Carbonaceous aerosols and their light absorption properties over the Bay of Bengal during continental outflow†
Received
17th August 2021
, Accepted 18th October 2021
First published on 20th October 2021
Abstract
The marine atmosphere of the Bay of Bengal (BoB) is prone to get impacted by anthropogenic aerosols from the Indo-Gangetic Plain (IGP) and Southeast Asia (SEA), particularly during the northeast monsoon (NEM). In this study, we quantify and characterize carbonaceous aerosols and their absorption properties collected in two cruise campaigns onboard ORV Sindhu Sadhana during the continental outflow period over the BoB. Aerosol samples were classified based on the air mass back trajectory analyses, wherein samples were impacted by the continental air parcel (CAP), marine air parcel (MAP), and mix of both (CAP + MAP). Significant variability in the PM10 mass concentration (in μg m−3) is found with a maximum value for MAP samples (75.5 ± 36.4) followed by CAP + MAP (58.5 ± 27.3) and CAP (58.5 ± 27.3). The OC/EC ratio (>2) and diagnostic tracers i.e. nss-K+/EC (0.2–0.96) and nss-K+/OC (0.11–1.32) along with the absorption angstrom exponent (AAE: 4.31–6.02) and MODIS (Moderate Resolution Imaging Spectroradiometer) derived fire counts suggest the dominance of biomass burning emission sources. A positive correlation between OC and EC (i.e. r = 0.86, 0.70, and 0.42 for CAP, MAP, and CAP + MAP, respectively) further confirmed the similar emission sources of carbonaceous species. Similarly, a significant correlation between estimated secondary organic carbon (SOC) and water-soluble organic carbon (WSOC; r = 0.99, 0.96, and 0.97 for CAP, MAP, and CAP + MAP, respectively) indicate their similar chemical nature as well as dominant contribution of SOC to WSOC. The absorption coefficient (babs-365) and mass absorption efficiency (MAEBrC-365) of the soluble fraction were estimated at 365 nm wherein, babs-365 showed a linear relationship with WSOC and nss-K+, signifying the contribution of water soluble brown carbon from biomass burning emissions. The estimated MAEBrC-365 (0.30–0.93 m2 g−1), during this study, was consistent with the earlier observations over the BoB, particularly during the continental outflow season.
Environmental significance
This study reports the chemical composition and characteristics of carbonaceous aerosols and their absorption properties over the Bay of Bengal during the continental outflow period. The chemical composition data demonstrate dominance of biomass burning emissions from the Indo-Gangetic Basin. A significant spatial variation in carbonaceous species and their characteristics is observed and attributed to long-range transport and prevailing meteorological conditions. The absorption properties of brown carbon were found to contribute significantly towards regional radiative forcing. The role of continental outflow is significant in impacting the aerosol composition in the Bay of Bengal, which have relevance to the understanding of complex linkages between aerosols and surface water biogeochemical processes. Such studies have implication toward the regional radiative forcing as well as on the climate.
|
1. Introduction
Carbonaceous aerosols, mainly derived from anthropogenic emissions, play a vital role in modulating the radiative budget of the Earth's atmosphere.1 Carbonaceous aerosols are composed of organic carbon (OC) and elemental carbon (EC), which contribute significantly to the particulate matter, particularly in the polluted region.2–5 Although the mass fraction of EC (also referred to as black carbon; BC or soot6) in the total aerosol mass load is relatively small compared to mineral dust or sea-salt, yet it is considered to be the second most significant anthropogenic contributor to the global climate change after carbon dioxide.7,8 A net positive BC radiative forcing (+1.1 W m−2) is estimated, with a large uncertainty that may be mainly attributed to poor understanding of its physicochemical characteristics, spatiotemporal distribution, emission sources, long-range transportation and complex atmospheric processes.8 EC is mainly derived as a primary particle from incomplete combustion processes (e.g., fossil fuels and/or biomass burning).3,9–14 The absorption of incoming radiation by EC exerts a direct effect by heating the atmospheric column1,15–17 and semi-direct effect by enhancing cloud evaporation18 and low cloud formation19 as well as by acting as cloud condensation nuclei.20 In contrast to EC, OC scatters the incoming solar radiation and promotes cloud formation, resulting in atmospheric cooling.21 OC is mainly derived from anthropogenic and biogenic sources, including primary emissions from smoldering combustion of fossil fuels as well as natural sources (e.g., pollen and plant waxes3,11,12,22). Global simulation studies indicate that in addition to EC and OC, a significant fraction (about 19%) of the contribution to the total radiation absorption by the anthropogenic aerosols comes from the absorbing fraction of organic aerosols—also known as brown carbon (BrC).6,23–25 This BrC is part of OC and contributes to the absorption of incoming solar ration particularly in the blue to ultraviolet spectral range.26 Thus, to quantify the impact of carbonaceous species using the climate model, it is necessary to assess the relative abundance of EC and OC (aka. the OC/EC ratio) by measuring their concentrations in the ambient atmosphere on the spatial as well as temporal scale, as reported in Novakov et al., 2005.22,27–32 Regional emission of carbonaceous aerosols from India is estimated to be the second-largest in the world with a forecast of increased emissions in the future.3
The Bay of Bengal (BoB), located in the eastern part of the North Indian Ocean, is an enclosed basin surrounded by a densely populated landmass from three sides. The semi-annual reversing monsoon wind system influences this basin and depending on the prevailing meteorology, the surrounding region acts as an important source of anthropogenic as well as natural aerosols.33–37 Due to its potential impact on regional and global climate, the BoB has been an important region and under investigation for the last two decades.35,38–43 Continental aerosols, mainly derived from the Indian subcontinent and Southeast Asia (SEA), advect into the open oceanic region of the BoB between the surface and the mid-troposphere during periods when conducive meteorological conditions exist.44,45 Typically, during the winter months (November—February), the prevailing low-level wind flow picks up gaseous as well as particulate pollutants while traversing through the polluted Indo-Gangetic Plain (IGP).46 During the winter season, IGP experiences large-scale subsidence and enhancement in particulate matter, particularly fine particles.37,47 Thus, the continental outflow from IGP, during winter, brings a unique blend of continental aerosols that gets further mixed while entering into the pristine region over the BoB.36 The prevailing meteorological conditions during the northeast monsoon facilitate significant input of carbonaceous aerosols to the BoB.34,36,39 Such an outflow plays a major role in impacting the characteristics and composition of ambient aerosols over the marine region. Even though several studies have been attempted previously to characterize and quantify EC and OC concentration over polluted urban regions,2,27,48–50 high altitude sites,51–53 and relatively pristine island locations28 from the Indian sub-continent and surrounding, very limited studies have been documented on the comprehensive characterization of carbonaceous species over the North Indian Ocean,54–56 particularly over the BoB.38,40 Efforts have been made to quantify BC concentrations over the North Indian Ocean and assess its radiative impact over the oceanic region.57–60 Sudheer and Sarin38 made the first attempt to report on the distribution of EC and OC over the BoB during the late winter monsoon and found a significant impact of downflow wind on the spatial distribution of the carbonaceous species within the marine boundary layer. Another study by Srinivas et al., 2013,40 reported the concentration of carbonaceous species, including BrC, for the first time, over the BoB and suggested their origin mostly from biomass burning emissions in the IGP and SEA. Recently, Lee and Wang61 reported a significant impact of biomass burning on convective systems over the maritime continent which weakens the nocturnal rainfall intensity by about 9%. On the other hand, Ding et al.19 reported that the semi-direct effect of biomass burning aerosols on low cloud formation is enhanced by the Asian monsoon. Thus, considering the role and significance of carbonaceous species, a comprehensive characterization of their absorption properties and spatial distribution over the marine region is highly needed, particularly during the continental outflow period. So far, BrC data from only three research cruises have been reported from the BoB, which provides a snapshot picture of the distribution pattern of carbonaceous species.
In this study, we collected samples from two campaigns, in different time periods, (i) at the beginning of the northeast monsoon and (ii) at the end of the northeast monsoon, covering a large area of the BoB. The crop residue burning is prominent in North India during late October and early November,49 and has significant potential to impact the aerosol loading in this region,44 which makes this study more relevant. The 2nd campaign is during February, when continental outflow mediated by the northeast monsoon is significant. Thus, this study highlights the distribution of carbonaceous species in varying monsoonal wind regimes. Here we report the spatial distribution of EC and OC as well as the OC/EC ratio in the marine atmospheric boundary layer (MABL) of the BoB during winter months. Besides, we have also measured the water-soluble organic carbon (WSOC) and estimated secondary organic carbon to assess the role of secondary processes associated with long-range transport. Along with the OC/EC ratio, we have used various proxies such as ratios of OC and EC with nss-K+ and nss-SO42− as diagnostic tracers to decipher the contribution from biomass vis-à-vis fossil fuel burning emissions. Further, we also discussed the absorption properties of water-soluble carbonaceous species (or BrC) and their contribution towards the regional radiative forcing over the BoB.
2. Materials and methods
2.1 Cruise track and regional meteorology
Bulk aerosol samples were collected during two cruises onboard ORV Sindhu Sadhana SSD-015 and SSD-019 during October 2015 and February 2016, respectively. Typically, MABL of the BoB is strongly influenced by the continental outflow during our study period. It experiences a sharp seasonal reversal of winds due to a strong land-ocean thermal gradient.62 The wind system changes from south-westerlies during the southwest monsoon to predominantly north-easterlies during the northeast monsoon. North-easterlies, blowing towards the ocean from polluted continental landmass during wintertime, transport a significant amount of aerosols over the BoB. Being a transitioning month, October experiences relatively weaker winds compared to the rest of the season. These winds transport a complex mixture of aerosols that contains biomass and fossil fuel burning products, mineral dust, sea-salt, and secondary particles such as sulfate, nitrate, and ammonium salts.35 The cruise track during October-2015 was mostly confined to the coastal region covering a latitudinal stretch between 13 °N and 21 °N (Fig. 1). However, the February 2016 cruise covered a larger area in the open oceanic region of BoB between 13–21°N and 80–90 °E (Fig. 1). Relative humidity, air temperature, surface pressure, and winds were monitored periodically on board ship at a height of 20 m above sea level. Relative humidity varied between 31% and 100%, with a mean of 73 ± 12% during October 2015 and between 56% and 96% with a mean of 77 ± 7% during February 2016. The corrected average wind speeds were 3.6 ± 2.9 m s−1 during October 2015 and 2.7 ± 1.7 m s−1 during February 2016, respectively. The surface-level pressure showed very low variability (1005–1013 mb), whereas, the temperature varied from 23 °C to 32 °C.
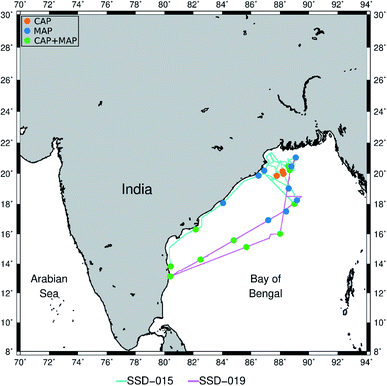 |
| Fig. 1 Cruise tracks of SSD-015 and SSD-019 during October 2015 and February 2016. The colour filled circle shows the midpoint of the sampling track used for one sample. Different colours indicate samples impacted by different air masses discussed in the text. | |
2.2 Sample collection and air-mass based classification
PM10 (particulate matter less than 10-micron aerodynamic diameter) aerosol samples (n = 10 and 11 for SSD-015 and SSD-019, respectively) were collected onboard using high volume samplers (Envirotech HVS APM-460). Pre-combusted PALLFLEX™ tissue quartz filters (20 × 25 cm2) were used for aerosol collection. The sampler was installed on the top deck of the ship, 20 m above the sea surface. Each sample was collected for a period ranging from 12–24 h (typically starting at 08:00 hours, local time) at a flow rate of 1.1 m3 min−1. The samples were collected only when the ship was cruising at a speed of 10 knots or above, ensuring that the winds were from the foreside of the ship and contamination from the ship's exhaust did not affect the sampling.36,38 Subsequent to sampling, all filters were wrapped in aluminum foil and stored under refrigeration (−19 °C) until analysis. Blank filters (field blanks) were also handled in a similar way to check for any contamination during the sampling procedure. All samples were equilibrated to room temperature for about 2–3 hours before the weight determination. The mass load of PM10 was determined gravimetrically by taking the difference of filter weights before and after sampling (by equilibrating at a relative humidity of 45 ± 5% and a temperature of 24 ± 1 °C). Repeated measurements of the filter weights yielded an uncertainty of ±1 mg in the measured sample weight.
Seven days air mass back trajectories (AMBT) were computed at 500 m above sea level, using the Hybrid Single-Particle Lagrangian Integrated Trajectory model (HYSPLIT-version 4; GDAS data set,63,64) from the National Oceanic and Atmospheric Administration (NOAA) air resources laboratory for each sampling day. All computed trajectories ended at the mid-point of transect covered for the collection of each sample (shown in Fig. S1†). The mid-point of every sampling transect is shown as a different filled coloured circle, showing distinct wind regimes (discussed later in this section) on the cruise tracks in Fig. 1. We observed that the mixed nature of trajectories derived either from land or from ocean ending at representative sampling locations (Fig. S1†). Based on different wind regimes, we identified three major air parcels: (1) those originating from land and spent less than a day over the ocean to reach the sampling transect; we label them as the continental air parcel (CAP), (2) those that spent a majority of the time (more than one day) over the ocean before reaching the sampling transects labeled as the marine air parcel (MAP), and (3) those that traveled more than two days over both ocean and land before reaching our representative sampling transect, defined as a mix of CAP and MAP (CAP + MAP). Accordingly, the samples were classified into these categories, wherein we found three samples of CAP, nine samples of MAP, and ten samples of CAP + MAP. We have used this distinction to discuss the distribution and variation of carbonaceous species over the BoB in this study. Due to the limited number of observation days over the marine region, we could get very limited samples for the CAP regime, which may not be as significant as the other two air masses; however, we have attempted to discuss the relative contribution of three different air masses in affecting the carbonaceous aerosol composition and properties over the BoB.
2.3 Sample analysis
A sub-sample punch (1.5 cm2 area) was taken from each sample, on a clean flow bench, for analyses of carbonaceous species (EC and OC). Their concentrations were measured on a Sunset EC-OC analyzer following the NIOSH-5040 Total Optical Transmission (TOT) protocol which is widely used and well adopted worldwide.2,65,66 More details related to EC and OC measurements including field blank corrections and detection limits are discussed in the ESI-1.† The concentrations of OC and EC in marine aerosols from this study were corrected by field blanks. Typically, OC measured in these filters is subjected to positive and negative artifacts because of adsorption and desorption of volatile and semi volatile organic compounds (VOCs and SVOCs), respectively.67 By using backup filters (and its correction) and denuders during active sampling, the OC overestimation can be reduced.67 In this study, we have not applied such corrections. However, we used field blanks, which were kept in a sampler for 12–15 h without pumping air, for correction. The contribution of ambient VOCs through condensation on the aerosol filter substrate is more prevalent in the urban/continental locations.68 In addition, Watson et al.69 showed that artifact corrections are less important for samples with OC concentrations more than 20 μg per filter. In our study, all samples have higher OC than 20 μg, suggesting relatively lower positive artifacts. Nevertheless, the OC concentration reported in this study provides an upper limit of the measurement. WSOC was measured in aerosol filters using a Shimadzu TOC-LCPH analyzer70 following the procedure detailed in the ESI 1.† In addition, inorganic constituents (Na+, K+ and SO42−) were measured by using an ion chromatograph (Dionex ICS-5000) as detailed in Kumar et al.51
To study the optical characteristics of the aerosol samples, we estimated the absorption coefficient (babs-365) as well as absorption efficiency by measuring the absorption signal of WSOC for the wavelength range of 200–800 nm. An aliquot of the water extract was used to measure absorbance using a Shimadzu UV-Vis spectrophotometer. Blank filter extracts were used as a reference to correct the absorption values.
Absorption coefficient (babs-365) was calculated as described in Hecobian et al.,71 and is expressed as:
|  | (1) |
where
A365 and
A700 are the absorbance values recorded using a spectrophotometer at 365 and 700 nm, respectively,
Vext is the volume of Milli-Q water used to extract WSOC (50 mL),
Vaero is the volume of the air filtered during sampling,
l is the path length of the cuvette (1 cm) and
M = 10
6. At 700 nm, it is assumed that there is no contribution from OC towards absorbance. Absorbance at 365 nm has been used as a measure of absorbance of all chromophoric aerosol constituents while making sure that the wavelength chosen was far enough from the UV region to avoid interference from non-organic compounds.
Mass absorption efficiency of BrC aerosols (MAEBrC) was calculated using estimated babs-365 and the measured mass concentration of WSOC.40,71
| 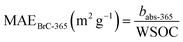 | (2) |
2.4 Estimation of secondary organic carbon
The EC tracer method has been used to compute the concentration of secondary organic carbon (SOC) in our study.51,72–74 In this method, the EC fraction is presumed to be derived from primary high-temperature combustion processes while the OC fraction is derived from primary emissions as well as formed via various secondary processes including photochemical reactions of gaseous organic matter and oxidation of volatile organic compounds.72 At first, primary OC is calculated using a primary OC/EC ratio, which is derived considering EC as a tracer for primary emissions of carbonaceous aerosols using the following equation: | OCmeasured = OCprimary + OCsecondary | (3) |
Rewriting OC primary after accounting for the primary nature of EC,
| OCprimary = (OC/EC)primary × ECmeasured | (4) |
Minimum values of OC/EC ratios for each regime are used as (OC/EC)primary values, which are likely to be of primary origin, and thus act as the first-order approximation to calculate SOC.75
| OCsecondary = OCmeasured − [(OC/EC)minimum × ECmeasured] | (5) |
However using the EC-tracer method, the calculated SOC has uncertainties due to the following reasons: (i) there may be considerable SOA formation when minimum OC/EC is observed; (ii) single data point may not be a true representation of the primary emission ratio of the season and (iii) emission sources of carbonaceous species may include varying biomass burning with higher OC/EC ratios. Even though the absolute values of SOC may be underestimated due to SOA formation when a minimum OC/EC ratio was observed, daily variability of SOC can be quantitatively represented with this calculation.
2.5 Carbonaceous species-mass absorption efficiency of EC
We need to translate our bulk solution (i.e., water extract) based MAEBrC to its particle nature in order to compare with other strongly absorbing EC aerosols over the BoB.24 Assuming spherical particles and using Mie theory, Liu et al.76 suggested that the absorption properties of BrC aerosols from the solvent extracts (e.g., water and methanol) can lead to underestimation by a factor of two when compared to their particle characteristics. In contrast, Sun et al.77 used a conversion factor of 0.7 to estimate the absorption efficiency of BrC for small spherical particles, whose dimensions are far less than the wavelength of incident light. For the field studies, focusing on the continental outflow to the northern Indian Ocean, an intermediate conversion factor i.e., ‘1’or unity times the MAEBrC has been used.78 Therefore, we have adopted this conversion factor of unity times the MAEBrC to obtain that of the aerosol particles over the BoB in this study. Subsequently, we compared directly the MAEBrC with that of EC estimated based on the light-extinction data obtained from the Sunset carbon analyzer according to the analytical protocol detailed by Ram and Sarin.79
The light-attenuation signal (ATN) at a wavelength (λ) of 678 nm was retrieved from the Sunset carbon analyzer, which corresponds to the EC absorption in the filters. The ATN signal of EC is normalized with the filtered air volume (Vair: 800–1664 m3) and integrated with the total filter area (Afilter: 417 cm2) to obtain the attenuation coefficient (bATN-EC-678, expressed in Mm−1; M = 106; eqn (6)). However, this estimate of bATN-EC-678 is affected by two inherent sampling artifacts, the scattering effect (C) and shadowing effect (R(ATN)). We corrected bATN-EC-678 for C and R(ATN) using eqn (7) to obtain the absorption coefficient of EC (babs-EC-678) over the BoB in the studied aerosol samples. However the coating of the EC core with sulfates results in an enhancement in the absorption because of the so-called “lensing effect”. Ram and Sarin79 reviewed the literature and proposed that a factor of “2.1” and “3.6” could best represent the externally (i.e., over source emissions) and internally (i.e., over receptor sites) mixed EC aerosols. As our study region is far away and downwind of the IGP-outflow, we used a value of 3.6 for ‘C’. Likewise, ‘R(ATN)’ is the result of EC particles embedded in the filter depths shadowed by other nonabsorbing particles and, therefore, contributes less absorption than the true EC signal if not accounted for this effect. Weingartner et al.80 proposed an empirical relationship for the filter-based absorption of BC particles on an Aethalometer to account for the shadowing effects (eqn (8)). Ram and Sarin79 proposed to use this empirical relationship by Weingartner and co-workers for the EC absorption data on a Sunset carbon analyzer.
| bATN-EC-678 (Mm−1) = ATNEC-678 × Afilter/Vair | (6) |
| babs-EC-660 (Mm−1) = bATN-EC-660 (Mm−1)/[C × R(ATN)] | (7) |
| R(ATN) = (1/f − 1) [(ln(ATN) − ln(0.1))/(ln(0.5) − ln(0.1))] + 1 | (8) |
In eqn (8), R(ATN) approaches unity for ATN ≤0.1 but less than unity if ATN >0.1. Here, we used f = 1.103 for the wintertime aerosols from the IGP-outflow to the BoB, similar to earlier studies.81,82 We deduced MAEEC-678 by taking the ratio of babs-EC-660 with the ambient EC loadings (in μg m−3) over the BoB, as described in eqn (9).
| MAEEC-678 (m2 g−1) = babs-EC-678 (Mm−1)/[EC (μg m−3)] | (9) |
2.6 Calculation of relative radiative forcing of BrC over EC
The fraction of light absorbed by the water-soluble BrC and EC was approximated using the wavelength dependence of MAEλ on the absorption Angstrom exponent (AAE) as per eqn (10) | MAEλ = MAEλ0 × (λ/λ0)−AAE | (10) |
Since EC is a strongly absorbing carbonaceous component than BrC, it is more often a common practice of the researchers to express the relative radiative forcing of BrC (RRFBrC), which is according to methods initially described by Kirillova et al.83 and references therein. The RRFBrC is simply the ratio of the amount of sunlight absorbed by BrC relative to EC across the full solar emission spectrum (I0(λ)) between wavelengths 300 and 2500 nm based on the Lambert–Beer law (see eqn (11) and (12)). Here we used the clear sky Air Mass 1 Global Horizontal (AM1GH) solar irradiance model by Levinson et al.84 Since this number is small, we expressed the RRFBrC values in percentage contribution relative to EC.
|  | (11) |
| 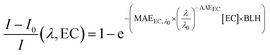 | (12) |
In eqn (11) and (12), BLH represents the atmospheric boundary layer height (BLH) and assumed a value of 1000 m for this study. Likewise, [WSOC] and [EC] correspond to their respective mass concentrations in ambient aerosols collected over the BoB. Besides, we chose the AAE of EC as ‘1.0’ to compare our estimates with those of others from the northern Indian Ocean conducted during the impact of continental outflow. Furthermore, we measured the BrC absorption at 365 nm and EC absorption at 678 nm. Hence, λ0 refers to 365 nm for BrC and 678 nm for EC in this study. We have estimated the fractional contribution of the amount of BrC absorption across the clear sky full solar spectrum (I0(λ)) between wavelengths 300 and 2500 nm (ref. 84) over that of EC (eqn (12)). Since this number is small, we expressed the RRFBrC values in percentage contribution relative to EC.
3. Results and discussion
3.1 Distribution of aerosol mass and carbonaceous species concentration over the BoB
Based on the above air mass back-trajectory classification, the concentration of OC, EC, WSOC, nss-K+, and mass load is presented in the form of Box–Whisker plots (Fig. 2). The maximum, minimum, average, and standard deviation (±1σ) values of the samples are also summarized in Table 1.
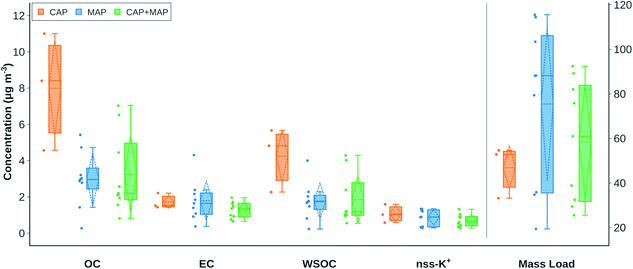 |
| Fig. 2 Box–Whisker plot showing variations in the concentration of OC, EC, WSOC, nss-K+ and PM10 mass load (in μg m−3) for samples represented by different air masses (CAP, MAP and CAP + MAP; see the text for details). The box represents the lower and upper quartile, while the whiskers represent lower and upper extremes. The dots outside the box represent the data spread. Solid and dotted lines inside the box represent median and mean, respectively. Lengthwise solid lines represent the standard deviation. | |
3.1.1 Total mass loading and total carbonaceous aerosol mass.
The PM10 mass loadings (in μg m−3) were found in the range of 33.2 to 54.7 (mean: 46.9 ± 11.9) in CAP, 19.4 to 115.6 (mean: 75.5 ± 36.4) in MAP, and 25.5 to 92.4 (mean: 58.5 ± 27.3) in CAP + MAP regimes. The highest average mass load is found in MAP followed by CAP + MAP and CAP samples. This is conspicuous, as CAP samples were collected near the coastal region and expected to have relatively higher aerosol load being near to continental emission sources. However, we observed high mass for MAP samples with large variability. The observed mass load in CAP is comparable with PM10 mass reported by Srinivas et al.85 and higher than that reported by Sudheer and Sarin.38 While analyzing the water-soluble composition, we observed very high sea-salt (calculated using Na+ and Cl− concentrations;35) contribution in MAP (sea-salt = 4.7 ± 1.5 μg m−3) and CAP + MAP (sea-salt = 2.3 ± 2.8 μg m−3) samples compared to CAP samples (sea-salt = 0.7 ± 0.5 μg m−3). Sea-salts are mainly produced under high wind conditions, which are prevalent in the open ocean region.58 Using estimated particulate organic matter (POM) and EC, we calculated the total carbonaceous aerosol (TCA) concentration to assess the percentage contribution of carbonaceous species to the total PM10 mass load. POM is estimated from organic carbon (OC) multiplied by a factor of 2.1.35 The factor 2.1 is an estimated average of the molecular weight per unit weight of carbon for the organic aerosols in the marine atmospheric boundary layer.86,87 A relatively higher concentration of TCA is found in CAP (18.5 μg m−3, 40% of total mass loading) than in MAP (8.0 μg m−3, 11% of total mass loading), and CAP + MAP (8.1 μg m−3, 11% of total mass loading) samples which could be mainly due to loss of OC via multiple gas-phase oxidation reactions during the long-range transportation.88
3.1.2 OC, EC, and WSOC mass concentrations and their variation.
The OC concentration varied from 4.6 to 11.0 μg m−3 (mean: 8.0 ± 3.2 μg m−3) in CAP, from 0.3 to 5.4 μg m−3 (mean: 3.0 ± 1.5 μg m−3) in MAP and from 0.8 to 7.0 μg m−3 (mean: 3.2 ± 2.2 μg m−3) in CAP + MAP samples. OC is also the dominant fraction of TCA in all three regimes (43% in CAP, 37% in MAP, and 40% in CAP + MAP, respectively). A relatively higher OC concentration was found in CAP than MAP and CAP + MAP (Fig. 2) which could be mainly attributed to the relative proximity of the source region (IGP) to samples collected in the CAP regime and also reflected from the AMBT (Fig. S1†). OC concentrations of MAP and CAP + MAP samples are comparable; however, it is observed that there is a decrease in the OC concentration mainly during transportation and it can be mainly due to the removal mechanism of OC.89–91 The OC values linked with long-range transport over the marine region as well as those observed from IGP outflow38,40,85 are comparable with our data (Table 1). A similar decrease in OC values was observed at remote locations which are far from the source region28,51,92–94 (Table 1). However, these values are significantly less than those reported for polluted source regions such as the IGP;95 urban area in the IGP (e.g. Kathmandu and Karachi96) and Delhi.27
Table 1 Comparison of various chemical species and diagnostic ratios. PM10 mass, OC, EC, WSOC, nss-SO42−, and nss-K+ (values are in μg m−3)
Study area |
PM10 mass |
OC |
EC |
WSOC |
nss SO42− |
nss K+ |
OC/EC |
WSOC/OC |
nss K+/EC |
nss K+/OC |
nss SO42−/EC |
nss SO42−/OC |
References |
BoB (CAP) |
46.9 ± 11.9 |
8.0 ± 3.2 |
1.7 ± 0.4 |
4.3 ± 1.8 |
15.7 ± 4.8 |
1.1 ± 0.5 |
4.6 ± 1.2 |
0.53 ± 0.04 |
0.6 ± 0.2 |
0.1 ± 0.01 |
9.3 ± 3.1 |
2.1 ± 0.4 |
This study |
BoB (MAP) |
75.5 ± 36.4 |
3.0 ± 1.5 |
1.8 ± 1.1 |
1.8 ± 1.0 |
15.7 ± 3.7 |
0.9 ± 0.5 |
1.7 ± 0.7 |
0.62 ± 0.12 |
0.6 ± 0.3 |
0.4 ± 0.4 |
12.7 ± 8.6 |
10.0 ± 13.0 |
BoB (CAP + MAP) |
55.5 ± 27.3 |
3.3 ± 2.2 |
1.3 ± 0.5 |
1.8 ± 1.4 |
16.2 ± 6.7 |
0.7 ± 0.4 |
2.6 ± 1.5 |
0.57 ± 0.10 |
0.5 ± 0.1 |
0.3 ± 0.2 |
14.5 ± 8.3 |
7.4 ± 4.7 |
North BoB (PM2.5) |
38.0 ± 20.2 |
5.9 ± 3.7 |
2.0 ± 1.3 |
|
|
|
2.9 ± 0.8 |
|
0.3 ± 0.1 |
|
|
|
Srinivas et al., 2011 (ref. 85) |
South BoB (PM2.5) |
22.3 ± 9.9 |
2.6 ± 1.9 |
1.2 ± 0.0.4 |
|
|
|
2.4 ± 1.3 |
|
0.3 ± 0.1 |
|
|
|
North BoB (PM10) |
57.8 ± 30.6 |
9.1 ± 6.0 |
2.7 ± 1.7 |
|
|
|
|
|
0.4 ± 0.1 |
|
|
|
South BoB (PM10) |
32.2 ± 15.1 |
3.9 ± 2.8 |
1.1 ± 0.5 |
|
|
|
|
|
0.5 ± 0.2 |
|
|
|
Entire BoB |
22 |
1.9 |
0.4 |
|
|
|
4.3 |
|
|
|
15 |
|
Sudheer and Sarin, 2008 (ref. 103) |
North BoB |
41 |
4.1 |
0.6 |
|
9 |
|
7.4 |
|
|
|
|
|
South BoB |
16 |
0.9 |
0.3 |
|
4.4 |
|
2.9 |
|
|
|
|
|
IGP out flow to BoB |
|
9.1 ± 6.0 |
2.7 ± 1.7 |
5.5 ± 3.1 |
|
|
3.4 ± 1.1 |
0.65 ± 0.11 |
|
|
|
|
Srinivas Sarin, 2013 (ref. 40) |
SEA out flow to BoB |
|
3.9 ± 2.8 |
1.1 ± 0.5 |
2.7 ± 1.7 |
|
|
3.2 ± 2.0 |
0.79 ± 0.17 |
|
|
|
|
Port Blair |
42 ± 11 |
6.3 ± 2.0 |
2.1 ± 0.0.9 |
3.0 ± 1.0 |
8.3 ± 3.9 |
0.5 ± 0.2 |
3.3 ± 0.9 |
0.5 ± 0.1 |
0.3 ± 0.1 |
|
|
|
Rastogi et al., 2020 (ref. 28) |
IGP biomass burning (day) |
181 ± 64 |
53 ± 21 |
6.5 ± 1.8 |
33.5 ± 11.3 |
24.5 ± 14.1 |
4.1 ± 1.5 |
8.1 ± 2.4 |
0.7 ± 0.1 |
0.6 ± 0.2 |
0.08 ± 0.02 |
3.9 ± 2.1 |
0.5 ± 0.3 |
Srinivas et al., 2016 (ref. 124) |
IGP biomass burning (night) |
239 ± 89 |
90 ± 49 |
7.9 ± 2.6 |
42.3 ± 21.1 |
21.9 ± 9.0 |
5.2 ± 2.5 |
11.3 ± 4.6 |
0.5 ± 0.1 |
0.7 ± 0.3 |
0.06 ± 0.01 |
3.1 ± 1.8 |
0.3 ± 0.15 |
Delhi |
177.9 ± 49.5 |
26.7 ± 9.2 |
6.1 ± 3.9 |
|
8.3 ± 3.8 |
1.4 ± 0.6 |
4.4 |
|
0.3 |
0.06 |
1.4 |
|
Rajput et al., 2014 (ref. 105) |
Mt. Abu winter |
|
3.5 ± 2.2 |
0.8 ± 0.6 |
2.6 ± 1.9 |
5.0 ± 2.3 |
0.4 ± 0.3 |
5.3 ± 1.4 |
0.7 ± 0.1 |
0.5 ± 0.1 |
0.1 ± 0.04 |
8.6 ± 4.0 |
1.7 ± 0.9 |
Kumar et al., 2016 (ref. 51) |
Mt. Abu summer |
|
1.6 ± 0.6 |
0.3 ± 0.1 |
0.8 ± 0.3 |
3.1 ± 1.0 |
0.1 ± 0.1 |
5.6 ± 1.7 |
0.5 ± 0.2 |
0.5 ± 0.2 |
0.1 ± 0.03 |
14.0 ± 6.8 |
2.2 ± 1.0 |
In comparison to OC, no significant variation was observed in the EC mass concentration during this study. EC varied from 1.4 to 2.2 μg m−3 (mean: 1.7 ± 0.4 μg m−3) in CAP, from 0.4 to 4.3 μg m−3 (mean: 1.8 ± 1.1 μg m−3) in MAP and from 0.7 to 2.0 μg m−3 (mean: 1.3 ± 0.5 μg m−3) in CAP + MAP samples. Such insignificant variation in EC concentrations suggests the transportation of soot particles (which is one of the subtypes of EC and mainly produced by fossil fuel combustion) which have relatively less gravitational settling than OC during long-range transport.89–91,97 The observed EC concentrations are comparable with those reported in earlier studies (Table 1),28,40,85 but are higher than those reported by Sudheer and Sarin.38 Sudheer and Sarin38 reported EC for samples collected during the late-March to mid-April period, wherein, the continental outflow is relatively weaker than that during the December–February period.36 This may be the reason for the lower EC concentration over the BoB reported by Sudheer and Sarin.38 The continental outflow during the winter season carrying carbonaceous aerosols from the IGP has significant fraction from post-harvest large-scale biomass burning in the IGP.38,95 Conspicuously, OC and EC values are high at the IGP95 and Delhi27 as the data have been collected near the source regions (Table 1). The concentration of OC and EC will decrease in the downwind region as compared to the source region mainly due to dispersal as well as removal (via gravitational settling) during transport.
The organic fraction of carbonaceous aerosols is often reported on the basis of its solubility in water, and major constituents of secondary organic aerosols are likely to be these water-soluble fractions of OC.56,98 In our study, WSOC was found to vary from 2.3 to 5.7 μg m−3 (mean: 4.3 ± 1.8 μg m−3) in the CAP; from 0.2 to 4.0 μg m−3 (mean: 1.8 ± 1.0 μg m−3) in MAP; and from 0.6 to 4.3 μg m−3 (mean: 1.8 ± 1.4 μg m−3) in CAP + MAP air parcel samples. The variation of WSOC was found to be similar to that exhibited by OC (Fig. 2). The concentration of WSOC in the CAP samples was found to be more than two times higher than that in the MAP and CAP + MAP samples, which further supports the influence of long-range transport of carbonaceous species from the IGP. A similar variability in the WSOC concentration is reported by Srinivas and Sarin40 over the BoB. The dependence of concentration with proximity to the source region is also supported by significantly higher values observed for the IGP region.95 The fractional contribution of WSOC to total OC is parameterized as the WSOC/OC ratio and its variation for all three types of wind regimes is shown in Fig. 3. The average WSOC/OC value is relatively higher (with large variability; 0.62 ± 0.12) for parcels that have spent a significant amount of time in the marine region (MAP) than those having larger contribution from continents (0.53 ± 0.04 for CAP and 0.57 ± 0.10 for CAP + MAP). In contrast to our observation, Srinivas and Sarin99 reported relatively higher WSOC/OC (around 0.7) ratios over Kharagpur, located in the southeast of the IGP and very close to the coast of the BoB (Table 1). The higher value over this region is mainly attributed to transport a significant amount of carbonaceous aerosols from the IGP mainly emitted from crop residue/biomass burning towards the downwind flow region during the winter season.44,95,100
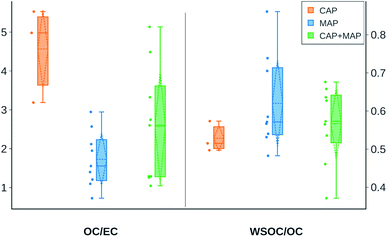 |
| Fig. 3 Box–Whisker plot showing variations in diagnostic ratios of OC/EC and WSOC/OC, which are used to decipher sources of carbonaceous aerosols, for CAP, MAP and CAP + MAP. The box represents the lower and upper quartile, while the whiskers represent lower and upper extremes. The dots outside the box represent the data spread. Solid and dotted lines inside the box represent median and mean, respectively. Lengthwise solid lines represent the standard deviation. | |
The relative concentration of OC and EC (also referred to as the OC/EC ratio) is important for the quantification of the impact of carbonaceous species (scattering vs. absorption).101 Typically, OC/EC ratios have been widely used to identify emission sources (biomass or fossil fuel burning) of carbonaceous species101,102 and hence it is important in the climate model for the computation of direct radiative forcing. The mean value of OC/EC is found maximum in the CAP (4.6) particulate samples followed by CAP + MAP (2.6) and MAP (1.7) and is shown in Fig. 3. The observed OC/EC ratio further confirmed the impact of biomass burning on the CAP samples as discussed earlier and comparable to the earlier studies over the BoB as well as other coastal locations.40,81,103 Previous studies have attributed to higher values of OC/EC (typically greater than 2) for biomass burning emissions and a lower value (less than 2) for fossil fuel burning emissions.28,104,105 During long-range transport of carbonaceous species, OC is preferentially removed, mainly due to the ageing effect wherein it undergoes oxidation or evaporative loss, compared to EC.91 Such preferential removal of OC and relative inertness of EC lead to lower OC/EC values for air parcels that are sampled in the remote location or as we move away from the landmass (source region). We also noticed a significant correlation between OC and EC for CAP (r = 0.86) and MAP (r = 0.70) compared to a moderate correlation for CAP + MAP samples (r = 0.42) (Fig. 4), indicating a common source of emission for OC and EC as well as primary nature of OC in CAP and MAP samples. It is surprising to observe a relatively better correlation in MAP samples compared to CAP + MAP, as there are no common emission sources of EC and OC in the marine region. In addition, WSOC shows a significant correlation with OC irrespective of the origin of the air parcels (r = 0.99, 0.94, and 0.98, for CAP, MAP and CAP + MAP, respectively), indicating the primary nature of WSOC (Fig. 4).
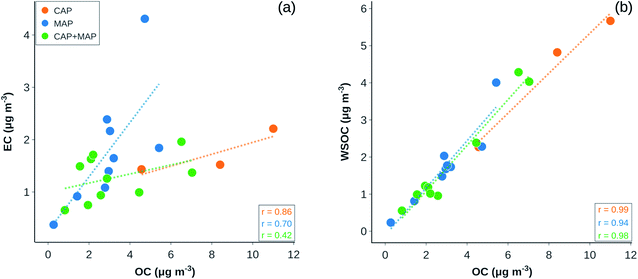 |
| Fig. 4 Relationship between (a) EC and OC and (b) WSOC and OC for aerosol samples collected over the BoB during different wind regimes (CAP, MAP and CAP + MAP). | |
Source identification using the OC/EC ratio is difficult, particularly for samples collected at remote locations, mainly due to associated complex physical and chemical processes.51 However, the OC/EC ratio along with other diagnostic parameters such as the nss-K+ concentration and nss-K+/OC and nss-K+/EC ratios have been used successfully for source identification.97,106–109 In this view, we further investigated the distribution of nss-K+ (considered as a tracer of biomass burning emission sources104) and of nss-SO42− (considered as a tracer of fossil fuel burning emission sources;106,110,111) and computed the correlation with carbonaceous species. CAP samples show an excellent correlation between OC and nss-K+ (r = 0.98, Fig. S2†), while a moderately better correlation is observed for CAP + MAP samples (r = 0.66, Fig. S2†). Similarly, nss-K+ has a very good correlation with EC for CAP and CAP + MAP (r = 0.94 and 0.85, respectively) samples while it shows a moderate correlation (r = 0.49) for MAP samples. On the other hand, nss-SO42− is poorly correlated with EC and OC (Fig. S3†) with an exception of OC in the CAP regime. These observations suggest that the OC and EC fractions of CAP and CAP + MAP samples are mainly derived from biomass burning emissions. These observations are consistent with previous studies reported over the BoB,28,38,40,100 which have highlighted the dominance of biomass burning emissions to carbonaceous aerosols. In addition to this, we also evaluated fire count maps with a threshold confidence level (>80%) retrieved from the Moderate Resolution Imaging Spectroradiometer (MODIS) satellite. The satellite retrievals suggest that the samples from CAP and CAP + MAP are influenced by the observed fire hotspots (indicated by red dots) located in the IGP and SEA through the long-range transport (in Fig. S1†) which further confirms the significant contribution of biomass burning in aerosol loading over the BoB during this study. In an earlier study, Tiwari et al.44 also reported an elevated absorbing aerosol layer (carbonaceous aerosol; up to 4 km) over the BoB during winter through the long-range transportation from the IGP. Although, the OC/EC ratio is less than 2 for MAP samples (1.7 ± 0.7), we did not find any correlation between nss-SO42− and OC or EC (Fig. S3†), ruling out fossil fuel emission being the dominant source for TCA. It is important to reiterate here that a moderate correlation was observed between OC and nss-K+ (Fig. S2†), along with nss-K+/EC ratios falling between 0.2 and 1.1 (Table 1), typical of the biomass burning range104 for MAP samples. These observations suggest a possible contribution from mixed sources (fossil fuel and biomass burning emissions) over the BoB within MABL. The reported values and the source attribution are in agreement with earlier studies in the BoB (Table 1).28,40
3.2 Variation of secondary organic carbon over the BoB
Secondary organic aerosols (SOAs) are produced from the photochemical reaction of gaseous organic aerosols and then further condensing to the particle phase.112 The SOC concentration, computed using the EC-tracer method, varied from 3.9 to 9.9 μg m−3 (mean: 7.2 ± 3.1 μg m−3); 0.1 to 4.5 μg m−3 (mean: 2.1 ± 1.2 μg m−3) and 0.5 to 6.4 μg m−3 (mean: 2.6 ± 2.1 μg m−3) in CAP, MAP and CAP + MAP samples which accounts for ∼90%, 70% and 81% of total OC, respectively.
The estimated SOC values were found to be lower in those samples which are of marine origin (MAP) than in those that have continental air mass contribution. The fractional contribution of SOC to OC is slightly higher for air parcels that have traveled exclusively over the continental region. SOC reduces as we move from the source region (landmass) to remote marine locations. The IGP and SEA emit a large quantity of pollutants via emission from vehicles, industries, large-scale post-harvest biomass burning, and biofuel burning.100 The enhanced photochemical activity over these polluted regions facilitates the formation of secondary organic aerosols.100 This could explain the relatively higher contribution of SOC to OC in the vicinity of the source region. Further, in our study, we observed a good correlation between SOC and WSOC for CAP, MAP, and CAP + MAP (r = 0.99, 0.96, and 0.97, respectively, Fig. 5). This observation points to the similar chemical nature of SOC and WSOC as well as the dominant contribution of SOC to WSOC for the samples collected during these two cruises in the BoB. SOC concentrations reported for the remote marine region were higher than those observed over pristine continental regions such as Mt. Abu, Rajasthan51 and Manora Peak, Uttarakhand.52 The percentage contribution of SOC to OC in these studies (∼30–50%) is also relatively lower than that observed in our study (>70%). This could be due to possible secondary organic aerosol contribution from the marine region.
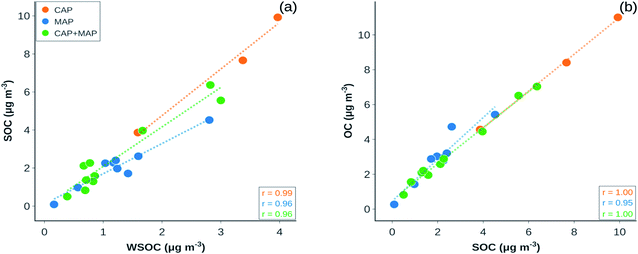 |
| Fig. 5 Scatter plots between (a) SOC and WSOC and (b) OC and SOC over the BoB during winter continental outflow. | |
3.3 Absorption characteristics of WSOC
The relative absorbance of WSOC at 365 nm compared to that at 700 nm is credited to “BrC”.24,71,113 BrC contains water-soluble and water-insoluble chromophores; however, about 70% of the BrC chromophores can be extracted by water.24 A growing amount of data supports that BrC may contribute substantially or even dominate the total aerosol absorption at specific wavelengths,24,40,114,115 particularly over the Indian subcontinent and adjoining marine region.116 This absorbing fraction has potential to significantly influence global radiative forcing.117–119 Weber et al.120 highlighted that BrC could contribute up to 48% to the top of the atmosphere average clear sky instantaneous forcing by light absorption aerosol extinction of ultraviolet sunlight and may reduce tropospheric ozone concentrations as well as lead to a significant reduction of atmospheric oxidants via reduced photolysis rates of photochemically active gaseous compounds.24,26,121,122
In this study, we measured the absorbance of WSOC chromophores of each sample using a UV-Vis spectrophotometer in a wide wavelength range from 200 to 800 nm. Using the difference of absorption values at 365 and 700 nm, we calculated the light absorption coefficient at 365 nm i.e. babs-365 following eqn (1) discussed in section 2. A distinctly higher babs-365 was observed for CAP (3.68 ± 1.23 Mm−1) samples as compared to rest two sample groups having nearly similar values (i.e. MAP: 0.92 ± 0.41 Mm−1 and CAP + MAP: 0.93 ± 0.36 Mm−1). Except CAP samples, babs-365 values over the BoB are relatively lower than those reported for the IGP (2.2 Mm−1) and SEA (1.4 Mm−1) outflow over the BoB40 (Table 2), as well as those in the urban sites (1.8 Mm−1) during summer located in China (Beijing123) and significantly lower than the values reported for biomass burning source regions in rural India ((40–52 Mm−1);124Table 2). Although, babs-365 for CAP and other set of samples shows distinct values, we observed a significant correlation for WSOC (r = 0.98, 0.78, and 0.83 for CAP, MAP and CAP + MAP, respectively; Fig. 6a) and nss-K+ (r = 0.86, 0.64, and 0.38 for CAP, MAP and CAP + MAP, respectively; Fig. 6b) versus babs-365. These observations indicate their common sources (biomass burning emissions) and common transport pathways. Similarly, a significant correlation was observed between WSOC and babs-365 in previous studies over urban71,116,125–127 as well as remote, pristine regions.116 In addition, Bikkina and Sarin116 demonstrated that biomass burning emission in the IGP dominantly contribute to BrC during continental outflow to the North Indian Ocean, particularly during the paddy burning season, attest to our observations during this study.
Table 2 Comparison of absorption coefficients (in Mm−1), absorption efficiencies (in m2 g−1), and Angstrom exponents with other studies
Study area |
b
abs-365
|
MAEBrC-365 |
AAEBrC |
References |
Bay of Bengal |
CAP |
3.68 ± 1.23 |
0.87 ± 0.07 |
5.11 ± 0.31 |
This study |
MAP |
0.93 ± 0.41 |
0.56 ± 0.15 |
4.95 ± 0.60 |
CAP + MAP |
0.93 ± 0.36 |
0.88 ± 0.84 |
5.22 ± 0.34 |
Indo-Gangetic Plain |
|
11.4 ± 4.8 |
0.78 ± 0.24 |
6.0 ± 1.1 |
Srinivas and Sarin, 2014 (ref. 99) |
Bay of Bengal |
IGP outflow |
2.2 ± 1.3 |
0.4 ± 0.1 |
9.1 ± 2.5 |
Srinivas and Sarin 2013 (ref. 40) |
SEA outflow |
1.4 ± 1.1 |
0.5 ± 0.2 |
6.9 ± 1.9 |
Patiala |
Day |
40 ± 18 |
1.3 ± 0.7 |
5.1 ± 1.9 |
Srinivas et al., 2016 (ref. 124) |
Night |
52 ± 27 |
1.3 ± 0.5 |
5.3 ± 2.0 |
Delhi |
|
— |
1.6 ± 0.5 |
5.1 ± 2.0 |
Kirillova et al., 2014 (ref. 130) |
Karachi |
|
|
0.97 ± 0.37 |
|
Chen et al., 2020 (ref. 26) |
Beijing, China |
Summer |
1.8 ± 0.2 |
— |
7.5 ± 0.9 |
Cheng et al., 2011 (ref. 123) |
Winter |
0.7 ± 0.2 |
— |
7.0 ± 0.8 |
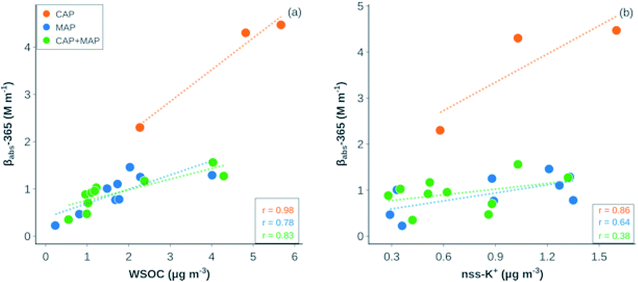 |
| Fig. 6 Scatter plots between (a) absorption coefficient at 365 nm and WSOC mass and (b) absorption coefficient at 365 nm and nss-K+ over the BoB during winter continental outflow. | |
The wavelength dependence of BrC absorption in a power relation is useful for understanding the influence of source-emissions and processing (or aging effects) during transport. The power exponent (babs-365 ∝ λ−AAEBrC) is referred as the absorption angstrom exponent (AAEBrC), which was obtained from the wavelength range 360–370 nm. The AAEBrC for the three different types of marine aerosols was analyzed and found in the range of 4.89 to 5.46 (mean = 5.11 ± 0.31), 4.66 to 5.70 (mean = 5.22 ± 0.34), and 4.31 to 6.02 (mean = 4.95 ± 0.60) for CAP, CAP + MAP, and MAP, respectively. These AAEBrC values from the present study (i.e., October, 2015 and February, 2016) in the BoB are comparable to those of earlier observations from this marine basin (5.7 ± 0.8) and the Arabian Sea (5.9 ± 1.8) that had the impact of biomass burning emissions in the IGP.128 Another diagnostic property of BrC aerosols specific to source emissions and processing effects is the mass absorption efficiency (MAEBrC-365). The estimated range of MAEBrC-365 for the CAP type (0.79–0.93 m2 g−1) overlap with that of CAP + MAP (0.30–0.92 m2 g−1) and MAP types (0.32–0.80 m2 g−1). These MAEBrC-365 values are also consistent with the earlier observations (0.3–1.5 m2 g−1) over the Arabian Sea and BoB during a fall season cruise (November, 2008) as well as the winter cruise data in the BoB (0.4–0.7 m2 g−1).40,116 In addition, our MAEBrC-365 values were also comparable with that of earlier observations from the North Indian Ocean, as well as those observed at a costal location in the Arabian Sea (Karachi: 0.97 ± 0.37 m2 g−1),26 however, relatively lower than those documented from the emission source region e.g. Patiala, Punjab:124 0.3–3 m2 g−1; Delhi:83 1.1–2.7 m2 g−1; Kanpur:129 1.8 m2 g−1; Kharagpur:125 0.2–1.5 m2 g−1. A recent study of the BoB during the southwest monsoon showed influence of oceanic derived BrC aerosols (0.16–1.45 m2 g−1) emitted along with sea-salt particles.82 Given the predominant oceanic air masses sampled in this study from the BoB with the similar cruise tracks of Bikkina et al.,82 it is likely to have a contribution of oceanic derived BrC aerosols for the MAP and CAP + MAP types.
3.4 Relative radiative forcing of BrC over EC
We have estimated the relative radiative forcing of water-soluble BrC over EC (RRFBrC) following the procedures detailed in earlier studies.83,116,125,130 This involves the normalization of the integrated amount of radiation absorbed by water-soluble BrC over that of EC across the full solar spectrum (300–2500 nm).
Fig. 7 shows the estimated RRFBrC for three types of samples wherein the CAP samples (1.5–2.4%) are slightly higher than those of the (CAP + MAP) type (0.7–1.9%) and MAP type samples (0.5–1.3%). These RRFBrC estimates are lower than those reported over the biomass burning influence source regions in the IGP (Patiala: 40%,124 Delhi: 10%,83 Kanpur: <24%;129 Kharagpur: <34% (ref. 125)). However, our estimates of RRFBrC over the BoB from this study (October 2015 and February 2016) are consistent with the earlier observations from the North Indian Ocean during winter (2 ± 1%;116 1% (ref. 78)). In contrast, our RRFBrC values are lower than the crop-residue burning influenced BrC aerosols from the Arabian Sea (8 ± 4%) and the BoB (12 ± 4%) during the fall season (November 2008).116 Although our present cruise (October 2015 and February 2016) coincide with a period of open burning of postharvest crop-residues in the northwest IGP and also influenced by air parcels originating from the IGP (referred here as CAP), we observed rather low RRFBrC (1–3%) than those obtained from the late fall season samples (November 2008).116 One reason could be the dilution of continental BrC aerosols with predominant oceanic air masses during our present cruise than that observed by Bikkina and Sarin.116
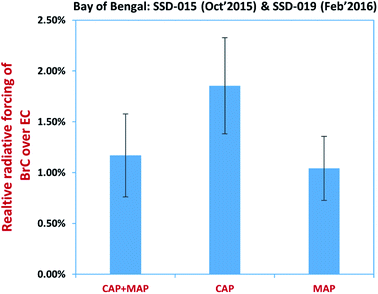 |
| Fig. 7 Relative radiative forcing of BrC over EC aerosols integrated between wavelengths 300 to 2500 nm using the clear sky air mass 1 global horizontal flux (I0(λ)) over the Bay of Bengal. | |
4. Summary
The spatio-temporal distribution of carbonaceous species was studied during the continental outflow season over the BoB. A large variability is observed in the mass concentration of carbonaceous species which were more affected by air masses derived from continents (CAP and CAP + MAP) compared to those which are affected by marine air masses (MAP). OC is well correlated with EC for CAP, CAP + MAP and MAP, indicating a common emission source. Significant correlations were observed between WSOC and OC irrespective of the origin of the air parcels. Along with the OC/EC ratio, several diagnostic ratios were investigated to identify the sources of carbonaceous species. OC/EC, nss-K+/OC, and nss-K+/EC along with the absorption angstrom exponent indicate the dominance of biomass burning emission sources in this study. These observations were also supported by the fire counts retrieved from MODIS, wherein air masses of CAP and CAP + MAP were observed to traverse over biomass burning hotspots located in the IGP and SEA. Even though the OC/EC ratio for the MAP regime was less than 2, these samples showed a poor correlation with the fossil fuel burning tracer, nss-SO42−. However, the samples showed a moderate correlation with nss-K+. Further, the nss-K+/EC ratio and absorption coefficient values indicate a mixed contribution from fossil fuel and biomass burning. Such observations underscore the need for more robust proxies (carbon isotopes) to assess sources of carbonaceous species.
In addition, we have used the EC tracer method to estimate SOC, which showed a good correlation with WSOC suggesting similar chemical behaviors during the study period over the BoB. We found a relatively higher contribution of SOC to OC near source regions implying enhanced photochemical activity that readily facilitates SOC formation. The absorption coefficient (babs-365) and mass absorption efficiency (MAEBrC-365) of WSOC were estimated at 365 nm and their values corroborate to the dominance of biomass burning emissions. A relatively lower babs-365 was found for MAP and CAP + MAP samples compared to previously reported values over the North Indian Ocean, except CAP samples. However, MAEBrC-365 was observed within the range of those documented previously in the marine region adjoining the Indian subcontinent. We have also estimated the relative radiative forcing of water-soluble BrC over EC which were found to be relatively lower (0.5–2.4%) than those estimated previously from the BoB and Arabian Sea during the continental outflow period. The regional scale composition data of carbonaceous aerosols and their absorption properties are important to constrain uncertainty associated with future climate projections.
Author contributions
Gourav Nayak: writing – original draft, investigation, data curation, methodology, writing – review & editing. Ashwini Kumar: conceptualization, data curation, funding acquisition, investigation, project administration, resources, supervision, writing – original draft, writing – review & editing. Srinivas Bikkina: methodology, writing – review & editing. Shani Tiwari: writing – review & editing. Suhas S. Sheteye: methodology. A. K. Sudheer: methodology, writing – review & editing.
Conflicts of interest
There are no conflicts to declare.
Acknowledgements
Funding for this research was supported by the CSIR project GEOSINK and CLICNIO. G. N. and A. K. thanks the Department of Science and Technology, Govt. of India for providing financial support under the INSPIRE programme (INSPIRE Fellowship, INSPIRE Faculty scheme; IFA13-EAS-13) and SPLICE Program (Grant No. DST/CCP/Aerosol/85/2017(G)). We thank the Chief Scientist, Captain and crew members of SSD-15 and 19 for their support during sample collection. The authors gratefully acknowledge the NOAA Air Resources Laboratory (ARL) for the provision of the HYSPLIT transport and dispersion model (http://www.ready.noaa.gov) and the Land, Atmosphere Near real-time Capability for EOS (LANCE) system operated by NASA's Earth Science Data and Information System (ESDIS) for the provision of MODIS fire counts data (https://www.earthdata.nasa.gov/active-fire-data) used in this study. NIO contribution number is 6822.
References
- S. K. Satheesh and K. Krishna Moorthy, Radiative effects of natural aerosols: A review, Atmos. Environ., 2005, 39, 2089–2110 CrossRef CAS
.
- K. Ram and M. M. Sarin, Spatio-temporal variability in atmospheric abundances of EC, OC and WSOC over Northern India, J. Aerosol Sci., 2010, 41, 88–98 CrossRef CAS
.
- T. C. Bond, S. J. Doherty, D. Fahey, P. Forster, T. Berntsen, B. DeAngelo, M. Flanner, S. Ghan, B. Kärcher and D. Koch, Bounding the role of black carbon in the climate system: A scientific assessment, J. Geophys. Res.: Atmos., 2013, 118, 5380–5552 CAS
.
- Z. Li, W. K.-M. Lau, V. Ramanathan, G. Wu, Y. Ding, M. G. Manoj, J. Liu, Y. Qian, J. Li, T. Zhou, J. Fan, D. Rosenfeld, Y. Ming, Y. Wang, J. Huang, B. Wang, X. Xu, S.-S. Lee, M. Cribb, F. Zhang, X. Yang, C. Zhao, T. Takemura, K. Wang, X. Xia, Y. Yin, H. Zhang, J. Guo, P. M. Zhai, N. Sugimoto, S. S. Babu and G. P. Brasseur, Aerosol and monsoon climate interactions over Asia, Rev. Geophys., 2016, 54, 866–929 CrossRef
.
- A. Rana, S. Jia and S. Sarkar, Black carbon aerosol in India: A comprehensive review of current status and future prospects, Atmos. Res., 2019, 218, 207–230 CrossRef CAS
.
- M. O. Andreae and A. Gelencsér, Black carbon or brown carbon? The nature of light-absorbing carbonaceous aerosols, Atmos. Chem. Phys., 2006, 6, 3131–3148 CrossRef CAS
.
- V. Ramanathan and G. Carmichael, Global and regional climate changes due to black carbon, Nat. Geosci., 2008, 1, 221–227 CrossRef CAS
.
-
T. Stocker, D. Qin, G. Plattner, M. Tignor, S. Allen, J. Boschung, A. Nauels, Y. Xia, B. Bex and B. Midgley, IPCC, 2013: Climate Change 2013: The Physical Science Basis. Contribution of Working Group I to the Fifth Assessment Report of the Intergovernmental Panel on Climate Change, Cambridge Univ. Press, 2013 Search PubMed
.
- H. Horvath, Atmospheric light absorption—A review, Atmos. Environ., Part A, 1993, 27, 293–317 CrossRef
.
- M. Z. Jacobson, Strong radiative heating due to the mixing state of black carbon in atmospheric aerosols, Nature, 2001, 409, 695–697 CrossRef CAS PubMed
.
- C. Sorensen, Light scattering by fractal aggregates: a review, Aerosol Sci. Technol., 2001, 35, 648–687 CrossRef CAS
.
- T. C. Bond and R. W. Bergstrom, Light absorption by carbonaceous particles: An investigative review, Aerosol Sci. Technol., 2006, 40, 27–67 CrossRef CAS
.
- G. Shrestha, S. J. Traina and C. W. Swanston, Black Carbon's Properties and Role in the Environment: A Comprehensive Review, Sustainability, 2010, 2, 294–320 CrossRef CAS
.
- S. Sharma, M. Ishizawa, D. Chan, D. Lavoué, E. Andrews, K. Eleftheriadis and S. Maksyutov, 16-year simulation of Arctic black carbon: Transport, source contribution, and sensitivity analysis on deposition, J. Geophys. Res.: Atmos., 2013, 118, 943–964 CAS
.
- J. Haywood and O. Boucher, Estimates of the direct and indirect radiative forcing due to tropospheric aerosols: A review, Rev. Geophys., 2000, 38, 513–543 CrossRef CAS
.
- Y. Yang, H. Wang, S. J. Smith, P.-L. Ma and P. J. Rasch, Source attribution of black carbon and its direct radiative forcing in China, Atmos. Chem. Phys., 2017, 17, 4319–4336 CrossRef CAS
.
- S. Kang, Q. Zhang, Y. Qian, Z. Ji, C. Li, Z. Cong, Y. Zhang, J. Guo, W. Du and J. Huang, Linking atmospheric pollution to cryospheric change in the Third Pole region: current progress and future prospects, Natl. Sci. Rev., 2019, 6, 796–809 CrossRef CAS PubMed
.
- D. Koch and A. Del Genio, Black carbon semi-direct effects on cloud cover: review and synthesis, Atmos. Chem. Phys., 2010, 10, 7685–7696 CrossRef CAS
.
- K. Ding, X. Huang, A. Ding, M. Wang, H. Su, V. M. Kerminen, T. Petäjä, Z. Tan, Z. Wang, D. Zhou and J. Sun, Aerosol-boundary-layer-monsoon interactions amplify semi-direct effect of biomass smoke on low cloud formation in Southeast Asia, Nat. Commun., 2021, 12(1), 1–9 CrossRef PubMed
.
- U. Lohmann and J. Feichter, Global indirect aerosol effects: a review, Atmos. Chem. Phys., 2005, 5, 715–737 CrossRef CAS
.
- J. L. Jimenez, M. R. Canagaratna, N. M. Donahue, A. S. H. Prévôt, Q. Zhang, J. H. Kroll, P. F. DeCarlo, J. D. Allan, H. Coe, N. L. Ng, A. C. Aiken, K. S. Docherty, I. M. Ulbrich, A. P. Grieshop, A. L. Robinson, J. Duplissy, J. D. Smith, K. R. Wilson, V. A. Lanz, C. Hueglin, Y. L. Sun, J. Tian, A. Laaksonen, T. Raatikainen, J. Rautiainen, P. Vaattovaara, M. Ehn, M. Kulmala, J. M. Tomlinson, D. R. Collins, M. J. Cubison, J. Dunlea, J. A. Huffman, T. B. Onasch, M. R. Alfarra, P. I. Williams, K. Bower, Y. Kondo, J. Schneider, F. Drewnick, S. Borrmann, S. Weimer, K. Demerjian, D. Salcedo, L. Cottrell, R. Griffin, A. Takami, T. Miyoshi, S. Hatakeyama, A. Shimono, J. Y. Sun, Y. M. Zhang, K. Dzepina, J. R. Kimmel, D. Sueper, J. T. Jayne, S. C. Herndon, A. M. Trimborn, L. R. Williams, E. C. Wood, A. M. Middlebrook, C. E. Kolb, U. Baltensperger and D. R. Worsnop, Evolution of Organic Aerosols in the Atmosphere, Science, 2009, 326, 1525–1529 CrossRef CAS PubMed
.
- T. Novakov, S. Menon, T. W. Kirchstetter, D. Koch and J. E. Hansen, Aerosol organic carbon to black carbon ratios: analysis of published data and implications for climate forcing, J. Geophys. Res.: Atmos., 2005, 110(D21) DOI:10.1029/2005jd005977
.
- Y. Feng, V. Ramanathan and V. Kotamarthi, Brown carbon: a significant atmospheric absorber of solar radiation?, Atmos. Chem. Phys., 2013, 13, 8607–8621 CrossRef
.
- A. Laskin, J. Laskin and S. A. Nizkorodov, Chemistry of atmospheric brown carbon, Chem. Rev., 2015, 115, 4335–4382 CrossRef CAS PubMed
.
- D. S. Jo, R. J. Park, S. Lee, S. W. Kim and X. Zhang, A global simulation of brown carbon: implications for photochemistry and direct radiative effect, Atmos. Chem. Phys., 2016, 16, 3413–3432 CrossRef CAS
.
- P. Chen, S. Kang, L. Tripathee, K. Ram, M. Rupakheti, A. K. Panday, Q. Zhang, J. Guo, X. Wang, T. Pu and C. Li, Light absorption properties of elemental carbon (EC) and water-soluble brown carbon (WS–BrC) in the Kathmandu Valley, Nepal: A 5-year study, Environ. Pollut., 2020, 261, 114239 CrossRef CAS PubMed
.
- S. K. Sharma, T. K. Mandal, M. Saxena, A. Sharma, A. Datta and T. Saud, Variation of OC, EC, WSIC and trace metals of PM10 in Delhi, India, J. Atmos. Sol.-Terr. Phys., 2014, 113, 10–22 CrossRef CAS
.
- N. Rastogi, R. Agnihotri, R. Sawlani, A. Patel, S. S. Babu and R. Satish, Chemical and isotopic characteristics of PM10 over the Bay of Bengal: Effects of continental outflow on a marine environment, Sci. Total Environ., 2020, 726, 138438 CrossRef CAS PubMed
.
- W. Cooke, C. Liousse, H. Cachier and J. Feichter, Construction of a 1 × 1 fossil fuel emission data set for carbonaceous aerosol and implementation and radiative impact in the ECHAM4 model, J. Geophys. Res.: Atmos., 1999, 104, 22137–22162 CrossRef CAS
.
- T. C. Bond, D. G. Streets, K. F. Yarber, S. M. Nelson, J. H. Woo and Z. Klimont, A technology-based global inventory of black and organic carbon emissions from combustion, J. Geophys. Res.: Atmos., 2004, 109(D14) DOI:10.1029/2003JD003697
.
- G. Wu, K. Ram, P. Fu, W. Wang, Y. Zhang, X. Liu, E. A. Stone, B. B. Pradhan, P. M. Dangol, A. K. Panday, X. Wan, Z. Bai, S. Kang, Q. Zhang and Z. Cong, Water-Soluble Brown Carbon in Atmospheric Aerosols from Godavari (Nepal), a Regional Representative of South Asia, Environ. Sci. Technol., 2019, 53, 3471–3479 CrossRef CAS PubMed
.
- J. Wang, A. Yu, L. Yang and C. Fang, Research on Organic Carbon and Elemental Carbon Distribution Characteristics and Their Influence on Fine Particulate Matter (PM2.5) in Changchun City, Environments, 2019, 6, 21 CrossRef
.
- J. Lelieveld, P. J. Crutzen, V. Ramanathan, M. O. Andreae, C. A. M. Brenninkmeijer, T. Campos, G. R. Cass, R. R. Dickerson, H. Fischer, J. A. de Gouw, A. Hansel, A. Jefferson, D. Kley, A. T. J. de Laat, S. Lal, M. G. Lawrence, J. M. Lobert, O. L. Mayol-Bracero, A. P. Mitra, T. Novakov, S. J. Oltmans, K. A. Prather, T. Reiner, H. Rodhe, H. A. Scheeren, D. Sikka and J. Williams, The Indian Ocean Experiment: Widespread Air Pollution from South and Southeast Asia, Science, 2001, 291, 1031–1036 CrossRef CAS PubMed
.
- V. Ramanathan, P. Crutzen, J. Kiehl and D. Rosenfeld, Aerosols, climate, and the hydrological cycle, Science, 2001, 294, 2119–2124 CrossRef CAS PubMed
.
- A. Kumar, A. Sudheer and M. Sarin, Chemical characteristics of aerosols in MABL of Bay of Bengal and Arabian Sea during spring inter-monsoon: a comparative study, J. Earth Syst. Sci., 2008, 117, 325–332 CrossRef CAS
.
- A. Kumar, M. M. Sarin and B. Srinivas, Aerosol iron solubility over Bay of Bengal: Role of anthropogenic sources and chemical processing, Mar. Chem., 2010, 121, 167–175 CrossRef CAS
.
- N. Ojha, A. Sharma, M. Kumar, I. Girach, T. U. Ansari, S. K. Sharma, N. Singh, A. Pozzer and S. S. Gunthe, On the widespread enhancement in fine particulate matter across the Indo-Gangetic Plain towards winter, Sci. Rep., 2020, 10, 5862 CrossRef CAS PubMed
.
- A. K. Sudheer and M. M. Sarin, Carbonaceous aerosols in MABL of Bay of Bengal: Influence of continental outflow, Atmos. Environ., 2008, 42, 4089–4100 CrossRef CAS
.
- B. Srinivas and M. M. Sarin, Atmospheric pathways of phosphorous to the Bay of Bengal: contribution from anthropogenic sources and mineral dust, Tellus B, 2012, 64(1), 17174 CrossRef
.
- B. Srinivas and M. M. Sarin, Light absorbing organic aerosols (brown carbon) over the tropical Indian Ocean: impact of biomass burning emissions, Environ. Res. Lett., 2013, 8, 044042 CrossRef
.
- S. Dasari, A. Andersson, S. Bikkina, H. Holmstrand, K. Budhavant, S. Satheesh, E. Asmi, J. Kesti, J. Backman, A. Salam, D. S. Bisht, S. Tiwari, Z. Hameed and Ö. Gustafsson, Photochemical degradation affects the light absorption of water-soluble brown carbon in the South Asian outflow, Sci. Adv., 2019, 5, eaau8066, DOI:10.1126/sciadv.aau8066
.
- M. M. Grand, C. I. Measures, M. Hatta, W. T. Hiscock, C. S. Buck and W. M. Landing, Dust deposition in the eastern Indian Ocean: The ocean perspective from Antarctica to the Bay of Bengal, Global Biogeochem. Cycles, 2015, 29, 357–374 CrossRef CAS
.
- S. K. Satheesh, V. Ramanathan, B. N. Holben, K. K. Moorthy, N. G. Loeb, H. Maring, J. M. Prospero and D. Savoie, Chemical, microphysical, and radiative effects of Indian Ocean aerosols, J. Geophys. Res.: Atmos., 2002, 107(D23), AAC-20 CrossRef
.
- S. Tiwari, A. K. Mishra and A. K. Singh, Aerosol Climatology over the Bay of Bengal and Arabian Sea Inferred from Space-Borne Radiometers and Lidar Observations, Aerosol Air Qual. Res., 2016, 16, 2855–2868 CrossRef CAS
.
- K. Niranjan, V. Sreekanth, B. L. Madhavan and K. Krishna Moorthy, Wintertime aerosol characteristics at a north Indian site Kharagpur in the Indo-Gangetic plains located at the outflow region into Bay of Bengal, J. Geophys. Res.: Atmos., 2006, 111(D24) DOI:10.1029/2006jd007635
.
- I. A. Girach, N. Tripathi, P. R. Nair, L. K. Sahu and N. Ojha, O3 and CO in the South Asian outflow over the Bay of Bengal: Impact of monsoonal dynamics and chemistry, Atmos. Environ., 2020, 233, 117610 CrossRef CAS
.
- T. N. Krishnamurti, B. Jha, P. J. Rasch and V. Ramanathan, A high resolution global reanalysis highlighting the winter monsoon. Part I, reanalysis fields, Meteorol. Atmos. Phys., 1997, 64, 123–150 CrossRef
.
- R. Rengarajan, A. K. Sudheer and M. M. Sarin, Wintertime PM2.5 and PM10 carbonaceous and inorganic constituents from urban site in western India, Atmos. Res., 2011, 102, 420–431 CrossRef CAS
.
- S. Tiwari, D. Kaskaoutis, V. K. Soni, S. Dev Attri and A. K. Singh, Aerosol columnar characteristics and their heterogeneous nature over Varanasi, in the central Ganges valley, Environ. Sci. Pollut. Res., 2018, 25, 24726–24745 CrossRef CAS PubMed
.
- S. Jain, S. Sharma, N. Vijayan and T. Mandal, Seasonal characteristics of aerosols (PM2.5 and PM10) and their source apportionment using PMF: a four year study over Delhi, India, Environ. Pollut., 2020, 262, 114337 CrossRef CAS PubMed
.
- A. Kumar, K. Ram and N. Ojha, Variations in carbonaceous species at a high-altitude site in western India: Role of synoptic scale transport, Atmos. Environ., 2016, 125, 371–382 CrossRef CAS
.
- K. Ram, M. Sarin and P. Hegde, Atmospheric abundances of primary and secondary carbonaceous species at two high-altitude sites in India: sources and temporal variability, Atmos. Environ., 2008, 42, 6785–6796 CrossRef CAS
.
- A. Kumar, S. Singh, N. Kumar, N. Singh, K. Kumar and S. Chourasiya, Assessment of carbonaceous fractions in ambient aerosols at high altitude and lowland urban locations of Kumaon Province, Indian Himalaya, SN Appl. Sci., 2021, 3, 1–14 Search PubMed
.
- O. Mayol-Bracero, P. Guyon, B. Graham, G. Roberts, M. Andreae, S. Decesari, M. Facchini, S. Fuzzi and P. Artaxo, Water-soluble organic compounds in biomass burning aerosols over Amazonia 2. Apportionment of the chemical composition and importance of the polyacidic fraction, J. Geophys. Res., 2002, 107, 8091 CrossRef
.
- C. Neusüβ, T. Gnauk, A. Plewka, H. Herrmann and P. K. Quinn, Carbonaceous aerosol over the Indian Ocean: OC/EC fractions and selected specifications from size-segregated onboard samples, J. Geophys. Res.: Atmos., 2002, 107(D19), INX2-30 Search PubMed
.
- A. Kumar, A. K. Sudheer, V. Goswami and R. Bhushan, Influence of continental outflow on aerosol chemical characteristics over the Arabian Sea during winter, Atmos. Environ., 2012, 50, 182–191 CrossRef CAS
.
- S. K. Satheesh, J. Srinivasan and K. K. Moorthy, Spatial and temporal heterogeneity in aerosol properties and radiative forcing over Bay of Bengal: Sources and role of aerosol transport, J. Geophys. Res.: Atmos., 2006, 111(D8) DOI:10.1029/2005jd006374
.
- V. Vinoj, S. S. Babu, S. K. Satheesh, K. Moorthy and Y. J. Kaufman, Radiative forcing by aerosols over the Bay of Bengal region derived from shipborne, island-based, and satellite (Moderate-Resolution Imaging Spectroradiometer) observations, J. Geophys. Res.: Atmos., 2004, 109(D5) DOI:10.1029/2003jd004329
.
- D. Ganguly, A. Jayaraman and H. Gadhavi,
In situ ship cruise measurements of mass concentration and size distribution of aerosols over Bay of Bengal and their radiative impacts, J. Geophys. Res.: Atmos., 2005, 110 Search PubMed
.
- K. K. Moorthy and S. S. Babu, Aerosol black carbon over Bay of Bengal observed from an island location, Port Blair: Temporal features and long-range transport, J. Geophys. Res.: Atmos., 2006, 111(D17) DOI:10.1029/2005jd006855
.
- H.-H. Lee and C. Wang, The impacts of biomass burning activities on convective systems over the Maritime Continent, Atmos. Chem. Phys., 2020, 20, 2533–2548 CrossRef CAS
.
- S. Naseema Beegum, K. Krishna Moorthy, M. M. Gogoi, S. Suresh Babu and S. Pandey, Multi-year investigations of aerosols from an island station, Port Blair, in the Bay of Bengal: climatology and source impacts, Ann. Geophys., 2012, 30(8), 1113–1127 CrossRef
.
- A. Stein, R. Draxler, G. Rolph, B. Stunder, M. Cohen and F. Ngan, NOAA's HYSPLIT atmospheric transport and dispersion modeling system, Bull. Am. Meteorol. Soc., 2015, 96, 2059–2077 CrossRef
.
- G. Rolph, A. Stein and B. Stunder, Real-time environmental applications and display system: READY, Environ. Model. Software, 2017, 95, 210–228 CrossRef
.
- M. E. Birch and R. A. Cary, Elemental Carbon-Based Method for Monitoring Occupational Exposures to Particulate Diesel Exhaust, Aerosol Sci. Technol., 1996, 25, 221–241 CrossRef CAS
.
- W. Xu, F. Wang, J. Li, L. Tian, X. Jiang, J. Yang and B. Chen, Historical variation in black carbon deposition and sources to Northern China sediments, Chemosphere, 2017, 172, 242–248 CrossRef CAS PubMed
.
- J. G. Watson, J. C. Chow and L.-W. A. Chen, Summary of organic and elemental carbon/black carbon analysis methods and intercomparisons, Aerosol Air Qual. Res., 2005, 5, 65–102 CrossRef CAS
.
- M. Catrambone, S. Canepari, M. Cerasa, T. Sargolini and C. Perrino, Performance evaluation of a very-low-volume sampler for atmospheric particulate matter, Aerosol Air Qual. Res., 2019, 19, 2160–2172 CrossRef CAS
.
- J. G. Watson, J. C. Chow, L.-W. A. Chen and N. H. Frank, Methods to assess carbonaceous aerosol sampling artifacts for IMPROVE and other long-term networks, J. Air Waste Manage. Assoc., 2009, 59, 898–911 CrossRef CAS PubMed
.
-
A. G. Dickson, C. L. Sabine and J. R. Christian, Guide to best practices for ocean CO2 measurements, North Pacific Marine Science Organization, 2007 Search PubMed
.
- A. Hecobian, X. Zhang, M. Zheng, N. Frank, E. S. Edgerton and R. J. Weber, Water-Soluble Organic Aerosol material and the light-absorption characteristics of aqueous extracts measured over the Southeastern United States, Atmos. Chem. Phys., 2010, 10, 5965–5977 CrossRef CAS
.
- B. J. Turpin and J. J. Huntzicker, Identification of secondary organic aerosol episodes and quantitation of primary and secondary organic aerosol concentrations during SCAQS, Atmos. Environ., 1995, 29, 3527–3544 CrossRef CAS
.
- Q. Zhang, S. Sarkar, X. Wang, J. Zhang, J. Mao, L. Yang, Y. Shi and S. Jia, Evaluation of factors influencing secondary organic carbon (SOC) estimation by CO and EC tracer methods, Sci. Total Environ., 2019, 686, 915–930 CrossRef CAS PubMed
.
- E. Merico, D. Cesari, A. Dinoi, A. Gambaro, E. Barbaro, M. R. Guascito, L. C. Giannossa, A. Mangone and D. Contini, Inter-comparison of carbon content in PM10 and PM2.5 measured with two thermo-optical protocols on samples collected in a Mediterranean site, Environ. Sci. Pollut. Res., 2019, 26, 29334–29350 CrossRef CAS PubMed
.
- L. Castro, C. Pio, R. M. Harrison and D. Smith, Carbonaceous aerosol in urban and rural European atmospheres: estimation of secondary organic carbon concentrations, Atmos. Environ., 1999, 33, 2771–2781 CrossRef CAS
.
- J. Liu, M. Bergin, H. Guo, L. King, N. Kotra, E. Edgerton and R. Weber, Size-resolved measurements of brown carbon in water and methanol extracts and estimates of their contribution to ambient fine-particle light absorption, Atmos. Chem. Phys., 2013, 13, 12389–12404 CrossRef
.
- H. Sun, L. Biedermann and T. C. Bond, Color of brown carbon: A model for ultraviolet and visible light absorption by organic carbon aerosol, Geophys. Res. Lett., 2007, 34(17) DOI:10.1029/2007gl029797
.
- C. Bosch, A. Andersson, E. N. Kirillova, K. Budhavant, S. Tiwari, P. S. Praveen, L. M. Russell, N. D. Beres, V. Ramanathan and Ö. Gustafsson, Source-diagnostic dual-isotope composition and optical properties of water-soluble organic carbon and elemental carbon in the South Asian outflow intercepted over the Indian Ocean, J. Geophys. Res.: Atmos., 2014, 119(20), 11743–11759 CAS
.
- K. Ram and M. Sarin, Absorption coefficient and site-specific mass absorption efficiency of elemental carbon in aerosols over urban, rural, and high-altitude sites in India, Environ. Sci. Technol., 2009, 43, 8233–8239 CrossRef CAS PubMed
.
- E. Weingartner, H. Saathoff, M. Schnaiter, N. Streit, B. Bitnar and U. Baltensperger, Absorption of light by soot particles: determination of the absorption coefficient by means of aethalometers, J. Aerosol Sci., 2003, 34, 1445–1463 CrossRef CAS
.
- S. Bikkina and M. Sarin, Brown Carbon in the continental outflow to the North Indian Ocean, Environ. Sci.: Processes Impacts, 2019, 21(6), 970–987 RSC
.
- P. Bikkina, S. Bikkina, K. Kawamura, A. K. Sudheer, G. Mahesh and S. K. Kumar, Evidence for brown carbon absorption over the Bay of Bengal during the southwest monsoon season: a possible oceanic source, Environ. Sci.: Processes Impacts, 2020, 22(8), 1743–1758 RSC
.
- E. N. Kirillova, A. Andersson, S. Tiwari, A. K. Srivastava, D. S. Bisht and Ö. Gustafsson, Water-soluble organic carbon aerosols during a full New Delhi winter: Isotope-based source apportionment and optical properties, J. Geophys. Res.: Atmos., 2014, 119, 3476–3485 CAS
.
- R. Levinson, H. Akbari and P. Berdahl, Measuring solar reflectance—Part I: Defining a metric that accurately predicts solar heat gain, Sol. Energy, 2010, 84, 1717–1744 CrossRef
.
- B. Srinivas, A. Kumar, M. Sarin and A. Sudheer, Impact of continental outflow on chemistry of atmospheric aerosols over tropical Bay of Bengal, Atmos. Chem. Phys., 2011, 11, 20667–20711 Search PubMed
.
- B. J. Turpin and H.-J. Lim, Species contributions to PM2.5 mass concentrations: Revisiting common assumptions for estimating organic mass, Aerosol Sci. Technol., 2001, 35, 602–610 CrossRef CAS
.
- P. K. Quinn, D. J. Coffman, T. S. Bates, E. J. Welton, D. S. Covert, T. L. Miller, J. E. Johnson, S. Maria, L. Russell, R. Arimoto and C. M. Carrico, Aerosol optical properties measured on board the Ronald H. Brown during ACE-Asia as a function of aerosol chemical composition and source region, J. Geophys. Res.: Atmos., 2004, 109(D19) DOI:10.1029/2003jd004010
.
- C. L. Heald, D. K. Henze, L. W. Horowitz, J. Feddema, J. F. Lamarque, A. Guenther, P. G. Hess, F. Vitt, J. H. Seinfeld, A. H. Goldstein and I. Fung, Predicted change in global secondary organic aerosol concentrations in response to future climate, emissions, and land use change, J. Geophys. Res.: Atmos., 2008, 113(D5) DOI:10.1029/2007jd009092
.
- J. Sciare, K. Oikonomou, O. Favez, E. Liakakou, Z. Markaki, H. Cachier and N. Mihalopoulos, Long-term measurements of carbonaceous aerosols in the Eastern Mediterranean: evidence of long-range transport of biomass burning, Atmos. Chem. Phys., 2008, 8, 5551–5563 CrossRef CAS
.
- S. Zhou, Z. Wang, R. Gao, L. Xue, C. Yuan, T. Wang, X. Gao, X. Wang, W. Nie and Z. Xu, Formation of secondary organic carbon and long-range transport of carbonaceous aerosols at Mount Heng in South China, Atmos. Environ., 2012, 63, 203–212 CrossRef CAS
.
- H.-J. Lim, B. Turpin, L. Russell and T. Bates, Organic and elemental carbon measurements during ACE-Asia suggest a longer atmospheric lifetime for elemental carbon, Environ. Sci. Technol., 2003, 37, 3055–3061 CrossRef CAS PubMed
.
- K. Fomba, K. Müller, D. Van Pinxteren, L. Poulain, M. Van Pinxteren and H. Herrmann, Long-term chemical characterization of tropical and marine aerosols at the Cape Verde Atmospheric Observatory (CVAO) from 2007 to 2011, Atmos. Chem. Phys., 2014, 14, 8883–8904 CrossRef CAS
.
- L. K. Sahu, Y. Kondo, Y. Miyazaki, M. Kuwata, M. Koike, N. Takegawa, H. Tanimoto, H. Matsueda, S. C. Yoon and Y. J. Kim, Anthropogenic aerosols observed in Asian continental outflow at Jeju Island, Korea, in spring 2005, J. Geophys. Res.: Atmos., 2009, 114(D3) DOI:10.1029/2008jd010306
.
- Y. P. Kim, J. H. Lee, N. J. Baik, J. Y. Kim, S.-G. Shim and C.-H. Kang, Summertime characteristics of aerosol composition at Cheju Island, Korea, Atmos. Environ., 1998, 32, 3905–3915 CrossRef CAS
.
- S. Bikkina, A. Andersson, M. M. Sarin, R. J. Sheesley, E. Kirillova, R. Rengarajan, A. K. Sudheer, K. Ram and Ö. Gustafsson, Dual carbon isotope characterization of total organic carbon in wintertime carbonaceous aerosols from northern India, J. Geophys. Res.: Atmos., 2016, 121, 4797–4809 CAS
.
- P. Chen, S. Kang, C. Li, Q. Zhang, J. Guo, L. Tripathee, Y. Zhang, G. Li, C. Gul and Z. Cong, Carbonaceous aerosol characteristics on the Third Pole: A primary study based on the Atmospheric Pollution and Cryospheric Change (APCC) network, Environ. Pollut., 2019, 253, 49–60 CrossRef CAS PubMed
.
- M. O. Andreae, Soot carbon and excess fine potassium: Long-range transport of combustion-derived aerosols, Science, 1983, 220, 1148–1151 CrossRef CAS PubMed
.
- P. Saxena and L. M. Hildemann, Water-soluble organics in atmospheric particles: A critical review of the literature and application of thermodynamics to identify candidate compounds, J. Atmos. Chem., 1996, 24, 57–109 CrossRef CAS
.
- B. Srinivas and M. M. Sarin, PM2.5, EC and OC in atmospheric outflow from the Indo-Gangetic Plain: Temporal variability and aerosol organic carbon-to-organic mass conversion factor, Sci. Total Environ., 2014, 487, 196–205 CrossRef CAS PubMed
.
- N. Rastogi, A. Singh, M. Sarin and D. Singh, Temporal variability of primary and secondary aerosols over northern India: Impact of biomass burning emissions, Atmos. Environ., 2016, 125, 396–403 CrossRef CAS
.
- T. Novakov, S. Menon, T. Kirchstetter, D. Koch and J. Hansen, Aerosol organic carbon to black carbon ratios: Analysis of published data and implications for climate forcing, J. Geophys. Res.: Atmos., 2005, 110 Search PubMed
.
- J. C. Chow, J. G. Watson, L. C. Pritchett, W. R. Pierson, C. A. Frazier and R. G. Purcell, The DRI thermal/optical reflectance carbon analysis system: description, evaluation and applications in US air quality studies, Atmos. Environ., Part A, 1993, 27, 1185–1201 CrossRef
.
- A. Sudheer and M. Sarin, Carbonaceous aerosols in MABL of Bay of Bengal: influence of continental outflow, Atmos. Environ., 2008, 42, 4089–4100 CrossRef CAS
.
- M. O. Andreae and P. Merlet, Emission of trace gases and aerosols from biomass burning, Global Biogeochem. Cycles, 2001, 15, 955–966 CrossRef CAS
.
- P. Rajput, M. Sarin, D. Sharma and D. Singh, Characteristics and emission budget of carbonaceous species from post-harvest agricultural-waste burning in source region of the Indo-Gangetic Plain, Tellus B, 2014, 66(1), 21026 CrossRef
.
- S. A. Guazzotti, D. T. Suess, K. R. Coffee, P. K. Quinn, T. S. Bates, A. Wisthaler, A. Hansel, W. P. Ball, R. R. Dickerson, C. Neusüβ, P. J. Crutzen and K. A. Prather, Characterization of carbonaceous aerosols outflow from India and Arabia: Biomass/biofuel burning and fossil fuel combustion, J. Geophys. Res.: Atmos., 2003, 108(D15) DOI:10.1029/2002jd003277
.
- R. Paris, K. V. Desboeufs, P. Formenti, S. Nava and C. Chou, Chemical characterisation of iron in dust and biomass burning aerosols during AMMA-SOP0/DABEX: implication for iron solubility, Atmos. Chem. Phys., 2010, 10, 4273–4282 CrossRef CAS
.
- S. K. Pani, S. Chantara, C. Khamkaew, C.-T. Lee and N.-H. Lin, Biomass burning in the northern peninsular Southeast Asia: aerosol chemical profile and potential exposure, Atmos. Res., 2019, 224, 180–195 CrossRef CAS
.
- L. Liang, G. Engling, Y. Cheng, X. Liu, Z. Du, Q. Ma, X. Zhang, J. Sun, W. Xu and C. Liu, Biomass burning impacts on ambient aerosol at a background site in East China: Insights from a yearlong study, Atmos. Res., 2020, 231, 104660 CrossRef CAS
.
- T. Novakov, M. Andreae, R. Gabriel, T. Kirchstetter, O. Mayol-Bracero and V. Ramanathan, Origin of carbonaceous aerosols over the tropical Indian Ocean: Biomass burning or fossil fuels, Geophys. Res. Lett., 2000, 27, 4061–4064 CrossRef CAS
.
- O. L. Mayol-Bracero, R. Gabriel, M. O. Andreae, T. W. Kirchstetter, T. Novakov, J. Ogren, P. Sheridan and D. G. Streets, Carbonaceous aerosols over the Indian Ocean during the Indian Ocean Experiment (INDOEX): Chemical characterization, optical properties, and probable sources, J. Geophys. Res.: Atmos., 2002, 107(D19), INX2-29 CrossRef
.
- U. Pöschl, Atmospheric aerosols: composition, transformation, climate and health effects, Angew. Chem., Int. Ed., 2005, 44, 7520–7540 CrossRef PubMed
.
- S. Liu, A. C. Aiken, K. Gorkowski, M. K. Dubey, C. D. Cappa, L. R. Williams, S. C. Herndon, P. Massoli, E. C. Fortner, P. S. Chhabra, W. A. Brooks, T. B. Onasch, J. T. Jayne, D. R. Worsnop, S. China, N. Sharma, C. Mazzoleni, L. Xu, N. L. Ng, D. Liu, J. D. Allan, J. D. Lee, Z. L. Fleming, C. Mohr, P. Zotter, S. Szidat and A. S. H. Prévôt, Enhanced light absorption by mixed source black and brown carbon particles in UK winter, Nat. Commun., 2015, 6, 8435 CrossRef CAS PubMed
.
- P. M. Shamjad, S. N. Tripathi, N. M. Thamban and H. Vreeland, Refractive Index and Absorption Attribution of Highly Absorbing Brown Carbon Aerosols from an Urban Indian City-Kanpur, Sci. Rep., 2016, 6, 37735 CrossRef CAS PubMed
.
- R. Satish, P. Shamjad, N. Thamban, S. Tripathi and N. Rastogi, Temporal Characteristics of Brown Carbon over the Central Indo-Gangetic Plain, Environ. Sci. Technol., 2017, 51, 6765–6772 CrossRef CAS PubMed
.
- S. Bikkina and M. Sarin, Brown carbon in the continental outflow to the North Indian Ocean, Environ. Sci.: Processes Impacts, 2019, 21, 970–987 RSC
.
- Y. Zhang, H. Forrister, J. Liu, J. Dibb, B. Anderson, J. P. Schwarz, A. E. Perring, J. L. Jimenez, P. Campuzano-Jost, Y. Wang, A. Nenes and R. J. Weber, Top-of-atmosphere radiative forcing affected by brown carbon in the upper troposphere, Nat. Geosci., 2017, 10, 486–489 CrossRef CAS
.
- S. Yue, S. Bikkina, M. Gao, L. A. Barrie, K. Kawamura and P. Fu, Sources and Radiative Absorption of Water-Soluble Brown Carbon in the High Arctic Atmosphere, Geophys. Res. Lett., 2019, 46, 14881–14891 CrossRef
.
- L. Zeng, A. Zhang, Y. Wang, N. L. Wagner, J. M. Katich, J. P. Schwarz, G. P. Schill, C. Brock, K. D. Froyd, D. M. Murphy, C. J. Williamson, A. Kupc, E. Scheuer, J. Dibb and R. J. Weber, Global Measurements of Brown Carbon and Estimated Direct Radiative Effects, Geophys. Res. Lett., 2020, 47, e2020GL088747 CAS
.
-
R. J. Weber, L. Zeng, A. Zhang, Y. Wang, N. L. Wagner, J. M. Katich, J. P. Schwarz, G. P. Schill, C. A. Brock and K. Froyd, Global Measurements of Brown Carbon and Estimated Direct Radiative Effects, 2020 Search PubMed
.
- T. W. Kirchstetter, T. Novakov and P. V. Hobbs, Evidence that the spectral dependence of light absorption by aerosols is affected by organic carbon, J. Geophys. Res.: Atmos., 2004, 109(D21) DOI:10.1029/2004jd004999
.
- E. Liakakou, D. Kaskaoutis, G. Grivas, I. Stavroulas, M. Tsagkaraki, D. Paraskevopoulou, A. Bougiatioti, U. Dumka, E. Gerasopoulos and N. Mihalopoulos, Long-term brown carbon spectral characteristics in a Mediterranean city (Athens), Sci. Total Environ., 2020, 708, 135019 CrossRef CAS PubMed
.
- Y. Cheng, K.-B. He, M. Zheng, F.-K. Duan, Z.-Y. Du, Y.-L. Ma, J.-H. Tan, F.-M. Yang, J.-M. Liu and X.-L. Zhang, Mass absorption efficiency of elemental carbon and water-soluble organic carbon in Beijing, China, Atmos. Chem. Phys., 2011, 11, 11497–11510 CrossRef CAS
.
- B. Srinivas, N. Rastogi, M. Sarin, A. Singh and D. Singh, Mass absorption efficiency of light absorbing organic aerosols from source region of paddy-residue burning emissions in the Indo-Gangetic Plain, Atmos. Environ., 2016, 125, 360–370 CrossRef CAS
.
- B. Srinivas and M. Sarin, Brown carbon in atmospheric outflow from the Indo-Gangetic Plain: Mass absorption efficiency and temporal variability, Atmos. Environ., 2014, 89, 835–843 CrossRef CAS
.
- Z. Du, K. He, Y. Cheng, F. Duan, Y. Ma, J. Liu, X. Zhang, M. Zheng and R. Weber, A yearlong study of water-soluble organic carbon in Beijing II: Light absorption properties, Atmos. Environ., 2014, 89, 235–241 CrossRef CAS
.
- C. Yan, M. Zheng, A. P. Sullivan, C. Bosch, Y. Desyaterik, A. Andersson, X. Li, X. Guo, T. Zhou and Ö. Gustafsson, Chemical characteristics and light-absorbing property of water-soluble organic carbon in Beijing: Biomass burning contributions, Atmos. Environ., 2015, 121, 4–12 CrossRef CAS
.
- S. Bikkina and M. M. Sarin, Light absorbing organic aerosols (brown carbon) over the tropical Indian Ocean: impact of biomass burning emissions, Environ. Res. Lett., 2013, 8, 044042 CrossRef CAS
.
- V. Choudhary, P. Rajput, P. Rajeev and T. Gupta, Synergistic effect in absorption properties of brown carbon and elemental carbon over IGP during weak south-west monsoon, Aerosol Sci. Eng., 2017, 1, 138–149 CrossRef
.
- E. N. Kirillova, A. Andersson, J. Han, M. Lee and Ö. Gustafsson, Sources and light absorption of water-soluble organic carbon aerosols in the outflow from northern China, Atmos. Chem. Phys., 2014, 14, 1413–1422 CrossRef
.
Footnote |
† Electronic supplementary information (ESI) available. See DOI: 10.1039/d1em00347j |
|
This journal is © The Royal Society of Chemistry 2022 |
Click here to see how this site uses Cookies. View our privacy policy here.