DOI:
10.1039/D2FO02197H
(Paper)
Food Funct., 2022,
13, 12105-12120
Valorisation of liquorice (Glycyrrhiza) roots: antimicrobial activity and cytotoxicity of prenylated (iso)flavonoids and chalcones from liquorice spent (G. glabra, G. inflata, and G. uralensis)†
Received
27th July 2022
, Accepted 31st October 2022
First published on 15th November 2022
Abstract
Prenylated phenolics are antimicrobials found in liquorice (Glycyrrhiza spp.). Liquorice spent is a by-product rich in prenylated phenolics obtained after water extraction of roots, and is currently not valorised. We analysed the prenylated phenolics composition of spent extracts from Glycyrrhiza glabra, G. inflata, and G. uralensis, their antimicrobial activity, cytotoxicity, and effects on Caco-2 cell viability. G. glabra, G. inflata, and G. uralensis spent extracts showed distinct phytochemical profiles. Antibacterial activity (Lactobacillus buchneri, Streptococcus mutans, and Staphylococcus aureus) of G. uralensis and G. inflata (MICs 25–250 μg mL−1) was higher than of G. glabra (MICs 75–1000 μg mL−1). Marker compounds glabridin, licochalcone A, and glycycoumarin were equally potent (MICs 12.5–25 μg mL−1). G. inflata and G. uralensis showed cytotoxicity at 500 μg mL−1, whereas G. glabra was not toxic up to 1000 μg mL−1, but showed reduced viability between 50–500 μg mL−1. Linking antibacterial activity of the liquorice spent extracts with cell viability showed that MICs against S. aureus coincide with concentrations where cell viability was not reduced, whereas for the other bacteria and yeasts MICs concurred at concentrations where cell viability was reduced. In this study we show that liquorice spent is a by-product rich in antibacterial prenylated phenolics that offers interesting oppurtunities for e.g. control of microorganisms and the discovery of novel plant-derived antimicrobials.
1. Introduction
The Glycyrrhiza genus comprises different species, with G. glabra, G. inflata, and G. uralensis being the best known commercially used. Water extracts of liquorice roots are i.a. used as a flavouring agent, but have also a longstanding use in traditional herbal medicine, used to treat i.a. cough, inflammation, and liver damage.1,2 As of 2012, the European Medicines Agency (EMA) has recommended the use of liquorice roots for their pharmacological properties.3 The world's demand for liquorice extract is increasing, with a global liquorice extract market value of $1.9 billion in 2019, which is anticipated to increase towards $2.9 billion by 2029.4,5 Glycyrrhizin (a triterpenoid saponin) is the main compound responsible for the liquorice flavour and sweetness.6 Besides glycyrrhizin, liquorice roots are rich in prenylated (iso)flavonoids and chalcones (collectively referred to as prenylated phenolics, for included subclasses see Fig. A1, ESI†). These compounds have shown to possess potent antimicrobial activity.7,8 Natural antimicrobials are in high demand as alternatives for existing antimicrobials that face increased resistance and often become part of the human food chain.9 In general, there is an increasing consumer interest for minimal processed and “natural” products.10 Specialised (‘secondary’) metabolites from plants, either as pure compounds or as extracts, may provide novel antimicrobials.11 Under (a)biotic stress, prenylated phenolics are produced by plants from the Fabaceae (Leguminosae) family, including liquorice roots (Glycyrrhiza spp.).12 Recently, we showed that an ethyl acetate (EtOAc) extract of G. glabra roots is rich in prenylated phenolics, with prenylated isoflavans being the most abundant.13 Additionally, it was shown that methanolic root extracts of G. glabra, G. inflata, and G. uralensis each have a distinct prenylated phenolic profile: G. glabra is rich in prenylated isoflavans, G. inflata in prenylated chalcones, and G. uralensis in prenylated isoflavans.14–17 Furthermore, these studies revealed considerable interspecies chemical variation, by discovering 27 species-specific compounds, the most abundant species-specific compounds were glabridin (glab), licochalcone A (licoA), and glycycoumarin (glycy) for G. glabra, G. inflata, and G. uralensis, respectively (Fig. 1).14 Previous research has established antibacterial activity of the aforementioned compounds, with minimum inhibitory concentrations (MICs) of 49 μM (16 μg mL−1) for glab, 77 μM (26 μg mL−1) for licoA, and 44 μM (16 μg mL−1) for glycy against methicillin-resistant Staphylococcus aureus (MRSA).18 Natural compounds with a MIC ≤25 μg mL−1 are considered potent antibacterials.19
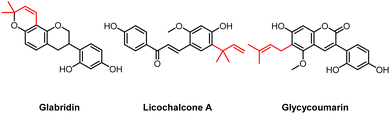 |
| Fig. 1 Molecular structures of the main species-specific compounds glabridin (glab) (ring prenylated isoflavan from G. glabra), licochalcone A (licoA) (chain prenylated chalcone from G. inflata), and glycycoumarin (glycy) (chain prenylated 3-arylcoumairn from G. uralensis). The prenyl group is indicated in red. | |
In general, prenylation of phenolics increases their antimicrobial activity, as was demonstrated in a previous study from our group, reporting MICs of 29 μM (10 μg mL−1) for 6-prenylgenistein (single prenylated) and 15 μM (6.3 μg mL−1) for 6,8-diprenylgenistein (double prenylated), against the food pathogen Listeria monocytogenes EGD-e.20 Reported MIC values of the non-prenylated counterpart (genistein) range between 237–3704 μM (64–1000 μg mL−1) against a variety of Gram-positive and Gram-negative bacteria.21,22
It has been proposed that prenylation leads to an increased antimicrobial activity by enhancing the hydrophobicity of the molecule, which leads to more interaction with- and/or disruption of bacterial membranes.23 As this might also hold for human cell membranes, concern regarding their safety for consumption is raised. Data from several studies show that prenylated phenolics induce cytotoxicity and reduce cell viability in different human cancer cell lines (with IC50 between 10–85 μM for prenylated chalcones and flavanones in HT-29 colon cells).24–27 However, possible cytotoxicity and potential effects on cell viability from complex liquorice spent extracts rich in prenylated phenolics has not been investigated before.
To date, liquorice roots are commercially used for e.g. glycyrrhizin extraction, leaving the spent as potential, thus far untapped source of bioactive compounds. With an average global annual liquorice water extract content of 58 kilotons (kt),28 it is expected that 232 kt spent is produced yearly (assuming a 25% (w/w) water extract yield), containing around 1.2 kt prenylated phenolics.13 Therefore, the by-product of liquorice roots after water extraction, also referred to as spent, was studied as a source of potent and non-toxic antimicrobial prenylated phenolics. To the best of our knowledge, the use of liquorice spent as source of antimicrobial prenylated phenolics has not been investigated before. As compounds glab, licoA, and glycy have shown potent antibacterial activity, valorisation of liquorice spent seems to offer interesting opportunities for e.g. control of microorganisms and the discovery of novel plant-derived antimicrobials. Therefore, the aim of this study is to get a comprehensive overview of the phytochemical composition, antimicrobial potential, and general toxicity of liquorice spent extracts and species-specific marker compounds glab, licoA, and glycy. For this, the phytochemical composition of G. glabra, G. inflata, and G. uralensis spent extracts was studied using reversed phase ultra-high pressure liquid chromatography (RP-UHPLC) coupled to photodiode array (PDA), ion trap mass spectrometry (IT-MSn) and Orbitrap-MS (FT-MSn). Antimicrobial activity of spent extracts and marker compounds was evaluated against food spoilers (preservation) Lactobacillus buchneri, Zygosaccharomyces parabailli, and Yarrowia lipolytica, oral pathogens (dental hygiene) Streptococcus mutans and Staphylococcus aureus, and the gastrointestinal (colonic health and fecal contamination) Escherichia coli. Last, potential effects on cytotoxicity (LDH) and cell viability (WST-1) of the spent extracts and marker compounds were evaluated in human colorectal adenocarcinoma cells (Caco-2 cells), a cell line that is widely used as in vitro screening model for intestinal barrier function and also in cytotoxicity studies.29
2. Materials and methods
2.1. Materials
Liquorice roots, Glycyrrhiza glabra (Iran), G. inflata (China), and G. uralensis (China) were provided by Ruitenberg BasIQs BV (Twello, The Netherlands). Glabridin (glab) (≥97.0%) was purchased from Wako (Osaka, Japan); licochalcone A (licoA) (≥96.0%), tert-butanol ≥98% (w/w), and Triton X-100 were from Sigma-Aldrich (St Louis, MO, USA); glycycoumarin (glycy) (≥98.0%) from Chemfaces (Wuhan, China; confirmed by RP-UHPLC-PDA-FT-MSn); ULC-MS grade ethyl acetate (EtOAc), methanol (MeOH), acetonitrile (ACN), ACN containing 0.1% (v/v) formic acid (FA), water containing 0.1% (v/v) FA were purchased from Biosolve (Valkenswaard, The Netherlands); dimethyl sulfoxide (DMSO) was purchased from Merck Millipore (Billerica, MA, USA). Water (MQ) for other purposes than UHPLC was prepared using a Milli-Q water purification system (Merck Millipore). Growth media, Bacto brain heart infusion (BHI) was purchased from BD (Franklin Lakes, NJ, USA); tryptone soya broth (TSB) and agar (TSA) bacteriological and DeMan-Rogosa-Sharpe (MRS) broth were obtained from Oxoid Ltd (Basingstoke, UK), yeast extract peptone dextrose broth (YPD) from Brunschwig Chemie (Amsterdam, The Netherlands), and peptone physiological salt solution (PPS) from Tritium Microbiologie (Eindhoven, The Netherlands). Phosphate buffered saline (PBS) and Dulbecco's modified Eagle's medium (DMEM) were purchased from Thermo Fisher Scientific Gibco (Waltham, Massachusetts, USA) and supplemented with glucose (4.5 g L−1), HEPES buffer solution (0.58 g L−1), L-glutamine (0.58 g L−1), 10% (v/v) heat inactivated fetal calf serum (FCS), and 1% (v/v) penicillin/streptomycin (P/S).
2.2. Microorganisms and mammalian cell line
Lactobacillus buchneri ATCC 4005, L. buchneri L4, Zygosaccharomyces parabailli ATCC 60483, and Z. parabailli UL 3699 were provided by Unilever (Unilever Foods Innovation Centre – Hive, Wageningen, The Netherlands); Streptococcus mutans (ATCC 27175) was purchased at DSMZ (Braunschweig, Germany); Staphylococcus aureus (ATCC 25923) and Escherichia coli (K12) were provided by the Laboratory of Food Microbiology (Wageningen University, Wageningen, The Netherlands); E. coli field isolates O54:H21 and O88:H8 were provided by Delacon Biotechnik GmbH (Engerwitzdorf, Austria); Yarrowia lipolytica food isolate was provided by Ruitenberg BasIQs BV (Twello, The Netherlands). Human colon adenocarcinoma cell line (Caco-2) was obtained from American Type Culture Collection (ATCC; Manassas, Virginia, US).
2.3. Sample preparation and extraction
Liquorice roots were freeze-dried prior to milling in a Retsch SM 2000 (Retsch, Haan, Germany), and subsequently sieved (pore size 250 μm, Retsch) to yield root powder. The resulting powder was extracted with MQ by ultrasound-assisted extraction (1
:
25 [w:w]) in 2 consecutive cycles of 60 min per cycle at 65 °C with extra shaking every 15 min, and the supernatants (after centrifugation for 10 min at 4696g at 4 °C) of both cycles were combined to yield the water extracts. Water extracts were freeze-dried (Christ Alpha 1-4 LD, Osterode am Harz, Germany) prior to compositional analysis by UHPLC-MS. Obtained pellet was referred to as spent and freeze-dried (Christ) prior to extraction with EtOAc. Subsequently, liquorice spent was extracted with EtOAc by ultrasound-assisted extraction (1
:
25 [w/w]) in 2 consecutive cycles of 60 min per cycle at 35 °C with extra shaking every 15 min. EtOAc extracts were centrifuged for 10 min at 4696g at room temperature (RT) and combined to yield spent extract. EtOAc in the spent extract was removed under reduced pressure and dried extract was resolubilized in tert-butanol and freeze-dried. Prior to UHPLC-MS analysis, the dried spent extract and standards were resolubilized in DMSO to a final concentration of 1 mg mL−1 and 100 μg mL−1 for ESI-IT-MSn analysis, or 50 μg mL−1 and 1 μg mL−1 for ESI-FT-MSn analysis, respectively; dried water extract was resolubilized in MQ to a final concentration of 5 mg mL−1 and analysed by ESI-IT-MSn. Prior to injection into the UHPLC-MS, all extracts and standards were centrifuged at 15
000g for 5 min at RT.
2.4. Reversed phase liquid chromatography (RP-UHPLC-PDA)
Water extracts, spent extracts, and standards were separated on a Thermo Vanquish UHPLC system (Thermo Scientific, San Jose, CA, USA) equipped with a pump, degasser, autosampler and photodiode array (PDA) detector. Identical column, eluents, and gradient elution program were used as reported by van Dinteren et al.13
2.5. Electrospray ionisation ion trap mass spectrometry (ESI-IT-MSn)
Mass spectrometric data were acquired using a LTQ Pro linear ion trap mass spectrometer (Thermo Scientific) equipped with a heated ESI probe coupled in-line to the Vanquish UHPLC system, as described elsewhere.13 For the water extracts, data were collected in negative ionisation (NI) and positive ionisation (PI) mode between m/z 200–2000. Besides, data-dependent MSn analyses by collision-induced dissociation with a normalized collision energy (NCE) of 35%, also NCE of 20% was used. MSn fragmentation was performed on the most intense product ion in the MSn−1 spectrum, as well as on characteristic fragments related to prenylation in PI mode ([M + H − C3H6]+ and [M + H − C4H8]+).
2.6. Electrospray ionisation hybrid quadrupole orbitrap mass spectrometry (ESI-FT-MSn)
Accurate mass data were acquired using a Thermo Q Exactive Focus hybrid quadrupole-Orbitrap Fourier transform mass spectrometer (FT-MS) (Thermo Scientific) equipped with a heated ESI probe, as described elsewhere.13
2.7. Identification of prenylated phenolics in water and spent extracts
Prenylated phenolics were tentatively identified based on our recently developed prenylation decision guideline,13 based on UV-Vis, and MS spectral data, obtained by Xcalibur 4.1. A summary of different UV-Vis absorption spectra and retro-Diels–Alder (RDA) fragmentation patterns is provided in Table B1 (ESI†).
2.8. Quantification of prenylated phenolics in water and spent extracts
Quantification of phenolics was based on their UV absorbance at 280 nm, applying a cut-off rule that the peak should account for at least 0.75% of the total UV area at 280 nm. For this, six-point (0.1–100 μg mL−1) calibration curves based on external standards of glab, licoA, and glycy were used (R2 = 0.999). UV peaks were integrated using the AVALON integration algorithm with the auto-calc function (Xcalibur 4.1). Subsequently, content of each compound was corrected for the differences in molar extinction coefficients between the standard and the compounds of interest, using a derivative of Lambert-Beer's law (eqn (1)). |  | (1) |
In which Ci is the concentration of the compound that is quantified, Area280 is the integrated area of the UV peak at 280 nm, kstandard is the response factor or slope of the standard (glab, licoA, or glycy), ε (AU M−1 cm−1) is the molar extinction coefficient, and Mw is the molecular weight (g mol−1). Because the ε-values of most tentatively identified compounds were unavailable, for each (iso)flavonoid subclass a representative compound was chosen and its ε reported in literature was used (corrected for the wavelength using
, in which A is the UV absorbance, i is the wavelength at which the compound is quantified, and r is the wavelength reported in literature, Table C1, ESI†).
To evaluate the effect of the sample matrix on the performance of the quantification method, EtOAc spent extracts of G. glabra, G. inflata, and G. uralensis spent were spiked with glab, licoA, or glycy at 25 and 50 μg mL−1. Recovery was calculated with eqn (2).
| 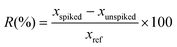 | (2) |
In which xspiked is the measured concentration of the analyte in the spiked experiment, xunspiked is the measured concentration of the analyte in the unspiked experiment, and xref is the true concentration which was spiked to the extract. Recovery of glab ranged between 97–102%, of licoA between 104–120%, and of glycy between 120–128%. Obtained recoveries were acceptable, as a good recovery should be between 80–120%.30
2.9. Antimicrobial activity assay by the broth microdilution assay
Bacteria (L. buchneri ATCC 4005, L. buchneri L4, S. mutans ATCC 27175, S. aureus ATCC 25923, E. coli K12, E. coli O54:H21, E. coli O88:H8), and yeasts (Y. lipolytica food isolate, Z. parabailli ATCC 60483, Z. parabailli UL 3699) were streaked from a glycerol stock to their optimal growth medium agar plate and incubated for 24–72 h for bacteria and 72 h for yeasts at 25–37 °C. One colony was transferred to corresponding broth and further incubated for 18–24 h at the microorganisms’ optimal growth temperature. Overnight cultures were diluted 50–10
000× to obtain an initial inoculum concentration of ∼5.0
log10 CFU mL−1. An overview of the optimal growth media, incubation temperatures, incubation times, and final initial inoculums of each microorganism is shown in Table D1 (ESI†).
Stock solutions of the liquorice spent extracts (100 mg mL−1) and species-specific marker compounds glab, licoA, and glycy (20 mg mL−1) were prepared in DMSO. Stock solutions of control compounds chlorhexidine, ampicillin, and carvacrol were prepared in DMSO at 20, 10, and 10 mg mL−1, respectively. Potassium sorbate stock was prepared at 250 mg mL−1 in MQ. Equal volumes (100 μL) of bacterium or yeast inoculum and a series of 2-fold dilutions of liquorice spent extracts (25–2000 μg mL−1, microorganism-dependent) and marker compounds (3.125–200 μg mL−1, microorganism-dependent) in growth medium were mixed into a 96-wells plate (maximum concentration of DMSO concentration was 2.0% (v/v), which did not affect bacterial/fungal growth, Table M1, ESI†). The 96-wells plate was incubated in a Spectramax ID3 (Molecular Devices, Sunnyvale, CA, USA) or in a Tecan Infinite 200Pro (Tecan Group Ltd, Zürich, Swiss) for 24–72 h. Microbial incubation was set at the microorganisms’ optimal growth temperature and time (Table D1†) with double orbital and high intensity shaking before each measurement and medium intensity shaking in-between each measurement for 5 seconds. Tecan incubation was set at the microorganisms’ optimal growth temperature and time (Table D1†) applying orbital shaking with an amplitude of 3 mm and frequency of 218.3 rpm, before each measurement for 5 seconds.
Growth was determined by measuring the optical density (OD) at 600 nm every 10 min. Time to detection (TTD) was defined as the time to reach a change in OD of 0.05 units.31 When no reliable TTD could be obtained, growth delay ratio was determined; growth delay ratio was defined as the ratio between intercept B in the presence of an antimicrobial compound and intercept A in the absence of antimicrobial compound (negative control), as is shown in Fig. G1.† Positive controls (chlorhexidine for S. mutans and S. aureus, carvacrol and ampicillin for E. coli, potassium sorbate for L. buchneri, Z. parabailli, and Y. lipolytica), negative controls (growth medium suspension of bacteria/yeasts with 2.0% (v/v) DMSO), and blanks (extracts and growth medium without bacteria/yeasts) were considered for optical comparison and sterility control. Liquorice spent extracts and species-specific markers were tested in three biological replicates, in duplicate or triplicate.
When ΔOD was less than 0.05 (or when flat lines after the incubation period were obtained), viable cell count was performed. In brief, 100 μL from each well was decimally diluted in PPS and spread onto optimal growth medium agar plate. Plates were incubated at the optimum growth temperature and time (Table D1†) after which colonies were counted.
The minimum inhibitory concentration (MIC) was determined as the lowest concentration of extract or compound that resulted in a cell count equal or lower than that of the initial inoculum. The minimum bactericidal or fungicidal concentration (MBC or MFC) was determined as the lowest concentration of extract or compound that resulted in >99% bacteria or yeast inactivation from the initial inoculum. Minimum inhibitory prenylated phenolics concentrations (MIPPC) and minimum bactericidal/fungicidal prenylated phenolics concentrations (MBPPC/MFPPC) were determined, which was the MIC or MBC corrected for the content of prenylated phenolics. For glab, licoA, and glycy the MIC/MBC/MFC was the same as the MIPPC/MBPPC/MFPPC.
To determine the role of molecular efflux pumps in the resistance of E. coli towards the liquorice spent extracts, the efflux pump inhibitor phenylalanine–arginine β-naphthylamide dihydrochloride (PaβN) was added with the liquorice spent extracts during the broth microdilution assay. PAβN inhibits AcrAB-TolC, the most prominent efflux pump in E. coli.32–34 PAβN was added in growth medium at 25 μg mL−1 (which did not affect bacterial growth, data not shown).
Natural compounds with a MIC ≤15 μg mL−1 are considered highly active antimicrobial compounds, with a MIC between 15–25 μg mL−1 they are classified as active antimicrobial compounds, compounds with MICs between 25–100 μg mL−1 are considered moderately active antimicrobials, and those with MICs >100 μg mL−1 are considered as inactive.19
2.10. Cell culture
Caco-2 cells were cultured in Dulbecco's Modified Eagle's supplemented with glucose (4.5 g L−1), HEPES buffer solution (0.58 g L−1), L-glutamine (0.58 g L−1), 10% (v/v) heat-inactivated fetal calf serum, and 1% (v/v) penicillin and streptomycin, in a humidified atmosphere of 5% CO2 at 37 °C until 80–90% confluency. Cells between passages 14 and 33 were used. To assess cell cytotoxicity (section 2.11) and cell viability (section 2.12), cells were seeded into 96-wells plates at a density of 15
000 cells per cm2 (or 4800 cells per well). Cells were differentiated for 21 days and medium was changed every 48 h.
2.11. Cell cytotoxicity
Cytotoxic effects of EtOAc spent extracts of G. glabra, G. inflata, and G. uralensis (12.5–1000 μg mL−1) and marker compounds glab, licoA, and glycy (3.125–100 μg mL−1) on Caco-2 cells were assessed after 4 h of incubation, by measuring leakage of intracellular lactate dehydrogenase (LDH) in supernatant and analysis using an LDH cytotoxicity detection kit (Roche Applied Science, Almere, The Netherlands), according to manufacturer's instructions. Liquorice spent extracts and marker compounds were dissolved in DMSO at 100 mg mL−1 and 10 mg mL−1, respectively. Highest concentration of DMSO in measurements was 1% (v/v), which did not affect cell cytotoxicity (Fig. N1, ESI†). LDH activity in the supernatant was expressed as percentage of the maximum releasable LDH in cells (Caco-2 cells treated with 1% (v/v) Triton X-100) and calculated with eqn (3).HH |  | (3) |
here, exp. value is UV absorbance at 492 nm (Multiskan Ascent, Thermo Fisher Scientific), low control is the spontaneous LDH release in untreated cells (Caco-2 cells in culture medium), and high control is the maximum releasable LDH in cells (Caco-2 cells treated with 1% (v/v) Triton X-100). Liquorice extracts and standards were considered cytotoxic when >20% cytotoxicity was observed.
To evaluate the stability of glab, licoA, and glycy in DMEM, 50 μg mL−1 of each standard was incubated for 4 h at 37 °C, after which glab, licoA, and glycy were quantified based on UV absorbance at 280 nm (section 2.8). For this, six-point (1–100 μg mL−1) calibration curves of external standards of glab, licoA, and glycy were used (R2 ≥ 0.999). Glab, licoA, and glycy were stable in DMEM, with recovery of 102 ± 6.3%, 101 ± 14%, and 106 ± 8.8%, respectively.
2.12. Cell viability
Effects on cell viability in Caco-2 cells by EtOAc spent extracts of G. glabra, G. inflata, and G. uralensis (12.5–1000 μg mL−1) and marker compounds glab, licoA, and glycy (3.125–100 μg mL−1) were assessed after incubation of 4 h, by measuring cleavage of the tetrazolium salt WST-1 to formazan by cellular mitochondrial dehydrogenases, and analysed using a WST-1 cell viability kit (PromoKine, Heidelberg, Germany), according to manufacturer's instructions. Cell viability was expressed as percentage of the control cells (Caco-2 cells in culture medium) and calculated with eqn (4). | 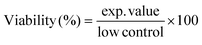 | (4) |
here, exp. value is UV absorbance at 450 nm (Multiskan Ascent, Thermo Fisher Scientific) and low control is spontaneous cleavage of WST-1 to formazan by mitochondrial dehydrogenases in untreated cells.
2.13. Statistical data analysis
Cell cytotoxicity and viability data were analysed by one-way analysis of variance (ANOVA) using IBM SPSS Statistics v.25 software (SPSS Inc., Chicago, IL, USA). Normality was confirmed by checking Q–Q plots. When equal variances were assumed (by Levene's test), significant differences (p ≤ 0.05) were compared using Dunnett's post hoc comparisons. When equal variances were not assumed, significant differences (p ≤ 0.05) were compared using Dunnett's T3 post hoc comparisons.
3. Results and discussion
3.1. Prenylated phenolics composition of Glycyrrhiza glabra, G. inflata, and G. uralensis spent extracts
Representative chromatographic profiles of the EtOAc spent extracts of G. glabra, G. inflata, and G. uralensis are shown in Fig. 2. In total, 41 peaks for G. glabra, 36 peaks for G. inflata, and 32 peaks for G. uralensis were selected (corresponding to 74%, 81%, and 75% of the total UV area at 280 nm, respectively). Using our recently developed prenylation decision guideline13 and the established fragmentation behaviour of different classes of phenolics (Table B1, ESI†), aforementioned peaks were annotated. Annotations are listed in Table E1 (IT-MSn) and Table F1 (FT-MSn) (ESI†).
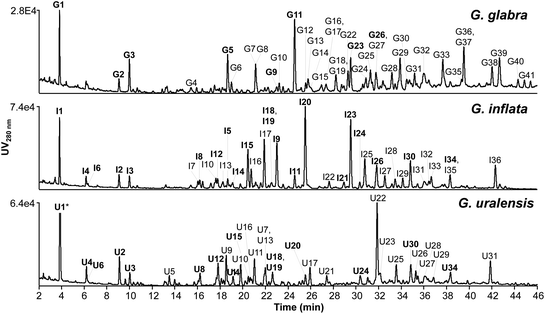 |
| Fig. 2 RP-UHPLC-PDA chromatograms (at 280 nm) of EtOAc spent extract of Glycyrrhiza glabra, G. inflata, and G. uralensis. Peaks labelled with a number indicate (tentatively) identified phenolic compounds (Table E1 and F1, ESI†). Bold similar numbers indicate the same compounds identified in at least two Glycyrrhiza species (G = G. glabra, I = G. inflata, and U = G. uralensis). * Peak U1 was cut at an intensity of 2.44 × 105. | |
The total content of annotated compounds in the EtOAc spent extracts of G. glabra, G. inflata, and G. uralensis was 105 ± 13 (10.5 ± 0.13% w/w), 187 ± 10 (18.7 ± 0.10% w/w), and 196 ± 20 (19.6 ± 0.20% w/w) mg per g DW extract, of which 96, 171, and 155 mg per g DW extract were prenylated phenolics. Estimated content of each individual compound is shown in Table H1 (ESI†). An overview of the tentative identification (in positive ionisation mode IT-MSn and HR-MS) and content of the top four major prenylated phenolics (from Table H1†) per liquorice species, including species-specific markers is shown in Table 1. In a recent study by Song and co-workers, phenolic content in methanolic root extracts of G. glabra, G. inflata, and G. uralensis was 65%.14 Methanolic extraction leads to extraction of a wider range of non-prenylated non-flavonoid phenolics, also resulting in a higher phenolics content compared to our extraction (content ranging between 10.5% and 19.6% between the different liquorice species). Non-prenylated phenolics, however, are known to have low antimicrobial activity, as was demonstrated in the work by Abreu and co-workers, in which apigenin, chrysin, daidzein, and genistein were inactive against different strains of MRSA with MICs >120 μg mL−1.35 Therefore, EtOAc extraction leads to more selective extraction of antimicrobial prenylated phenolics for liquorice roots valorisation.
Table 1 Tentative identification of the major prenylated phenolics in EtOAc extracts of G. glabra, G. inflata, and G. uralensis spent and the spectroscopic and spectrometric data obtained in positive ionisation (PI) mode in ESI-IT-MSn and ESI-FT-MS. Numbers refer to peaks in Fig. 2. Estimated content of prenylated phenolics is shown in mg per g DW liquorice spent extract
No.a |
Identified compound (Subclass) |
UVmax b (nm) |
Molecular formula |
IT-MSn |
FT-MS |
Contentc (mg per g DW extract) |
[M + H]+ (m/z) |
MS2 PI mode m/z (R.A.) |
MS3 PI mode m/z (R.A.) |
[M + H]+m/z calculated |
[M + H]+m/z observed |
Error (ppm) |
R.A. = Relative abundance. No = number corresponds to peaks in Fig. 2 and tentatively identified compounds in Tables E1 and F1 (ESI†). Underlined numbers indicate the main UV absorbance peak. Estimated content of all tentatively identified compounds is shown in Table H1 (ESI†). 3,3-DMA = 3,3-dimethylallyl chain. |
G-I-11 |
Glabridin (Isoflavan) |
![[2 with combining low line]](https://www.rsc.org/images/entities/char_0032_0332.gif) ![[7 with combining low line]](https://www.rsc.org/images/entities/char_0037_0332.gif) , 322 |
C20H20O4 |
325 |
189 (100), 203 (18) |
147 (100), 171 (24) |
325.14345 |
325.14335 |
−0.26 |
8.68 ± 0.71 (G. glabra) |
3.59 ± 0.25 (G. inflata) |
G18 |
Kanzonol Y/Glycybridin C (2′-hydroxy-dihydrochalcone) |
282, 338 |
C25H30O5 |
411 |
175 (34), 337 (16), 355 (19), 365 (9), 393 (100) |
175 (100), 337 (33), 365 (11) |
411.21660 |
411.21698 |
0.92 |
5.93 ± 0.56 |
G33 |
Hispaglabridin A (Isoflavan) |
![[2 with combining low line]](https://www.rsc.org/images/entities/char_0032_0332.gif) ![[7 with combining low line]](https://www.rsc.org/images/entities/char_0037_0332.gif) , 330 |
C25H28O4 |
393 |
191 (72), 337 (100) |
149 (23), 161 (16), 173 (12), 177 (10), 187 (37), 189 (100), 201 (32), 203 (22), 295 (22), 319 (10) |
393.20604 |
393.20609 |
0.14 |
6.51 ± 0.63 |
G39 |
Hispaglabridin B (Isoflavan) |
278 |
C25H26O4 |
391 |
147 (8), 189 (100) |
147 (100), 171 (22) |
391.19038 |
391.19034 |
−0.12 |
5.10 ± 0.49 |
G-I-9 |
Glabrocoumarin (3-Arylcoumarin) |
350 |
C20H16O5 |
337 |
267 (11), 283 (34), 295 (100), 296 (10), 309 (41), 319 (41) |
137 (12), 239 (78), 267 (100), 268 (10), 277 (8), 295 (87) |
337.10704 |
337.10699 |
−0.018 |
10.16 ± 0.45 |
I17 |
Licochalcone A (Chalcone) |
314, ![[3 with combining low line]](https://www.rsc.org/images/entities/char_0033_0332.gif) ![[7 with combining low line]](https://www.rsc.org/images/entities/char_0037_0332.gif) ![[8 with combining low line]](https://www.rsc.org/images/entities/char_0038_0332.gif) |
C21H22O4 |
339 |
219 (10), 245 (21), 271 (30), 297 (100) |
191 (100), 203 (31), 227 (27), 229 (17), 237 (17), 241 (11), 255 (21), 265 (22) |
339.15907 |
339.15933 |
0.72 |
23.7 ± 1.01 |
I-U-20 |
Licoisoflavone B/Sophoraisoflavone A/Semilicoisoflavone B (Isoflavone) |
262 |
C20H16O6 |
353 |
299 (20), 311 (100), 325 (30), 335 (22) |
153 (24), 255 (58), 283 (100), 284 (10), 293 (12),311 (54) |
353.10197 |
353.10184 |
−0.35 |
23.69 ± 1.71 (G. inflata) |
2.67 ± 0.28 (G. uralensis) |
G-I-23 |
Glabrol (Flavanone) |
![[2 with combining low line]](https://www.rsc.org/images/entities/char_0032_0332.gif) ![[8 with combining low line]](https://www.rsc.org/images/entities/char_0038_0332.gif) , 318 |
C25H28O4 |
393 |
205 (13), 337 (100) |
177 (27), 191 (16), 195 (52), 203 (17), 213 (100), 281 (19), 295 (22), 319 (63), 322 (27) |
393.20604 |
393.20599 |
−0.12 |
20.86 ± 1.13 (G. inflata) |
4.47 ± 0.47 (G. glabra) |
U7 |
n.i. O-3,3-DMAd (Chalcone) |
370 |
C21H22O5 |
355 |
287 (100), 299 (70) |
165 (13), 231 (11), 241 (14), 287 (100) |
355.15400 |
355.15396 |
−0.11 |
14.20 ± 0.60 |
U9 |
Glycycoumarin (3-Arylcoumarin) |
350 |
C21H20O6 |
369 |
285 (45), 301 (5), 313 (100), 314 (13), 327 (14), 341 (11) |
149 (19), 191 (19), 209 (11), 243 (17), 257 (10), 271 (27), 281 (23), 283 (32), 285 (100), 298 (14) |
369.13325 |
369.13330 |
0.10 |
18.52 ± 1.25 |
U22 |
Licoricidin (Isoflavan) |
![[2 with combining low line]](https://www.rsc.org/images/entities/char_0032_0332.gif) ![[8 with combining low line]](https://www.rsc.org/images/entities/char_0038_0332.gif) , 338 |
C26H32O5 |
425 |
191 (11), 221 (19), 369 (100) |
167 (85), 189 (100), 191 (17), 219 (17), 233 (17), 313 (45), 351 (13) |
425.23225 |
425.23178 |
1.11 |
32.64 ± 2.07 |
U31 |
Licorisoflavan A (Isoflavan) |
![[2 with combining low line]](https://www.rsc.org/images/entities/char_0032_0332.gif) ![[8 with combining low line]](https://www.rsc.org/images/entities/char_0038_0332.gif) , 342 |
C27H34O5 |
439 |
371 (47), 383 (100) |
181 (100), 189 (86), 191 (20), 193 (15), 215 (12), 327 (58) |
439.24791 |
439.24805 |
0.34 |
10.04 ± 0.61 |
Content of species-specific marker compounds glab (G-I-11, G. glabra), licoA (I17, G. inflata), and glycy (U9, G. uralensis) was 8.7 ± 0.7, 23.7 ± 1.1, and 18.5 ± 1.3 mg per g DW extract, respectively (corresponding to 0.24 ± 0.02, 0.54 ± 0.02, and 0.86 ± 0.06 mg per g dry roots) (Table H1, ESI†). These results are in line with reported average contents of 0.028–1.0, 0.3–3.8, and 0.018–1.1 mg per g DW roots for glab, licoA, and glycy in 95 batches of G. glabra, G. inflata, and G. uralensis, respectively.14 These results indicate that liquorice spent is an equally rich source in prenylated phenolics compared to liquorice roots.
Special attention was given to peaks I20 (licoisoflavone B or sophoraisoflavone A or semilicoisoflavone B) in G. inflata and U22 (licoricidin) in G. uralensis (Fig. 2), as these prenylated compounds showed the highest peak intensity at 280 nm.
Content of I20 and U22 was 23.7 ± 1.7 and 32.6 ± 2.1 mg per g DW extract (corresponding to 0.54 ± 0.04 and 1.51 ± 0.1 mg per g DW roots) (Table H1, ESI†), respectively. For peak I20, we could not differentiate between B-ring 2,2-dimethylpyran (2,2-DMP) ring prenylated isomers licoisoflavone B, sophoraisoflavone A, and semilicoisoflavone B with our recently developed prenylation guideline.13 Licoisoflavone B, sophoraisoflavone A, and semilicoisoflavone B have been identified and quantified in G. inflata before, with contents ranging between 9–463, 1.0–16, and 0.21–31 μg per g DW roots, respectively. Also licoricidin has been identified and quantified in G. uralensis roots, with contents ranging between 11.1–1507 μg per g DW roots.14
Analysis of phenolic compound distribution in the EtOAc spent extracts of G. glabra, G. inflata, and G. uralensis showed that the majority of compounds belonged to the isoflavonoids (Fig. 3A), with 52 ± 4.3, 93 ± 4.8, and 117 ± 6.9 mg per g DW extract, respectively (or 49 ± 1.9, 50 ± 0.24, and 60 ± 0.20% (w/w) extract). With respect to subclass, G. glabra and G. uralensis were particularly rich in isoflavans with contents of 32 ± 3.0 and 48 ± 3.2 mg per g DW extract, respectively (or 31 ± 0.93% and 25 ± 0.26% (w/w) extract), and isoflavones representing the majority in G. inflata with 73 ± 4.0 mg per g DW extract (or 39 ± 0.12% (w/w) extract). Surprisingly, our results demonstrate that chalcones were not particularly abundant in G. inflata (29 ± 1.2 mg per g DW extract or 15 ± 0.21% (w/w) extract), as was shown by Song and co-workers, in which approximately 50% of non-glycosylated prenylated phenolics in G. inflata were chalcones. This difference might be explained by batch variability (e.g. differences in harvest time, origin, cultivation).14
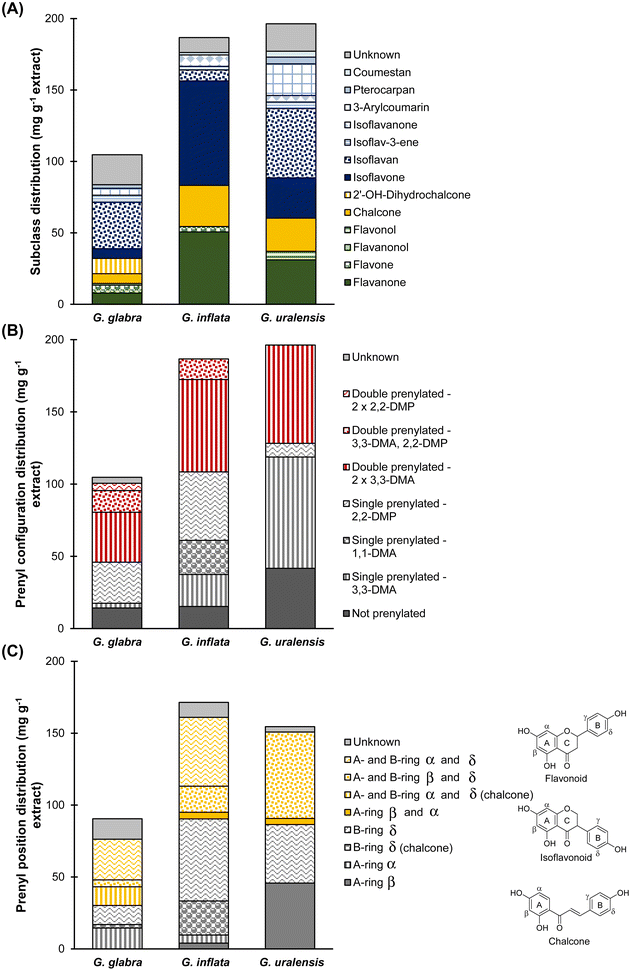 |
| Fig. 3 Weight distribution of (A) phenolic subclass, (B) prenyl configuration, and (C) prenyl position in EtOAc spent extracts Glycyrrhiza glabra, G. inflata, and G. uralensis. | |
Further analysis focused on prenylation (Fig. 3B) showed that G. glabra was abundant in double prenylated compounds with 55 ± 8.4 mg per g DW extract (or 52 ± 1.7% (w/w) extract), and G. inflata and G. uralensis were rich in single prenylated compounds with 93 ± 4.7 and 86 ± 6.3 mg per g DW extract, respectively (or 50 ± 0.15% and 44 ± 0.52% (w/w) extract). Regarding prenyl configuration, the majority of double prenylated compounds in G. glabra were double 3,3-dimethylallyl (3,3-DMA) chain prenylated (35 ± 6.8 mg per g DW extract, or 33 ± 2.5% (w/w) extract). The single prenylated compounds in G. inflata were equally 3,3-DMA chain or 1,1-dimethylallyl (1,1-DMA) chain (with 46 ± 1.8 mg g−1 chain prenylated or 24 ± 0.34% (w/w) extract) and 2,2-DMP ring prenylated (with 47 ± 2.9 mg per g ring prenylated or 25 ± 0.22% (w/w) extract). Lastly, the majority of single prenylated compounds in G. uralensis were 3,3-DMA chain prenylated (77 ± 5.5 mg per g DW extract or 39 ± 0.41% (w/w) extract). Regarding the prenyl position (Fig. 3C), the majority of double prenylated compounds in G. glabra were A- and B-ring prenylated with 46 mg per g DW extract (or 48 ± 1.1% (w/w) extract); the majority of single prenylated compounds in G. inflata were B-ring prenylated with 81 ± 4.3 mg per g DW extract (or 47 ± 0.14% (w/w) extract), and the single prenylated compounds in G. uralensis were equally A- and B-ring prenylated with 46 ± 4.1 and 41 ± 2.3 mg per g DW extract, respectively (or 30 ± 0.62% and 26 ± 0.34% (w/w) extract) (Fig. 3C).
To conclude, spent extracts of G. glabra, G. inflata, and G. uralensis showed a distinct compositional profile (Fig. 2), in which species-specific compounds glab (G. glabra), licoA (G. inflata), and glycy (G. uralensis) were the main prenylated phenolics present, with 8.7 ± 0.7, 23.7 ± 1.1, and 18.5 ± 1.3 mg per g DW extract, respectively, next to licoisoflavone B or sophoraisoflavone A or semilicoisoflavone B with 23.7 ± 1.7 mg per g DW spent extract in G. inflata, and licoricidin with 32.6 ± 2.1 mg per g DW spent extract in G. uralensis.
3.2. Antimicrobial activity of liquorice spent extracts and species-specific markers glabridin, licochalcone A, and glycycoumarin
EtOAc spent extracts of G. glabra, G. inflata, and G. uralensis and species-specific marker compounds glab, licoA, and glycy were tested for their antimicrobial activity against L. buchneri, Z. parabailli, Y. lipolytica, S. mutans, S. aureus, and E. coli. Minimum inhibitory concentrations (MIC) and minimum bactericidal/fungicidal concentrations (MBC/MFC) are shown in Table 2.
Table 2 Minimum inhibitory concentrations (MIC) and minimum bactericidal/fungicidal concentrations (MBC/MFC) in μg mL−1 of liquorice spent extracts and marker compounds against various microorganisms
Bacteria/yeasts |
G. glabra
|
G. inflata
|
G. uralensis
|
Glabridin |
Licochalcone A |
Glycycoumarin |
MIC (LRa ± SDb) |
MBC/MFC (LR ± SD) |
MIC (LR ± SD) |
MBC/MFC (LR ± SD) |
MIC (LR ± SD) |
MBC/MFC (LR ± SD) |
MIC (LR ± SD) |
MBC/MFC (LR ± SD) |
MIC (LR ± SD) |
MBC/MFC (LR ± SD) |
MIC (LR ± SD) |
MBC/MFC (LR ± SD) |
LR = log reduction after 24 h incubation from initial inoculum.
SD = standard deviation.
n.d. = not determined.
In the presence of efflux pump inhibitor PAβN.
|
BACTERIA
|
L. buchneri
|
ATCC 4005 |
250–500 (2.22 ± 1.54) |
250–1000 (3.14 ± 0.79) |
250 (3.55 ± 0.98) |
250 (3.55 ± 0.98) |
50 (2.66 ± 2.54) |
50–100 (4.65 ± 0.78) |
12.5–25 (2.33 ± 0.28) |
12.5–25 (2.33 ± 0.28) |
12.5 (4.47 ± 1.47) |
12.5–25 (5.07 ± 0.48) |
25 (4.04 ± 1.54) |
25 (4.04 ± 1.54) |
L4 |
250–500 (1.59 ± 1.33) |
250–1000 (3.56 ± 1.34) |
250 (4.48 ± 1.12) |
250 (4.48 ± 1.12) |
50–100 (3.94 ± 0.39) |
50–100 (3.94 ± 0.39) |
12.5–25 (2.57 ± 2.27) |
25 (5.13 ± 0.05) |
3.125–6.25 (3.75 ± 1.39) |
3.125–6.25 (3.75 ± 1.39) |
12.5 (4.80 ± 0.55) |
12.5 (4.80 ± 0.55) |
S mutans
|
ATCC 27175 |
>1000 (n.d.c) |
>2000 (n.d.) |
100 (0.59 ± 0.35) |
>1000 (n.d.) |
250 (0.75 ± 0.06) |
1000 (2.58 ± 0.12) |
12.5 (0.98 ± 0.08) |
25–50 (3.62 ± 1.20) |
12.5 (0.72 ± 0.09) |
>100 (n.d.) |
25 (1.60 ± 0.13) |
50 (4.52 ± 0.40) |
S. aureus
|
ATCC 25923 |
75 (3.71 ± 0.01) |
75 (3.71 ± 0.01) |
25–50 (1.86 ± 2.80) |
50–100 (4.88 ± 0.34) |
25 (1.92 ± 1.75) |
25–50 (3.61 ± 1.43) |
12.5–25 (3.31 ± 1.25) |
12.5–25 (4.35 ± 0.83) |
12.5 (3.68 ± 2.42) |
12.5–25 (4.88 ± 0.34) |
12.5 (0.93 ± 0.59) |
16.7–25 (4.35 ± 0.77) |
E. coli
|
K12 |
>2000 (n.d.) |
>2000 (n.d.) |
>2000 (n.d.) |
>2000 (n.d.) |
>2000 (n.d.) |
>2000 (n.d.) |
>200 (n.d.) |
>200 (n.d.) |
>200 (n.d.) |
>200 (n.d.) |
>200 (n.d.) |
>200 (n.d.) |
|
|
|
|
|
|
|
12.5–25d (5.23 ± 0.08) |
12.5–25d (5.23 ± 0.08) |
>100d (n.d.) |
>100d (n.d.) |
25d (5.23 ± 0.08) |
25d (5.23 ± 0.08) |
O54:H21 |
>2000 (n.d.) |
>2000 (n.d.) |
>2000 (n.d.) |
>2000 (n.d.) |
>2000 (n.d.) |
>2000 (n.d.) |
>100 (n.d.) |
>100 (n.d.) |
>100 (n.d.) |
>100 (n.d.) |
>100 (n.d.) |
>100 (n.d.) |
O88:H8 |
>2000 (n.d.) |
>2000 (n.d.) |
>2000 (n.d.) |
>2000 (n.d.) |
>2000 (n.d.) |
>2000 (n.d.) |
>100 (n.d.) |
>100 (n.d.) |
>100 (n.d.) |
>100 (n.d.) |
>100 (n.d.) |
>100 (n.d.) |
|
YEASTS
|
Y. lipolytica
|
Food isolate |
>2000 (n.d.) |
>2000 (n.d.) |
>2000 (n.d.) |
>2000 (n.d.) |
>2000 (n.d.) |
>2000 (n.d.) |
>25–≤50 (4.94 ± 0.23) |
50 (4.94 ± 0.23) |
>100 (n.d.) |
>100 (n.d.) |
100 (4.90 ± 0.17) |
100 (4.90 ± 0.17) |
Z. parabailli
|
ATCC 60483 |
>2000 (n.d.) |
>2000 (n.d.) |
>2000 (n.d.) |
>2000 (n.d.) |
>2000 (n.d.) |
>2000 (n.d.) |
>12.5–≤25 (4.34 ± 1.29) |
25 (4.34 ± 1.29) |
>100 (n.d.) |
>100 (n.d.) |
>100 (n.d.) |
>100 (n.d.) |
UL 3699 |
>2000 (n.d.) |
>2000 (n.d.) |
>2000 (n.d.) |
>2000 (n.d.) |
>2000 (n.d.) |
>2000 (n.d.) |
12.5–25 (3.62 ± 2.67) |
25 (5.24 ± 0.15) |
>100 (n.d.) |
>100 (n.d.) |
100 (4.19 ± 0.98) |
100 (4.19 ± 0.98) |
3.2.1. Antibacterial activity.
Liquorice spent extracts and marker compounds glab, licoA, and glycy showed antibacterial activity against the Gram-positive bacteria S. aureus, S. mutans, and L. buchneri, whereas no activity was seen against the Gram-negative bacterium E. coli (Table 2). For example, liquorice spent extracts inhibited growth of S. aureus, with MICs of 75, 25–50, and 25 μg mL−1 for G. glabra, G. inflata, and G. uralensis, respectively (with log reductions (LRs) of 3.7 ± 0.01, 1.9 ± 2.8, and 1.9 ± 1.7). At these MICs, the content of prenylated phenolics (minimum inhibitory prenylated phenolics concentration, or MIPPC, (Table I1, ESI†)) was 7.2 μg mL−1 for G. glabra, 4.3–8.6 μg mL−1 for G. inflata, and 3.9 μg mL−1 for G. uralensis. In contrast, liquorice spent extracts did not show antibacterial activity up to 2000 μg mL−1 against different E. coli strains (with MIPPCs >192, >342, and >310 μg mL−1 for G. glabra, G. inflata, and G. uralensis, respectively, Table I1†). The same trend was observed for the marker compounds. Glab, licoA, and glycy showed anti-S. aureus activity with MICs between 12.5–25 μg mL−1 (with LRs of 3.3 ± 1.3, 3.7 ± 2.4, and 0.9 ± 0.6), which is associated with good activity,19 whereas no anti-E. coli activity was seen up to 200 μg mL−1 (Table 2). Gram-negative bacteria are known to have higher intrinsic resistance towards most antimicrobials than Gram-positive bacteria, caused by (i) the limited permeability by the highly ordered and crystalline lipopolysaccharides (LPS) in the outer bacterial membrane, (ii) size restrictions of hydrophilic porins (generally Mw < 600–700), and (iii) effective excretion of antimicrobials across the double membrane by double-membrane spanning efflux pumps.33,36 Addition of efflux pump inhibitor PAβN did not increase susceptibility towards the liquorice spent extracts up to 2000 μg mL−1 (data not shown). However, addition of PAβN increased susceptibility of E. coli K12 towards glab and glycy, as MICs decreased from >100 μg mL−1 to 12.5–25 μg mL−1, respectively (Table 2). These results demonstrate that glab and glycy can enter the outer membrane of E. coli, and that intrinsic resistance of E. coli was due to active efflux rather than limited influx. As the liquorice spent extracts are rich in prenylated phenolics, we propose that the extracts interact with the bacterial cytoplasmic membrane, thereby changing membrane fluidity, which can lead to membrane permeabilization, and bacterial cell death.20,37,38
Large differences in susceptibility (MICs) between Gram-positive bacteria towards the liquorice spent extracts were observed (Table 2). S. aureus was the most susceptible towards the spent extracts, with MICs between 25 and 75 μg mL−1, whereas MICs against S. mutans were ranging between 100 μg mL−1 for G. inflata, 250 μg mL−1 for G. uralensis, and >1000 μg mL−1 for G. glabra. Liquorice spent extracts showed bactericidal activity against S. aureus, as the ratio MBC/MIC was <4 (Table 2).39 In contrast, G. inflata spent extract was bacteriostatic against S. mutans, shown by the ratio MBC/MIC >4.39 The underlying mechanism behind this increased susceptibility of S. aureus compared to S. mutans is unknown. Possibly, the production of a glue-like extracellular polymer glucan (that promotes formation of a biofilm) in S. mutans provides an extra barrier against the prenylated phenolics by complicating the interaction with the bacterial cell membrane.40
In some cases, antibacterial activity of marker compounds was found to correlate with the antibacterial activity of the liquorice spent extracts; for example, the MIPPC of G. inflata against S. mutans was 17.1 μg mL−1 (Table I1, ESI†), and the MIC of licoA was 12.5 μg mL−1 (Table 2). This might hint that the marker prenylated phenolic in G. inflata spent extract is the main contributor to its antibacterial activity. In contrast, we observed that the MIPPC of G. uralensis extract against S. aureus (3.9 μg mL−1) was about 3-fold lower than the MIC of the marker glycy (12.5 μg mL−1) (Table I1,†Table 2). This might be explained by other potent antibacterial prenylated phenolics in our EtOAc spent extract of G. uralensis. For example, the double chain prenylated isoflavone 6,8-diprenylgenistein (4.2 ± 0.2 mg per g DW extract, Table H1, ESI†) has shown anti-MRSA activity with a MIC of 9 μg mL−1,37 and the double chain prenylated isoflavan licorisoflavan A (10.0 ± 0.6 mg per g DW extract, Table H1†) showed a MIC between 1–4 μg mL−1 against different species of S. mutans.41 We cannot rule out that prenylated phenolics show synergistic effects with each other, as was shown by Chen and co-workers,42 or with other minor or unknown compounds (small amounts of non-prenylated phenolics and triterpenoid aglycones were found in the liquorice spent extracts). However, the identified non-prenylated phenolics (e.g.G-I-U-1 to G-I-U-3, Table H1, ESI†) and triterpenoid (G37, Table H1†) are not expected to significantly contribute to the antibacterial activity observed.7,35,43,44
In summary, G. glabra, G. inflata, and G. uralensis spent extracts and marker compounds glab, licoA, and glycy showed antibacterial activity against Gram-positive bacteria L. buchneri, S. mutans, and S. aureus. The order of antibacterial potency for the extracts was G. uralensis (MICs 25–250 μg mL−1) ≈ G. inflata (MICs 25–250 μg mL−1) > G. glabra (MICs 75 → 1000 μg mL−1), whereas marker compounds were equally potent (MICs 12.5–25 μg mL−1). No antibacterial activity was observed against the Gram-negative bacterium E. coli up to 2000 μg mL−1 for the liquorice spent extracts, and 200 μg mL−1 for marker compounds, without presence of efflux pump inhibitor PAβN. Antibacterial activity of glab and glycy was observed upon addition of PAβN (as MICs reduced from >200 to 12.5–25 μg mL−1).
3.2.2. Antifungal activity.
Liquorice spent extracts did not show antifungal activity against Z. parabailli and Y. lipolytica, with MICs >2000 μg mL−1 of crude extract (MIPPCs of >192, >342, and >310 μg mL−1 for G. glabra, G. inflata, and G. uralensis, respectively) (Table 2 and Table I1, ESI†). However, at 2000 μg mL−1, G. glabra, G. inflata, and G. uralensis spent extracts showed delay in fungal growth of Y. lipolytica, with growth delay ratios of 2.8 ± 1.6, 2.7 ± 0.7, and 3.0 ± 1.3, as is shown in Table K1 (ESI†). Against Z. parabailli, this growth delay ratio was less pronounced, with maximum delays for G. uralensis (ratio 2.0 ± 0.1 for ATCC 60483 and ratio 2.8 ± 0.1 for UL 3699), and no growth delay for G. glabra (ratio 1.3 ± 0.1 for ATCC 60483 and 1.1 ± 0.1 for UL 3699). Aforementioned higher growth delay ratio's in G. uralensis extract might be explained by the higher content of prenylated phenolics in the extract (155 vs. 96 mg g−1 prenylated phenolics DW extract for G. uralensis and G. glabra, respectively) (section 3.1). Additionally, G. uralensis extract is rich in single prenylated compounds, whereas G. glabra is more abundant in double prenylated compounds. It was recently shown by Kalli and co-workers that single prenylated (iso)flavonoids possess superior anti-Z. parabailli activity compared to double prenylated (iso)flavonoids (section 3.1).45 All growth delay ratio's at the different concentrations tested are shown in Table K1 (ESI†).
Glab showed good antifungal activity against Z. parabailli, with a MIC between 12.5–25 μg mL−1, which corresponded to the reported MIC (against Z. parabailli) of glab between 6.25–12.5 μg mL−1.45 In contrast, licoA and glycy showed weak antifungal activity, with MICs of >100 μg mL−1 and 100 μg mL−1, respectively. When comparing antifungal activity of the marker compounds with the most potent natural agent against Z. parabailli, polygodial (MIC 50 μg mL−1), fungicidal activity of glab was 2–4× higher.46 Against Y. lipolytica, the species-specific marker compounds were moderately active to inactive, with a MIC of 50 μg mL−1 for glab, >100 μg mL−1 for licoA, and 100 μg mL−1 for glycy, respectively.
Recently, it was proposed that antifungal activity of prenylated (iso)flavonoids was associated with i.a. their overall hydrophobicity and the location of hydrophobic moieties, which are involved in fungal membrane permeabilization and consequently fungal cell death.45 The same authors suggested that prenylated (iso)flavonoids with a log
D (lipophilicity) value ≤4.5 possess antifungal activity, whereas very hydrophobic molecules (log
D > 5.0) were inactive. Possibly, hydrophobic molecules, such as licoA (with a MIC of >100 μg mL−1 and a log
D value of 4.8, Table J1, ESI†), are not able to cross the complex hydrophilic cell wall of yeasts. The location of hydrophobic moieties (described with the hydrophobic integy moment, Table J1†) was also shown to be important for effective hydrophobic interactions with the lipid core of the fungal membrane.45 Potent antifungal prenylated (iso)flavonoids showed a hydrophobic integy moment >0.9 Å.45 Besides the higher hydrophobicity, this could also also explain the inactivity of licoA, which has a hydrophobic integy moment of 0.5 Å (Table J1, ESI†). The difference in antifungal activity between glab (MIC 12.5–25 μg mL−1, Table 2) and glycy (100 μg mL−1, Table 2) can possibly be explained by their flexibility. We previously postulated that flexible prenylated (iso)flavonoids interact more effectively inside a bacterial membrane than less flexible prenylated (iso)flavonoids, leading to disruption of membrane integrity.23 In the present study, similarly to that of a set of prenylated (iso)flavonoids tested against Z. parabailli (Table J1, ESI†),45 the opposite effect was observed. Less flexible compounds, such as glab (with a flexibility index of 2.9) or dehydroglyceollin I (a pterocarpene with a flexibility index of 2.2 and a MIC of 12.5–25 μg mL−1, Table J1†) were more potently antifungal than more flexible compounds, such as glycy (with a flexibility index of 4.1) or 6′-prenylpiscidone (a isoflavone with a flexibility index of 5.9 and a MIC of ≫25 μg mL−1, Table J1†). However, this does not explain the inactivity (>100 μg mL−1) of licoA with a flexibility index of 4.2. We suggest that a proper balance between hydrophobicity, hydrophobic integy moment, and flexibility is considered optimal for effective interactions with the fungal membrane.45,47
To summarize, the liquorice spent extracts did not show antifungal activity against Z. parabailli and Y. lipolytica at the highest concentrations tested (MIC >2000 μg mL−1), although fungal growth delay (ratios ranging between 1.1–2.5), especially for G. uralensis, was seen at 2000 μg mL−1 (with a ratio between 2.0–2.8). Marker compound glab showed good activity against Z. parabailli (MIC 12.5–25 μg mL−1) and moderate activity against Y. lipolytica (MIC 50 μg mL−1). Overall, these prenylated compounds showed higher or similar anti-yeast activity than the reported natural agent polygodial.
3.3. Cytotoxicity and effects on cell viability of liquorice spent extracts and species-specific markers glabridin, licochalcone A, and glycycoumarin
To evaluate potential effect of liquorice spent extracts and species-specific markers on cell cytotoxicity and viability, the release of LDH (Fig. 4A and B) and cellular mitochondrial dehydrogenase activity (WST-1) (Fig. 4C and D), respectively, were measured in human colorectal adenocarcinoma cells (Caco-2).
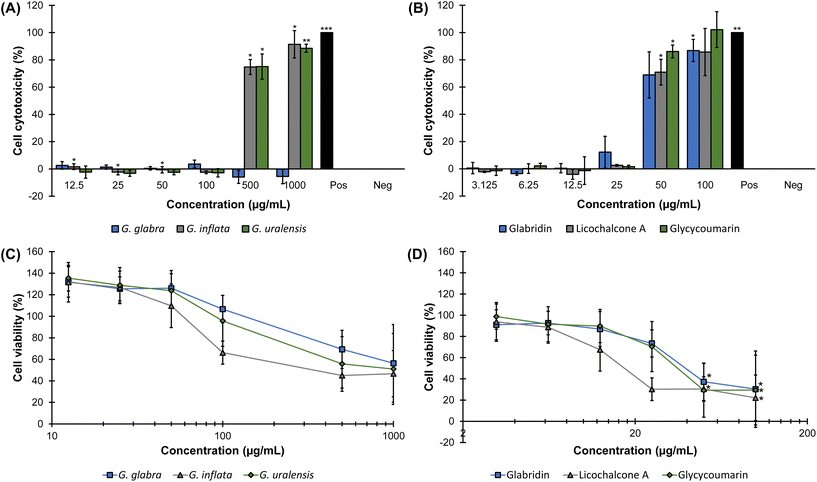 |
| Fig. 4 Cell cytotoxicity (A and B) and cell viability (C and D) of (A and C) EtOAc spent extracts of G. glabra, G. inflata, and G. uralensis and (B and D) species-specific marker compounds glabridin, licochalcone A, and glycycoumarin. Values are means ± standard deviation, measured in triplicate in three biological replicates. Cell cytotoxicity and cell viability is compared with the negative control (cells with growth medium, set at 0% for cytotoxicity and 100% for cell viability). *p < 0.05, **p < 0.01, and ***p < 0.001. | |
3.3.1. Cytotoxicity and cell viability of Caco-2 cells after exposure to liquorice spent extracts.
Spent extract of G. glabra did not show any cytotoxicity up to the highest concentration tested compared to the negative control (growth medium) (1000 μg mL−1 crude extract, MIPPC 96 μg mL−1) (Fig. 4A). In contrast, spent extracts of G. inflata (p = 0.023) and G. uralensis (p = 0.048) were cytotoxic at 500 μg mL−1 crude extract with 75% cytotoxicity for both G. inflata and G. uralensis (MIPPCs 85.5 μg mL−1 and 77.5 μg mL−1) (Fig. 4A). Cell viability of Caco-2 cells was affected at lower concentrations than cell cytotoxicity after exposure to the liquorice spent extracts. WST-1 determines metabolic activity of cells and as such is more sensitive than LDH, which is a measure of cell membrane damage. A trend of decreased cell viability was observed after exposure to G. glabra and G. uralensis spent extracts, with a decrease from 107% to 69% for G. glabra and from 96% to 56% for G. uralensis at 100 and 500 μg mL−1 crude extract (MIPPCs 9.1–45.5 and 15.5–77.5 μg mL−1) (Fig. 4C). For G. inflata spent extract, a decreased trend in cell viability from 110% to 66% was observed between 50 and 100 μg mL−1 (MIPPC 8.6–17.1 μg mL−1). Colour was visually observed at the highest concentrations of the liquorice spent extracts (>100 μg mL−1) compared to Caco-2 cells with growth medium alone. Therefore, we verified whether colour affected cell cytotoxicity and viability measurements (Fig. L1, ESI†). Cell cytotoxicity and cell viability measurements were not significantly affected by the coloured spent extracts up to 1000 μg mL−1 crude spent extract. Recently, Molčanová et al. reviewed effects of 394 prenylated phenolics with potential cytotoxic activity against cancer cells and summarized suggested structure–activity relationships (SAR) of prenylated phenolics.27 Generally (i) addition of a prenyl moiety increased cytotoxicity,48,49 (ii) single prenylated compounds showed higher cytotoxicity than double prenylated compounds,50 (iii) ring prenylated compounds (pyran [e.g. 2,2-DMP] and furan) were more cytotoxic than chain (e.g. 3,3-DMA) prenylated compounds,50 (iv) flavones and flavonols were more cytotoxic than flavanones and chalcones,51 and (v) prenylation at C8 (or α position, Fig. 3C) showed higher cytotoxicity than prenylation at position C6 (β) on the A-ring.51 The high percentage of prenylation (92%, Fig. 3B) in G. inflata spent extract of which a large part is single prenylated (50%, Fig. 3B) could explain its relatively high cytotoxicity compared to G. glabra and G. uralensis spent extracts (Fig. 4A and C). However, G. inflata spent extract is neither rich in flavones (2%) and flavonols (not detectable), nor rich in C8 (β) prenylated compounds (3%), but is relatively rich in flavanones (27%) and chalcones (15%), and C3′ (γ) prenylated compounds (33%) (Fig. 3). We hypothesize that other substitutions on the phenolic backbone, i.a. hydroxylation and methoxylation also affect cytotoxicity (and cell viability) of prenylated phenolics, as was shown by Daskiewicz and co-workers.51
Previously, it was shown that G. glabra solvent-based root extracts were cytotoxic between 7.4–34 μg mL−1 in colorectal cancer cells.52 Additionally, Jo et al. evaluated cytotoxicity of G. uralensis root extracts and reported antiproliferative effects at 50 μg mL−1 in a breast cancer cell line, which was caused by apoptosis due to cell cycle arrest.53 Prenylated phenolics were not quantified in these extracts. However, aforementioned root extracts52,53 showed cytotoxicity at lower concentrations than observed in our study (we observed cytotoxicity at 500 μg mL−1 for G. inflata and G. uralensis, and >1000 μg mL−1 for G. glabra, MIPPCs of 85.5 μg mL−1, 77.5 μg mL−1, and >96 μg mL−1, respectively, Fig. 4A). Differences in cytotoxicity could be explained by differences in prenylated phenolics content of the extracts, incubation time, and cell line.
An increased trend in apparent cell viability (or metabolic activity) in Caco-2 cells after exposure to the liquorice spent extracts <50 μg mL−1 was seen. For example, exposure to 12.5 μg mL−1 crude liquorice spent extracts increased metabolic activity 32%, 32%, and 35% for G. glabra, G. inflata, and G. uralensis, respectively (Fig. 4C). It is unknown why apparent cell viability at low concentrations was increased, but it could be hypothesized this was caused by other compounds in the liquorice spent extracts, since we did not observe an upward trend in metabolic activity for glab, licoA, and glycy (Fig. 4D). Another hypothesis is that this observed trend is an early indication of apoptosis, which is an active process that coincides with apparently increased metabolic activity. However, in general it has been shown that prenylated phenolics show antiproliferative effects and induce cell cytotoxicity in cancer cell lines.24,27,54 Interestingly, for some prenylated chalcones such as xanthohumol, the concentrations for these cytotoxic effects were much higher in primary cell lines in comparison to cancer cell lines.24–26
3.3.2. Cytotoxicity and cell viability of Caco-2 cells after exposure to glabridin, licochalcone A, and glycycoumarin.
Cytotoxicity in Caco-2 cells after exposure to the species-specific marker compounds glab (p = 0.18), licoA (p = 0.035), and glycy (p = 0.019) was observed at 50 μg mL−1 with a distinct off-on effect, with 69%, 71%, and 86% cytotoxicity, respectively, while there was no cytotoxicity measured at 25 μg mL−1 (cytotoxicity < 20%, p > 0.05) (Fig. 4B). All standards showed a similar trend (p > 0.05), where cell viability decreased to 73% at 25 μg mL−1 (77 μM) for glab, to 70% at 25 μg mL−1 (68 μM) for glycy, and to 37% at 12.5 μg mL−1 (37 μM) for licoA (Fig. 4D). At 12.5 μg mL−1 for glab and glycy, and at 6.25 μg mL−1 for licoA, no effects on cell viability were measured (viability >80% compared to the negative control, p > 0.05). Our observed cytotoxicity is in line with reported cytotoxicity of glab against other cell lines.52,55 Cytotoxic mode of action of structural similar compounds 4′-OH-methylglabridin and glabrene was related to changes in cell cycle signalling pathways, ultimately leading to apoptotic cell death via different mechanisms.52,56 It could be hypothesized that, due to structural similarity, glab shows a similar cytotoxic mode of action. Our observed cytotoxicity of licoA at 12.5 μg mL−1 is in line with previously reported cytotoxicity in different cancer cell lines.57,58 Reported cytotoxic mode of action of licoA has been linked to cell cycle arrest that leads to apoptosis.59 Lastly, our observed decreased viability (from 90% to 70% cell viability compared to the negative control, Fig. 4D) between 12.5 and 25 μg mL−1 of glycy is in line with reported cytotoxicity in cancer cells.60,61 Besides in vitro cytotoxicity in cancer cells, glycy has also shown selective anti-cancer activity in Hep-G2 xenograft mice, due to cell cycle arrest and subsequent apoptosis.61 It should be noted that we did not take into account possible interactions, absorption, distribution, and metabolism of prenylated phenolics in the gastrointestinal tract. Notably, it was previously shown that there was no significant first-pass metabolism of prenylated phenolics (i.a. licoA and licochalcone C) during intestinal absorption in a Caco-2 monolayer model.62 Nevertheless, possible interactions (e.g. with proteins), but also phase I and II metabolism, including glucuronidation, sulfation, and methylation63 of prenylated phenolics need to be further investigated.
To summarize, our results demonstrate that EtOAc spent extracts of G. inflata, and G. uralensis were cytotoxic at 500 μg mL−1 with reduced cell viability between >100–500 μg mL−1 and >50–100 μg mL−1, respectively, whereas G. glabra spent extract did not show cytotoxicity up to 1000 μg mL−1, with reduced cell viability between >100–500 μg mL−1. Marker compounds glab, licoA, and glycy showed cytotoxicity with a distinct off–on effect at 50 μg mL−1, but cell viability showed a reduced trend at 25 μg mL−1 for glab and glycy, and 12.5 μg mL−1 for licoA.
4. Conclusions
In this comprehensive study, we have characterized the prenylated phenolics composition, antimicrobial activity, cytotoxicity, and cell viability of EtOAc spent extracts of G. glabra, G. inflata, and G. uralensis, together with their marker compounds glab, licoA, and glycy. G. uralensis and G. inflata spent extracts were more antibacterially potent than G. glabra, possible due to their higher (approximately 2× higher) content of prenylated phenolics. G. glabra spent extract showed lower cytotoxicity than those of G. inflata and G. uralensis, possibly explained by its lower prenylated phenolics content and higher relative content in double prenylated phenolics. Linking antibacterial activity of the liquorice spent extracts with cell viability showed that MICs against S. aureus coincide with concentrations where cell viability was not reduced. For the other tested Gram positive and Gram negative bacteria, and yeasts, MICs concurred at concentrations where cell viability was reduced. To conclude, in this study we show that liquorice spent extracts rich in prenylated phenolics are potent antimicrobials against Gram positive bacteria, however given their cytotoxicity profiles, further in-depth investigating should reveal their future applicability.
Author contributions
Sarah van Dinteren: Conceptualization, methodology, investigation, visualization, writing – original draft, preparation. Carla Araya-Cloutier: Conceptualization, methodology, writing – review & editing, supervision. Renger Witkamp: Writing – review & editing. Bo van Ieperen: Investigation. Jocelijn Meijerink: Conceptualization, methodology, writing – review & editing, supervision. Jean-Paul Vincken: Writing – review & editing, supervision.
Conflicts of interest
The authors declare that they have no known competing financial interests or personal relationships that could have appeared to influence the work reported in this paper.
Acknowledgements
This work was supported by Topconsortium voor Kennis en Innovatie (TKI, grant number TKI-AF-18124). Private partners did not have any influence on the scientific content.
References
- G. R. Fenwick, J. Lutomski and C. Nieman, Liquorice, Glycyrrhiza glabra L.—Composition, uses and analysis, Food Chem., 1990, 38, 119–143 CrossRef
.
- Z. Shang, C. Liu, X. Qiao and M. Ye, Chemical analysis of the Chinese herbal medicine licorice (Gan-Cao): An update review, J. Ethnopharmacol., 2022, 299, 115686 CrossRef PubMed
.
- EMA, Journal, 2012.
- Licorice Extracts Market, Global Industry Analysis, Size, Share, Growth, Trends and Forecast 2019–2029, https://www.transparencymarketresearch.com/licorice-extracts-market.html, (accessed 03 October 2022).
- Y. X. Han, X. R. Pang, X. M. Zhang, R. L. Han and Z. S. Liang, Resource sustainability and challenges: Status and competitiveness of international trade in licorice extracts under the Belt and Road Initiative, Glob. Ecol. Conserv., 2022, 34, e02014 CrossRef
.
-
T. K. Lim, in Edible Medicinal and Non-Medicinal Plants, Springer, 2016, pp. 354–457 Search PubMed
.
- C. Araya-Cloutier, H. M. W. den Besten, S. Aisyah, H. Gruppen and J.-P. Vincken, The position of prenylation of isoflavonoids and stilbenoids from legumes (Fabaceae) modulates the antimicrobial activity against Gram positive pathogens, Food Chem., 2017, 226, 193–201 CrossRef PubMed
.
- B. Botta, P. Menendez, G. Zappia, R. A. de Lima, R. Torge and G. Delle Monachea, Prenylated Isoflavonoids: Botanical Distribution, Structures, Biological Activities and Biotechnological Studies. An Update (1995-2006), Curr. Med. Chem., 2009, 16, 3414–3468 CrossRef CAS PubMed
.
- C. L. Ventola, The antibiotic resistance crisis: part 1: causes and threats, Pharm. Ther., 2015, 40, 277–283 Search PubMed
.
- M. Carocho, M. F. Barreiro, P. Morales and I. C. F. R. Ferreira, Adding Molecules to Food, Pros and Cons: A Review on Synthetic and Natural Food Additives, Compr. Rev. Food Sci. Food Saf., 2014, 13, 377–399 CrossRef PubMed
.
- P. S. Negi, Plant extracts for the control of bacterial growth: Efficacy, stability and safety issues for food application, Int. J. Food Microbiol., 2012, 156, 7–17 CrossRef
.
- N. C. Veitch, Isoflavonoids of the Leguminosae, Nat. Prod. Rep., 2013, 30, 988–1027 RSC
.
- S. van Dinteren, C. Araya-Cloutier, W. J. C. de Bruijn and J.-P. Vincken, A targeted prenylation analysis by a combination of IT-MS and HR-MS: identification of prenyl number, configuration, and position in different subclasses of (iso) flavonoids, Anal. Chim. Acta, 2021, 338874 CrossRef CAS PubMed
.
- W. Song, X. Qiao, K. Chen, Y. Wang, S. Ji, J. Feng, K. Li, Y. Lin and M. Ye, Biosynthesis-Based Quantitative Analysis of 151 Secondary Metabolites of Licorice To Differentiate Medicinal Glycyrrhiza, Species and Their Hybrids, Anal. Chem., 2017, 89, 3146–3153 CrossRef
.
- W. C. Liao, Y. H. Lin, T. M. Chang and W. Y. Huang, Identification of two licorice species, Glycyrrhiza uralensis and Glycyrrhiza glabra, based on separation and identification of their bioactive components, Food Chem., 2012, 132, 2188–2193 CrossRef
.
- X. Qiao, W. Song, S. Ji, Q. Wang, D. A. Guo and M. Ye, Separation and characterization of phenolic compounds and triterpenoid saponins in licorice (Glycyrrhiza uralensis) using mobile phase-dependent reversed-phase x reversed-phase comprehensive two-dimensional liquid chromatography coupled with mass spectrometry, J. Chromatogr. A, 2015, 1402, 36–45 CrossRef PubMed
.
- T. T. Xu, M. Yang, Y. F. Li, X. Chen, Q. R. Wang, W. P. Deng, X. Y. Pang, K. T. Yu, B. H. Jiang, S. H. Guan and D. A. Guo, An integrated exact mass spectrometric strategy for comprehensive and rapid characterization of phenolic compounds in licorice, Rapid Commun. Mass Spectrom., 2013, 27, 2297–2309 CrossRef
.
- T. Hatano, Y. Shintani, Y. Aga, S. Shiota, T. Tsuchiya and T. Yoshida, Phenolic constituents of licorice. VIII. Structures of glicophenone and glicoisoflavanone, and effects of licorice phenolics on methicillin-resistant Staphylococcus aureus, Chem. Pharm. Bull., 2000, 48, 1286–1292 CrossRef CAS
.
- S. Gibbons, Anti-staphylococcal plant natural products, Nat. Prod. Rep., 2004, 21, 263–277 RSC
.
- C. Araya-Cloutier, J.-P. Vincken, R. van Ederen, H. M. W. den Besten and H. Gruppen, Rapid membrane permeabilization of Listeria monocytogenes and Escherichia coli, induced by antibacterial prenylated phenolic compounds from legumes, Food Chem., 2018, 240, 147–155 CrossRef CAS
.
- S. G. Parkar, D. E. Stevenson and M. A. Skinner, The potential influence of fruit polyphenols on colonic microflora and human gut health, Int. J. Food Microbiol., 2008, 124, 295–298 CrossRef CAS
.
- J. Hummelova, J. Rondevaldova, A. Balastikova, O. Lapcik and L. Kokoska, The relationship between structure and in vitro antibacterial activity of selected isoflavones and their metabolites with special focus on antistaphylococcal effect of demethyltexasin, Lett. Appl. Microbiol., 2015, 60, 242–247 CrossRef CAS PubMed
.
- C. Araya-Cloutier, J.-P. Vincken, M. G. M. van de Schans, J. Hageman, G. Schaftenaar, H. M. W. den Bestens and H. Gruppen, QSAR-based molecular signatures of prenylated (iso)flavonoids underlying antimicrobial potency against and membrane-disruption in Gram positive and Gram negative bacteria, Sci. Rep., 2018, 8, 1–14 CAS
.
- A. Bartmańska, T. Tronina, J. Poploński, M. Milczarek, B. Filip-Psurska and J. Wietrzyk, Highly Cancer Selective Antiproliferative Activity of Natural Prenylated Flavonoids, Molecules, 2018, 23, 2922 CrossRef
.
- C. Dorn, T. S. Weiss, J. Heilmann and C. Hellerbrand, Xanthohumol, a prenylated chalcone derived from hops, inhibits proliferation, migration and interleukin-8 expression of hepatocellular carcinoma cells, Int. J. Oncol., 2010, 36, 435–441 Search PubMed
.
- C. H. Jiang, T. L. Sun, D. X. Xiang, S. S. Wei and W. Q. Li, Anticancer Activity and Mechanism of Xanthohumol: A Prenylated Flavonoid From Hops (Humulus lupulus, L.), Front. Pharmacol., 2018, 9, 530 CrossRef PubMed
.
- L. Molčanová, D. Janošíková, S. Dall'Acqua and K. Šmejkal,
C-prenylated flavonoids with potential cytotoxic activity against solid tumor cell lines, Phytochem. Rev., 2019, 18, 1051–1100 CrossRef
.
- Z. B. Yu, S. H. Dong, J. W. Zhang, D. Y. Li, Y. Liu, H. Li and S. S. Du, Analysis of international competitiveness of the China's licorice industry from the perspective of global trade, J. Ethnopharmacol., 2022, 298, 115613 CrossRef PubMed
.
- A. Cencič and T. Langerholc, Functional cell models of the gut and their applications in food microbiology - A review, Int. J. Food Microbiol., 2010, 141, S4–S14 CrossRef PubMed
.
- K. Ferenczi-Fodor, Z. Vegh, A. Nagy-Turak, B. Renger and M. Zeller, Validation and quality assurance of planar chromatographic procedures in pharmaceutical analysis, J. AOAC Int., 2001, 84, 1265–1276 CrossRef CAS PubMed
.
- D. C. Aryani, H. M. W. den Besten, W. C. Hazeleger and M. H. Zwietering, Quantifying strain variability in modeling growth of Listeria monocytogenes, Int. J. Food Microbiol., 2015, 208, 19–29 CrossRef CAS
.
- M. C. Sulavik, C. Houseweart, C. Cramer, N. Jiwani, N. Murgolo, J. Greene, B. DiDomenico, K. J. Shaw, G. H. Miller, R. Hare and G. Shimer, Antibiotic susceptibility profiles of Escherichia coli strains lacking multidrug efflux pump genes, Antimicrob. Agents Chemother., 2001, 45, 1126–1136 CrossRef CAS
.
- H. I. Zgurskaya, C. A. Lopez and S. Gnanakaran, Permeability Barrier of Gram-negative Cell Envelopes and Approaches To Bypass It, ACS Infect. Dis., 2015, 1, 512–522 CrossRef CAS PubMed
.
- W. V. Kern, P. Steinke, A. Schumacher, S. Schuster, H. von Baum and J. A. Bohnert, Effect of 1-(1-naphthylmethyl)-piperazine, a novel putative efflux pump inhibitor, on antimicrobial drug susceptibility in clinical isolates of Escherichia coli, J. Antimicrob. Chemother., 2006, 57, 339–343 CrossRef CAS PubMed
.
- A. C. Abreu, A. Coqueiro, A. R. Sultan, N. Lemmens, H. K. Kim, R. Verpoorte, W. J. B. Van Wamel, M. Simões and Y. H. Choi, Looking to nature for a new concept in antimicrobial treatments: isoflavonoids from Cytisus striatus as antibiotic adjuvants against MRSA, Sci. Rep., 2017, 7, 1–16 CrossRef CAS
.
- S. P. Denyer and J. Y. Maillard, Cellular impermeability and uptake of biocides and antibiotics in Gram-negative bacteria, J. Appl. Microbiol., 2002, 92, 35s–45s CrossRef
.
- S. Kalli, C. Araya-Cloutier, J. Hageman and J.-P. Vincken, Insights into the molecular properties underlying antibacterial activity of prenylated (iso)flavonoids against MRSA, Sci. Rep., 2021, 11, 1–14 CrossRef PubMed
.
- M. Song, Y. Liu, T. Li, X. Liu, Z. Hao, S. Ding, P. Panichayupakaranant, K. Zhu and J. Shen, Plant Natural Flavonoids Against Multidrug Resistant Pathogens, Adv. Sci., 2021, 8, e2100749 CrossRef PubMed
.
-
V. Lorian, Antibiotics in laboratory medicine, Lippincott Williams & Wilkins, 2005 Search PubMed
.
- J. A. Lemos, S. R. Palmer, L. Zeng, Z. T. Wen, J. K. Kajfasz, I. A. Freires, J. Abranches and L. J. Brady, The Biology of Streptococcus mutans, Microbiol. Spectrum, 2019, 7, 7–1 Search PubMed
.
- S. J. Ahn, S. N. Park, Y. J. Lee, E. J. Cho, Y. K. Lim, X. M. Li, M. H. Choi, Y. W. Seo and J. K. Kook,
In vitro Antimicrobial Activities of 1-Methoxyficifolinol, Licorisoflavan A, and 6,8-Diprenylgenistein against Streptococcus mutans, Caries Res., 2015, 49, 78–89 CrossRef PubMed
.
- Y. W. Chen, S. R. Ye, C. Ting and Y. H. Yu, Antibacterial activity of propolins from Taiwanese green propolis, J. Food Drug Anal., 2018, 26, 761–768 CrossRef CAS PubMed
.
- R. Gaur, V. K. Gupta, P. Singh, A. Pal, M. P. Darokar and R. S. Bhakuni, Drug Resistance Reversal Potential of Isoliquiritigenin and Liquiritigenin Isolated from Glycyrrhiza glabra, Against Methicillin-Resistant Staphylococcus aureus (MRSA), Phytother. Res., 2016, 30, 1708–1715 CrossRef CAS PubMed
.
- L. R. Huang, X. J. Hao, Q. J. Li, D. P. Wang, J. X. Zhang, H. Luo and X. S. Yang, 18beta-Glycyrrhetinic Acid Derivatives Possessing a Trihydroxylated A Ring Are Potent Gram-Positive Antibacterial Agents, J. Nat. Prod., 2016, 79, 721–731 CrossRef CAS
.
- S. Kalli, C. Araya-Cloutier, J. Chapman, J. W. Sanders and J. P. Vincken, Prenylated (iso)flavonoids as antifungal agents against the food spoiler Zygosaccharomyces parabailii, Food Control, 2022, 132, 108434 CrossRef CAS
.
- K. Fujita and I. Kubo, Naturally occurring antifungal agents against Zygosaccharomyces bailii and their synergism, J. Agric. Food Chem., 2005, 53, 5187–5191 CrossRef CAS
.
- K. Fujita, T. Fujita and I. Kubo, Antifungal activity of alkanols against Zygosaccharomyces bailii and their effects on fungal plasma membrane, Phytother. Res., 2008, 22, 1349–1355 CrossRef CAS
.
- N. T. Dat, P. T. Binh, T. P. Quynh le, C. Van Minh, H. T. Huong and J. J. Lee, Cytotoxic prenylated flavonoids from Morus alba, Fitoterapia, 2010, 81, 1224–1227 CrossRef PubMed
.
- H. Poerwono, S. Sasaki, Y. Hattori and K. Higashiyama, Efficient microwave-assisted prenylation of pinostrobin and biological evaluation of its derivatives as antitumor agents, Bioorg. Med. Chem. Lett., 2010, 20, 2086–2089 CrossRef CAS PubMed
.
- M. P. Neves, H. Cidade, M. Pinto, A. M. S. Silva, L. Gales, A. M. Damas, R. T. Lima, M. H. Vasconcelos and M. D. J. Nascimento, Prenylated derivatives of
baicalein and 3,7-dihydroxyflavone: Synthesis and study of their effects on tumor cell lines growth, cell cycle and apoptosis, Eur. J. Med. Chem., 2011, 46, 2562–2574 CrossRef CAS
.
- J. B. Daskiewicz, F. Depeint, L. Viornery, C. Bayet, G. Comte-Sarrazin, G. Comte, J. M. Gee, I. T. Johnson, K. Ndjoko, K. Hostettmann and D. Barron, Effects of flavonoids on cell proliferation and caspase activation in a human colonic cell line HT29: An SAR study, J. Med. Chem., 2005, 48, 2790–2804 CrossRef CAS PubMed
.
- D. Çevik, Ş. B. Yilmazgöz, Y. Kan, E. A. Güzelcan, I. Durmaz, R. Çetin-Atalay and H. Kirmizibekmez, Bioactivity-guided isolation of cytotoxic secondary metabolites from the roots of Glycyrrhiza glabra and elucidation of their mechanisms of action, Ind. Crops Prod., 2018, 124, 389–396 CrossRef
.
- E. H. Jo, H. D. Hong, N. C. Ahn, J. W. Jung, S. R. Yang, J. S. Park, S. H. Kim, Y. S. Lee and K. S. Kang, Modulations of the Bcl-2/Bax family were involved in the chemopreventive effects of licorice root (Glycyrrhiza uralensis fisch) in MCF-7 human breast cancer cell, J. Agric. Food Chem., 2004, 52, 1715–1719 CrossRef CAS
.
- K. Šmejkal, Cytotoxic potential of C-prenylated flavonoids, Phytochem. Rev., 2014, 13, 245–275 CrossRef
.
- J. Cao, X. Chen, J. Liang, X. Q. Yu, A. L. Xu, E. Chan, D. Wei, M. Huang, J. Y. Wen, X. Y. Yu, X. T. Li, F. S. Sheu and S. F. Zhou, Role of P-glycoprotein in the intestinal absorption of glabridin, an active flavonoid from the root of Glycyrrhiza glabra, Drug Metab. Dispos., 2007, 35, 539–553 CrossRef
.
- M. J. Hsieh, C. W. Lin, S. F. Yang, M. K. Chen and H. L. Chiou, Glabridin inhibits migration and invasion by transcriptional inhibition of matrix metalloproteinase 9 through modulation of NF-kappaB and AP-1 activity in human liver cancer cells, Br. J. Pharmacol., 2014, 171, 3037–3050 CrossRef PubMed
.
- M. M. Rafi, R. T. Rosen, A. Vassil, C. T. Ho, H. Y. Zhang, G. Ghai, G. Lambert and R. S. DiPaola, Modulation of bcl-2 and cytotoxicity by licochalcone-A, a novel estrogenic flavonoid, Anticancer Res., 2000, 20, 2653–2658 Search PubMed
.
- L. F. B. Bortolotto, F. R. Barbosa, G. Silva, T. A. Bitencourt, R. O. Beleboni, S. J. Baek, M. Marins and A. L. Fachin, Cytotoxicity of trans-chalcone and licochalcone A against breast cancer cells is due to apoptosis induction and cell cycle arrest, Biomed. Pharmacother., 2017, 85, 425–433 CrossRef PubMed
.
- Y. Fu, T. C. Hsieh, J. Q. Guo, J. Kunicki, M. Y. W. T. Lee, Z. Darzynkiewicz and J. M. Wu, Licochalcone-A, a novel flavonoid isolated from licorice root (Glycyrrhiza glabra), causes G2 and late-G1 arrests in androgen-independent PC-3 prostate cancer cells, Biochem. Biophys. Res. Commun., 2004, 322, 263–270 CrossRef PubMed
.
- Q. Wang, Y. Kuang, W. Song, Y. Qian, X. Qiao, D. A. Guo and M. Ye, Permeability through the Caco-2 cell monolayer of 42 bioactive compounds in the TCM formula Gegen-Qinlian Decoction by liquid chromatography tandem mass spectrometry analysis, J. Pharm. Biomed. Anal., 2017, 146, 206–213 CrossRef PubMed
.
- X. H. Song, S. T. Yin, E. X. Zhang, L. H. Fan, M. Ye, Y. Zhang and H. B. Hu, Glycycoumarin exerts anti-liver cancer activity by directly targeting T-LAK cell-originated protein kinase, Oncotarget, 2016, 7, 65732–65743 CrossRef PubMed
.
- X. X. Wang, G. Y. Liu, Y. F. Yang, X. W. Wu, W. Xu and X. W. Yang, Intestinal Absorption of Triterpenoids and Flavonoids from Glycyrrhizae radix, et rhizoma in the Human Caco-2 Monolayer Cell Model, Molecules, 2017, 22, 1627 CrossRef PubMed
.
- G. B. Gonzales, G. Smagghe, C. Grootaert, M. Zotti, K. Raes and J. Van Camp, Flavonoid interactions during digestion, absorption, distribution and metabolism: a sequential structure-activity/property relationship-based approach in the study of bioavailability and bioactivity, Drug Metab. Rev., 2015, 47, 175–190 CrossRef PubMed
.
|
This journal is © The Royal Society of Chemistry 2022 |
Click here to see how this site uses Cookies. View our privacy policy here.