:10.1111/ina.12966.
- W. Wang, B. Yuan, Y. Peng, H. Su, Y. Cheng, S. Yang, C. Wu, J. Qi, F. Bao and Y. Huangfu, Direct observations indicate photodegradable oxygenated VOCs as larger contributors to radicals and ozone production in the atmosphere, Atmos. Chem. Phys. Discuss., 2021 DOI:10.5194/acp-2021-913.
- E. Gómez Alvarez, H. Wortham, R. Strekowski, C. Zetzsch and S. Gligorovski, Atmospheric Photosensitized Heterogeneous and Multiphase Reactions: From Outdoors to Indoors, Environ. Sci. Technol., 2012, 46, 1955–1963 CrossRef PubMed.
- Q. Chen, M. Wang, H. Sun, X. Wang, Y. Wang, Y. Li, L. Zhang and Z. Mu, Enhanced health risks from exposure to environmentally persistent free radicals and the oxidative stress of PM2. 5 from Asian dust storms in Erenhot, Zhangbei and Jinan, China, Environ. Int., 2018, 121, 260–268 CrossRef CAS PubMed.
- R. Qian, S. Zhang, C. Peng, L. Zhang, F. Yang, M. Tian, R. Huang, Q. Wang, Q. Chen and X. Yao, Characteristics and potential exposure risks of environmentally persistent free radicals in PM2. 5 in the three gorges reservoir area, Southwestern China, Chemosphere, 2020, 252, 126425 CrossRef CAS PubMed.
- P. Wang, B. Pan, H. Li, Y. Huang, X. Dong, F. Ai, L. Liu, M. Wu and B. Xing, The overlooked occurrence of environmentally persistent free radicals in an area with low-rank coal burning, Xuanwei, China, Environ. Sci. Technol., 2018, 52, 1054–1061 CrossRef CAS PubMed.
- Q. Chen, H. Sun, Z. Mu, Y. Wang, Y. Li, L. Zhang, M. Wang and Z. Zhang, Characteristics of environmentally persistent free radicals in PM2. 5: Concentrations, species and sources in Xi'an, Northwestern China, Environ. Pollut., 2019, 247, 18–26 CrossRef CAS PubMed.
- G. L. Squadrito, R. Cueto, B. Dellinger and W. A. Pryor, Quinoid redox cycling as a mechanism for sustained free radical generation by inhaled airborne particulate matter, Free Radicals Biol. Med., 2001, 31, 1132–1138 CrossRef CAS PubMed.
- A. A. Shaltout, J. Boman, Z. F. Shehadeh, R. Dhaif-allah, O. Hemeda and M. M. Morsy, Spectroscopic investigation of PM2. 5 collected at industrial, residential and traffic sites in Taif, Saudi Arabia, J. Aerosol Sci., 2015, 79, 97–108 CrossRef CAS.
- X. Guo, N. Zhang, X. Hu, Y. Huang, Z. Ding, Y. Chen and H.-z. Lian, Characteristics and potential inhalation exposure risks of PM2. 5–bound environmental persistent free radicals in Nanjing, a mega–city in China, Atmos. Environ., 2020, 224, 117355 CrossRef CAS.
- Q. Chen, H. Sun, W. Song, F. Cao, C. Tian and Y.-L. Zhang, Size-resolved exposure risk of persistent free radicals (PFRs) in atmospheric aerosols and their potential sources, Atmos. Chem. Phys., 2020, 20, 14407–14417 CrossRef CAS.
- Y. Wang, S. Li, M. Wang, H. Sun, Z. Mu, L. Zhang, Y. Li and Q. Chen, Source apportionment of environmentally persistent free radicals (EPFRs) in PM2. 5 over Xi'an, China, Sci. Total Environ., 2019, 689, 193–202 CrossRef CAS PubMed.
- Q.-T. Liu, M. L. Diamond, S. E. Gingrich, J. M. Ondov, P. Maciejczyk and G. A. Stern, Accumulation of metals, trace elements and semi-volatile organic compounds on exterior window surfaces in Baltimore, Environ. Pollut., 2003, 122, 51–61 CrossRef CAS PubMed.
- M. J. Davies, Detection and characterisation of radicals using electron paramagnetic resonance (EPR) spin
trapping and related methods, Methods, 2016, 109, 21–30 CrossRef CAS PubMed.
-
P. Bertrand, Electron Paramagnetic Resonance Spectroscopy, Springer Nature, 2020 Search PubMed.
- B. Hwang, T. Fang, R. Pham, J. Wei, S. Gronstal, B. Lopez, C. Frederickson, T. Galeazzo, X. Wang, H. Jung and M. Shiraiwa, Environmentally Persistent Free Radicals, Reactive Oxygen Species Generation, and Oxidative Potential of Highway PM2. 5, ACS Earth Space Chem., 2021, 5, 1865–1875 CrossRef CAS.
- B. Dellinger, S. Lomnicki, L. Khachatryan, Z. Maskos, R. W. Hall, J. Adounkpe, C. McFerrin and H. Truong, Formation and stabilization of persistent free radicals, Proc. Combust. Inst., 2007, 31, 521–528 CrossRef PubMed.
- P. D. Sly, S. A. Cormier, S. Lomnicki, J. N. Harding and K. Grimwood, Environmentally persistent free radicals: linking air pollution and poor respiratory health?, Am. J. Respir. Crit. Care Med., 2019, 200, 1062–1063 CrossRef CAS PubMed.
- B. E. Boor, J. A. Siegel and A. Novoselac, Monolayer and multilayer particle deposits on hard surfaces: literature review and implications for particle resuspension in the indoor environment, Aerosol Sci. Technol., 2013, 47, 831–847 CrossRef CAS.
- E. Mikhailov, S. Vlasenko, S. Martin, T. Koop and U. Pöschl, Amorphous and crystalline aerosol particles interacting with water vapor: conceptual framework and experimental evidence for restructuring, phase transitions and kinetic limitations, Atmos. Chem. Phys., 2009, 9, 9491–9522 CrossRef CAS.
- H. Schwartz-Narbonne and D. J. Donaldson, Water uptake by indoor surface films, Sci. Rep., 2019, 9, 1–10 CrossRef CAS PubMed.
- L. Khachatryan, C. A. McFerrin, R. W. Hall and B. Dellinger, Environmentally persistent free radicals (EPFRs). 3. Free versus bound hydroxyl radicals in EPFR aqueous solutions, Environ. Sci. Technol., 2014, 48, 9220–9226 CrossRef CAS PubMed.
- J. Zhou, M. Elser, R.-J. Huang, M. Krapf, R. Fröhlich, D. Bhattu, G. Stefenelli, P. Zotter, E. A. Bruns and S. M. Pieber, Predominance of secondary organic aerosol to particle-bound reactive oxygen species activity in fine ambient aerosol, Atmos. Chem. Phys., 2019, 19, 14703–14720 CrossRef CAS.
- H. Tong, P. S. Lakey, A. M. Arangio, J. Socorro, C. J. Kampf, T. Berkemeier, W. H. Brune, U. Pöschl and M. Shiraiwa, Reactive oxygen species formed in aqueous mixtures of secondary organic aerosols and mineral dust influencing cloud chemistry and public health in the Anthropocene, Faraday Discuss., 2017, 200, 251–270 RSC.
- D. B. Collins and D. K. Farmer, Unintended Consequences of Air Cleaning Chemistry, Environ. Sci. Technol., 2021, 55, 12172–12179 CrossRef CAS PubMed.
- N. Carslaw, L. Fletcher, D. Heard, T. Ingham and H. Walker, Significant OH production under surface cleaning and air cleaning conditions: Impact on indoor air quality, Indoor Air, 2017, 27, 1091–1100 CrossRef CAS PubMed.
- T. Joo, J. C. Rivera-Rios, D. Alvarado-Velez, S. Westgate and N. L. Ng, Formation of oxidized gases and secondary organic aerosol from a commercial oxidant-generating electronic air cleaner, Environ. Sci. Technol. Lett., 2021, 8, 691–698 CrossRef CAS.
- C. Wang, D. B. Collins and J. P. Abbatt, Indoor illumination of terpenes and bleach emissions leads to particle formation and growth, Environ. Sci. Technol., 2019, 53, 11792–11800 CrossRef CAS PubMed.
- H. Hartwig, Impact of the usage of an UV-C cleaning device on indoor air quality : Part I quantification of the UV-C dose, Zenodo, 2021 DOI:10.5281/zenodo.5145453.
Footnote |
† Electronic supplementary information (ESI) available. See DOI: 10.1039/d1ea00075f |
|
This journal is © The Royal Society of Chemistry 2022 |
Click here to see how this site uses Cookies. View our privacy policy here.