DOI:
10.1039/D1GC01799C
(Critical Review)
Green Chem., 2022,
24, 62-101
Chitosan-based inks for 3D printing and bioprinting
Received
21st May 2021
, Accepted 9th August 2021
First published on 8th December 2021
Abstract
The advent of 3D-printing/additive manufacturing in biomedical engineering field has introduced great potential for the preparation of 3D structures that can mimic native tissues. This technology has accelerated the progress in numerous areas of regenerative medicine, especially led to a big wave of biomimetic functional scaffold developments for tissue engineering demands. In recent years, the introduction of smart bio-inks has created growing efforts to facilitate the preparation of complex and homogeneous living-cell-containing 3D constructs. In the past decade, a considerable body of literature has been created on identifying an ideal bioinspired-ink with excellent printability, cell viability, bioactivity, and mechanical properties. This state-of-the-art review article briefly outlines 3D-printing/bioprinting techniques applied for chitosan-based bio-inks, their resources, crosslinking methods, characteristics, reasons for their superiority over other bio-inks, and challenges of commercialization; this is followed by a comprehensive description of the full potential and the key indicators of success in terms of 3D bio-printing of such bio-inks as platforms for tissue regeneration, advanced biosensors, drug delivery, and wastewater treatment. Next, the restrictions and challenges of chitosan bio-inks are highlighted. In this work, we also discussed about developing a coherent research strategy based on combination of microfluidics-based lab-on-a-chip (organ-on-a-chip) platforms with 3D-bioprinting which enables designing of self-healing scaffolds. And finally, the potential of smart inks based on chitosan for 4D bioprinting of more detailed and practical engineered tissues and artificial organs is reviewed.
1. Introduction
Bioprinters, unlike 3D-printers, are suited to printing gel-based materials and biological materials (in particular bio-inks) and, additionally, they can perform noncontact droplet printing.1–3 In other words, 3D-printing of either biological inks (bio-inks) or cell-laden inks is considered as a new process called 3D-bioprinting. During the past few years, bioprinting has become a new approach to manufacture various kinds of 3D framework with different bioprintable inks for tissue engineering and drug delivery applications.4 The proposed scaffolds’ features are similar to living body parts.5 There is a multitude of reasons why bioprinting has been attracting attention of researchers for biomedical applications (see Fig. 1). The advantages of using 3D-bioprinting could be listed as: (a) controlling the system and the process, which allows for biomolecules and cells to be directly encapsulated in the bioprinted network, (b) post-modification of 3D-bioprinted constructs, which is frequently found to be problematic for scaffolds using conventional techniques, and (c) bioprinting as one of the appealing processes to open a new window into regenerative medicine through enabling a high resolution of organ printing.6,7
 |
| Fig. 1 Yearly evolution of papers on 3D-printing and 3D-bioprinting based on the number of publications. These data were extracted on July, 12 2021 from the Scopus database.5,8 | |
According to Fig. 1, along with development of 3D-printing, the application of 3D bioprinting in biomedical engineering has sharply developed. Surpassing the number of research studies based on 3D-bioprinting from five hundred by 2020 (according to the Scopus database) gives us a great hope for possible successful achievements in the near future. Materials used in 3D-bioprinting should have printing potential without deterioration in their mechanical stability and shape integrity, as well as supporting cell viability and differentiation.9 To achieve a construct with improved physical stability, chemical, physical, or enzymatic crosslinking of the bio-ink is required. Besides, bio-ink formulations should support cell adhesion and proliferation combined with growth factors and cell media to enable cells to flourish in the bioprinted constructs while mimicking the target tissue architecture. From a bio-ink perspective, finding natural or synthetic bioprintable materials with essential features such as suitable mechanical stability to withstand physical stress and improved integrity of their structure and network within in vivo microenvironments is the major challenge. Recent advances in 3D-bioprinting for biomedical engineering can respond to the emerging needs through emergence of a wide range of hydrogels based on natural or synthetic precursors and more accurate 3D-bioprinting methodologies (Fig. 2).10
 |
| Fig. 2 Timeline of the most significant steps forward in the development of 3D-bioprinting.11–14 | |
Due to the countless disadvantages of using synthetic polymers, such as poor biocompatibility and cellular adhesion as well as mechanical instability along with producing noxious by-products during the degradation process, indisputably natural polymers are considered as the better choice for 3D-printing demands.15–17 Given the information proposed in Table 1, there are multiple concerns regarding the use of biopolymers as inks. The most significant groups of natural polymers are marine polysaccharides (e.g., alginate, chitosan, agarose, etc.). Disadvantages like the immunogenicity of fibrin, shape inconsistency of collagen, high viscosity of agarose, rheology optimization requirements of silk fibroin, and low cell attachment and low protein absorption of alginate push us to make use of alternative biopolymers. Chitosan, a polycationic biocompatible natural polymer, possesses some unique merits for use in bioprinting. Chitosan-containing solutions remain stable under physiological conditions and show appropriate values of viscosity, applicable for bioprinting applications.18–23 Moreover, chitosan supports proper cell proliferation and differentiation. Cells cultured on chitosan scaffolds show high viability.24,25 Thus, chitosan resins and hydrogels meet these requirements; furthermore, chitosan hydrogels can be tuned to be good mimics of the native extracellular matrix (ECM) of tissues.25 However, this natural polymer has shown some drawbacks in tissue engineering, including a slow gelation rate and weak mechanical strength.26,27 Here, it is vital to note that the physical stability and mechanical strength of a bio-ink require it to be a liquid or semi-solid material that can be chemically or physically crosslinked. Thus, chitosan-based biomaterials can be modified by a variety of approaches like coupling with methacrylic anhydride (methacrylation of backbone), to improve the possibility of a stronger crosslinking. Indeed, the crosslinking of chitosan hydrogels facilitates the process of overcoming its inherent minor disadvantages and makes it a biomaterial of choice for bioprinting due to its attractive portfolio of properties mentioned above. As a consequence, it is rational to use chitosan and chitosan derivatives for applications in tissue engineering to replace or fix bone, cartilage, and skin.28
Table 1 Currently used 3D-bioprinting bio-inks for engineered body tissues8,19
Bio-ink |
Chemical structure |
Printing process |
Application |
Bio-ink properties |
|
Ref. |
Chitosan (natural polymer) |
|
Extrusion-based bioprinting (layer-by-layer deposition), laser-based bioprinting (e.g. stereolithography) |
Bone tissue, vascular tissue, cartilage tissue (e.g., human ear), skin tissue |
Stability under physiological conditions, appropriate range of solution viscosity values, cell viability, cell proliferation and differentiation, applicability, printability |
|
29–34
|
GelMA (functionalized natural polymer) |
|
Extrusion-based bioprinting (layer-by-layer (LBL)) deposition, laser-based bioprinting droplet-based bioprinting |
Articular cartilage, bone regeneration, cardiac myocytes and fibroblasts |
Good cell adhesion, low mechanical properties, low viscosity at low shear rates |
|
35–37
|
Alginate (natural polymer) |
|
Extrusion-based bioprinting (layer-by-layer (LBL)) deposition, laser-based bioprinting droplet-based bioprinting |
Bone, vascular tissue, cartilage |
Fast crosslinking ability, non-toxic, non-immunogenic, quick rate of degradation, various cellular responses due to different resources, elevated hydrophilic nature which causes low cell attachment and absorbability of protein |
|
10 and 38–41 |
Gelatin (natural polymer) |
|
Extrusion-based bioprinting |
Human nasal inferior turbinate tissue, cartilage, vascular tissue |
Possessing inherent signaling molecules for cell adhesion, thermoresponsive, good cellular proliferation, low immunogenicity, mechanically weak at physiological temperatures |
|
42–44
|
Silk fibroin (natural polymer) |
|
Extrusion-based bioprinting (e.g., inkjet) |
Musculoskeletal tissue, human bone marrow mesenchymal stem cells, human nasal inferior turbinate tissue |
Ease of structural modification, controlled degradation, crosslinking, and co-polymerization required to optimize the rheology of the bio-ink for more optimal printability |
|
45 and 46 |
Pluronic F-127 (synthetic polymer) |
|
Extrusion-based bioprinting |
Fibroblasts, vascularized tissue (vessel formation), neural and glial tissues |
Excellent degradation, good printability, and bioprintability, nonimmunogenic, heat needed for printability, low mechanical properties, fast gelation at 37 °C to avoid cell sedimentation, small shear forces during the printing process to preserve cell viability, sufficient concentration of polymer to allow quick supply of nutrients and oxygen to encapsulated cells and removal of waste |
|
47–49
|
Agarose (natural polymer) |
|
Extrusion-based bioprinting |
Vascular tissue |
Low cell adhesion and spreading, cell viability, non-toxic, non-degradable, gel at low temperatures, high viscosity which makes it not suitable for inkjet printing |
|
50 and 51 |
Fibrin (natural polymer) |
|
Droplet-based bioprinting |
Neural stem cells, adipose tissue, bone, cardiac tissue, ocular tissue, cartilage, skin, liver, tendons, nervous tissue, and ligaments |
Possesses inherent signaling molecules for cell adhesion, low mechanical properties, infectious transmission, non-shear-thinning behavior |
|
10, 52 and 53 |
Collagen type I (natural polymer) |
|
Extrusion-based bioprinting, droplet-based bioprinting |
Vascular tissue, skin tissue |
Appropriate cell adhesion, minimal immunological reactions, slow gelation rate for bioprinting, immense structural changes by volume shrinkage, poor mechanical properties |
|
10
|
Hyaluronan gel (natural polymer) |
|
Extrusion-based bioprinting, laser-based bioprinting |
Bone tissue, cartilage, vascular tissue |
Biocompatibility, ability to form flexible hydrogels, poor mechanical properties, gel often contains impurities, mechanically weak without modification |
|
54 and 55 |
PEG (synthetic polymer) |
|
Extrusion-based bioprinting, droplet-based bioprinting |
Articular cartilage |
Nonimmunogenic, high cell viability, considerable cell proliferation rate, biocompatibility, poor mechanical stability without modification, low cell adhesion |
|
56 and 57 |
In this review, we highlighted the key reasons for selecting chitosan-based inks for 3D-printing and bioprinting; moreover, a wide array of applications of these bioprinted-based scaffolds in various branches of biomedical engineering are described to understand chitosan's full potential. Besides, the remained challanges in the way of using chitosan-based bio-inks were fully disscused. Indeed, quantifying the characteristics of chitosan-based bio-inks and comparing them with the ideal bio-ink is such an important issue and results in an understanding of the best way to exploit their full strengths and to overcome the existing constraints through modification processes. In this study, we have tried to cover the most recent papers, which have expanded the description and important analysis of documented methods of functionalization, concentrating on their results and contributions towards 3D-printable bio-inks based on chitosan. At the end, we also explained the future directions of 3D-bioprinting based on chitosan.
2. 3D-Bioprinting and the role of chitosan
3D-bioprinting represents a fast-growing field of science with a strong commitment to mimicking applicable artificial tissues and organs. This technology provides the means of designing and generating structures to replace damaged tissues and impaired organs with patients’ own cells. The main ingredients of bioprinting processes are bioprinters, 3D-bioprinting modalities, and bio-inks. Bio-inks usually are 3D-printable bio-based material solutions, applied in living cell bioprinting, which are classified into two big groups of (a) scaffold-based bio-inks (including microcarriers, hydrogels, cell aggregates, and decellularized matrix) and (b) scaffold-free bio-inks (tissue strands, tissue spheroids, cell pellets).58 To facilitate the synthesis of efficient and applicable bio-inks, interdisciplinary collaborations of scientists working in different disciplines such as chemistry, materials science, mechanical engineering, biology, and biomedical engineering are advantageous. Indeed, an applicable bioprinting modality needs to be adapted to the specific requirements of the intended printing process and to be suited to appropriate printing of a wide range of biomaterials. In general, it is considered to be more compatible with novel emerging bio-inks, in particular those with characteristics that build current constructs (see Fig. 3).59
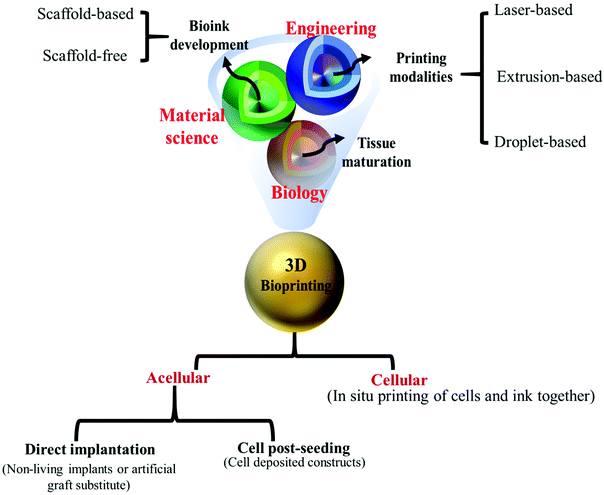 |
| Fig. 3 An overview of different aspects of 3D-bioprinting.68 Bioprinting as a multi-disciplinary science required a collaborative work between chemists, engineers, and biologists to study tissue maturation and design new, suitable scaffold-free/scaffold-based bio-inks to apply them through three primary 3D-bioprinting methods (extrusion-, droplet-, and laser-based methods) in order to prepare applicable, exquisite cellular/acellular 3D-bioprinted constructs for biomedical uses. | |
According to the literature, bio-inks could be printed through three main bioprinting methods:
1. Extrusion-based (powered by pneumatic, mechanical, or solenoid drivers),
2. Laser-based (stereolithography, mask-image projection, laser-induced forward transfer, beam scanning, laser-guided direct writing, selective laser sintering),
3. Droplet-based (inkjet-based, multi-jet modeling, electrohydrodynamic jetting, laser assisted-droplets, and pneumatic pressure assisted) bioprinting.60–62
Extrusion-based bioprinting methods (EBB) are most commonly used in the topic of chitosan-based bio-inks for 3D-scaffold construction (Table 2). Laser-based bioprinting techniques (LBB) have been rarely used in this area, and on the other hand, the droplet-based bioprinting method (DBB) needs to be investigated.
Table 2 Comparison of various kinds of 3D-printing/bioprinting approaches for preparation of engineered tissue scaffolds3,12,60,61
Additive manufacturing categories (3D-printing) |
3D bioprinting |
Features |
Ref. |
Printing modality |
Material |
Description |
Chitosan 3D-printability |
Bioprinting modality |
Material |
Chitosan 3D bioprintability |
Binder jetting |
Metals, ceramics, polymers, & powders (plastics & sands) |
Powered layers are joined together by adhesive liquid binder |
Applicable |
Laser-based bioprinting |
Laser-induced forward transfer |
Cells in media |
Applicable |
Low mechanical properties, high preparation time, high precision, weak structural integrity, very good resolution, time-consuming process, single-cell level printability, low scalability, high printing cost, high cell densities printable, process often requires high-intensity UV light and long post-processing (potentially problematic for cells) |
10, 69 and 70 |
Stereolithography-based bioprinting |
Hydrogels |
Applicable |
Smooth surface finish, fast printing, high spatial resolution, low cost, good quality of vertical printing, fair cell density (<108 cells per mL), high cell viability, layer-by-layer printing process |
10, 69 and 70 |
Powder bed fusion (DMLS, EBM, SLS, SLM) |
Powders (plastics & metals) |
Thermal energy selectively fuses regions of a powder bed |
N/A |
Droplet-based bioprinting |
Granule-based medium-assisted |
Cells in media |
|
To increase printability of bio-inks with weak printability and a poor crosslinking density |
71
|
Material jetting |
Polymers & gels |
Droplets of build material are selectively deposited |
N/A |
Inkjet-based |
Liquids and hydrogels |
Applicable |
Low preparation time, low mechanical properties with poor structure integrity, high throughput (scalable), high cell viability, moderate accuracy, affordable moderate precision, low printing cost, low cell densities can be printed, inferior droplet size control, an important condition for low viscous bio-inks |
69 and 72 |
Sheet lamination (LOM, UC) |
Paper, plastic, wood, metals like aluminum, & composites |
Sheets of material are bonded to form an object |
N/A |
Material extrusion (FDM, bioplotting) |
Polymers & gels |
Inks extrude through nuzzle tips |
Applicable |
Multi-jet modeling |
Hard and soft plastics, elastomers |
Applicable |
High resolution, high accuracy, suitable for small cell-culturing approach (microfluidic channels and chip), fast printing, ease of multi-material preparation at the same time, high cost, current resolution of microchannels printing |
73
|
Directed energy deposition (LENS) |
Metals |
Focused thermal energy is utilized to produce a melt pool powders on a base platform |
N/A |
Extrusion-based bioprinting |
Hydrogels & cell aggregates |
Applicable |
High mechanical properties, fair preparation time, very good structural integrity poor fidelity, multi-nozzle multi-material printability, cells undergo shear stress at the nozzle tip, fair printing cost, viscous bio-inks, and high cell densities could be applied, cell structure distortion and often inferior resolution, good quality of vertical |
10, 69, 70 and 72 |
2.1. Extrusion-based
Using extrusion-based deposition system, working with air pressure (pneumatic) or mechanical forces (pistons or screws), bio-inks can be extruded precisely in three dimensions to form the desired 3D patterns in bioprinting. In general, these bioprinters are capable of quickly producing scaffolds with a resolution of around 100 to 200 μm.63 Extrusion-based bioprinted architectures with deposited cells and matrix proteins within hydrogel networks are focused on distinctive design, porosity, biomechanics, and composition, in which every factor is separately monitored to create structures with high accuracy from the submicron to the few-millimeter level, depending on the application. Bioprinting based on extrusion is the most commonly used method for 3D-bioprinting of chitosan-based bio-inks (Table 2).
2.2. Droplet-based
Droplet-based bioprinting differs from extrusion-based methods by a discontinuous application of microdroplets in a high-throughput manner, driven by various energy sources. Inkjet-based bioprinting techniques are the most prominent subclass of droplet-based bioprinting and are very cost-efficient. Cell-laden bio-inks can be printed with a speed of 104 to 105 drops per second at a high resolution of approximately down to 50 μm. Although some papers suggested possible cell damage related to both temperature and mechanical stresses during the printing process, surprisingly, suitable cooling systems prevent cell apoptosis.64–66 Inkjet-based bioprinting with single- and multi-jet techniques is suitable for the preparation of both cellular and acellular 3D-bioprinted chitosan-based scaffolds, especially for printing prevascular structures.
2.3. Laser-based
Laser-based modalities, as non-contact methods, are usually referred to as techniques making use of laser pulses to produce a droplet that will be deposited on the print surface that can eventually achieve a single-cell resolution. Cells printed via this method, in comparison with the extrusion-based modality, experience less mechanical stress, leading to superior viability. However, their operating speed is relatively lower than that of extrusion-based modalities.63,67 On the other hand, laser-based bioprinting allows for printing hydrogels with a high viscosity – above the viscosity limit of droplet-based bioprinting processes. Although a lack of accurate information on the impact of laser pulses on living cells, along with expensive machinery, has limited the use of laser-based methods, applicability of this approach in the past, especially stereolithography (SLA) and laser-induced forward transfer (LIFT), has been reported for scaffold biofabrication based on high viscous chitosan-based bio-inks.31
3. Reasons for choosing chitosan
There are a range of natural and synthetic polymers suitable for 3D-printing and bioprinting. Some examples of printable biopolymers are gelatin,74 silk,75 collagen,76 alginate77 and their functionalized types (e.g., gelatin methacryloyl (GelMA)78 and nano-cellulose79). There are also some well-known synthetic polymers,80 such as polyester-based, Pluronic®-based, polyhydroxy acid-based, polyurethane-based and PEG-based, thermoplastics, and silicones, used as 3D-bioprinting inks.81–83 For applications in medicine such as drug/gene/DNA delivery,84,85 biosensors,86 tissue engineering,87 cancer therapy,88,89 and diagnosis,90,91 the distinctive structural features require a high amount of hydrophilicity and sufficient porosity (interconnected) of the biomaterials for better growth and migration of the cells.92 The chitosan-based printed bio-inks possess unique characteristics such as excellent cell/matrix interactions, mimicking the native tissue structure, providing a microenvironment for oxygen and nutrition exchanges, and favorable immune responses following implantation.93–95 The presence of chitosan in bio-ink compositions could impart high antimicrobial activities. The ability for electron penetration into the negative shell membrane of bacteria from the electrostatic interaction of protonated NH3+ in chitosan leads to bacterial death or restricts their growth.96,97 Moreover, chitosan-based printed platforms exhibited biomolecule adaption, stable biocompatibility, and biological activity even after various post-printing modifications.98,99
3.1. Cell viability, migration, and proliferation
Crucial factors of basic 3D-bioprinted structures are proliferation, matrix remodeling, and cell motility. As the cells are surrounded by a hydrogel, after the 3D-bioprinting process they have to be capable of proliferating into complicated anatomically related 3D cellular architectures. Wang et al.100 suggested new hydroxyethyl-chitosan/cellulose scaffolds with bubble-like porous media. The chemical and physical structure of bio-inks controls the deposition of the released proteins, including protein signals. Cells can release morphogens to migrate to the surrounding. Then, a 3D-bioprinted hydrogel matrix supplies mechanical and architectural signals that direct cells for alignment. The cell motility may be influenced by various micro-environmental situations, including the geometry of the construct and the hydrophilicity of the material. The bio-ink, therefore, has a significant effect on setting the destiny of the surrounding cells.101 With the aid of integrin molecules, the cells stick to extracellular matrix proteins like fibronectin. The organization of protein collections and special adhesions is created while integrins are connected to the ligand.102
Since gelatin includes the Arg–Gly–Asp-like sequence to enhance its biological activity, it was combined with chitosan by Huang et al.103 Chitosan in chitosan/gelatin constructs is capable of creating insoluble ionic collections that contain gelatin, which is negatively charged.103,104 This can have an impact on the integrin ligands. The organization of protein collections and cell/matrix adhesions happens while the integrins are connected to the ligands.105 Therefore, it has a notable effect on the tissue remodeling procedure.
Bio-inks should demonstrate a low viscous behavior in order to adequately maintain the shear stress levels under moderate extrusion pressures to balance good cell viability and thin printing (high resolution).106 For 3D-bioprinting, various variables such as the diameter of the nozzle, the temperature, and the viscosity of the bio-inks have a direct effect on the shear stresses experienced by the bio-ink and, thus, by the embedded cells. Hence, controlling and balancing between the parameters involved in bioprinting is essential, where high-viscosity bio-inks and narrow nozzles are used to enhance the ultimate printer resolution, which, however, is associated with high shear stresses that might lead to cell-membrane damage and, thus, cell apoptosis.107
Bioprinting based on 3D extrusion most crucially focuses on tailoring the bio-ink to preserve cell viability by preventing damage to the cell membrane from process-induced mechanical impairments such as excessive shear stresses. This is achieved by an extremely hydrated environment to prevent drying and a physiological environment after printing. In this context, multi-nozzle bioprinting has a poor performance due to the low cell viability caused by external forces during extrusion. Furthermore, while a high viscosity (caused by a higher molar mass or crosslinking) leads to a superior post-printing physical stability, it lowers the cell viability because of the higher shear stresses. Moreover, for bioprinting, methods based on photocuring, the exposure of cells to excessive amounts of light even in the visible light range, might negatively impact cell functions as well as cell viability. Thus, cell viability is a key indicator for judging the processing properties of the extruded bio-inks. Accordingly, it is necessary to optimize crosslinking and viscosity to produce a bio-ink that is compatible with the cells and supplies high-quality scaffold constructions.
Whether cells are used for test procedures such as examining novel scaffolds or to be placed in patients, viability experiments are essential to analyze. Typically, viability experiments are conducted via Trypan blue exclusion approaches, live/dead assays, and MTT assays. It is crucial to preserve a high degree of cell viability throughout and after the bioprinting process. Up to now, according to the literature, low cell viability in printed scaffolds is one of the essential challenges.108 To address this deficiency, scientists add biopolymers (e.g., chitosan) to the inks to enhance the viscosity and, thus, the printing resolution. Although the presence of such biopolymers could improve the printability of bio-inks, cell viability might be reduced, in particular for high polymer contents. For instance, Chavanne et al.109 applied an adapted 3D-bioprinting process to prepare cylinder-shaped hydroxyapatite–chitosan with 40 wt% lactic acid (LA) for bone regeneration applications, where the authors used assessed chitosan concentration and LA addition to improve the hydrophilicity, rheological properties, and gelation time. A scaffold with 20 wt% chitosan and 40 wt% LA had a superior printability, and furthermore a post-treatment of the scaffolds with 10 wt% lactic acid leads to collapsed pores and a smooth chitosan cover layer on the constructs.
Some methods are available to improve cell viability: for example, in the case of a loss of integrity after printing, a modification of the ECM after decellularization could lead to increased structural viability.28,110 After seeding, cells cause ECM degradation because of the generation of matrix metalloproteinases. The most crucial disadvantage of this approach is the required step of ECM extraction, which is a complicated and risky procedure, which leads to very high costs for this tissue engineering technique.28
Some works focus on introducing nanostructured cavities into bio-inks. Results indicate that permeability could be enhanced by the nanoporosity of the printed structure,111,112 which, however, includes poorly understood connections between nanostructure characteristics and their influence on cell functions. Kyle et al.113 attempted to understand the printability procedure by analyzing the effects of bio-ink features and printing parameters on cell viability. They understood that printability affects cell viability in three ways: (1) the printing temperature, (2) the concentration of bio-ink, and (3) the holding time. Therefore, bioprinting parameter optimization of polymeric-based hydrogels has a severe impact on the printability of cell-embedded bio-inks.113,114 Poorly chosen operational parameters during and after printing such as crosslinking, temperature, and pH alteration can cause severe damage to the cell membranes and biological molecules (e.g., proteins and growth factors). Furthermore, crucial factors of a basic 3D-bioprinted structure are proliferation, matrix remodeling, and cell motility. Considering that an ink surrounds the cells after bioprinting, they have to be capable of proliferating into complicated anatomically related 3D cellular architectures. Accordingly, the ink has an essential effect in determining the destiny of the surrounded cells. The bio-ink's chemical structure adjusts the deposition of released proteins, including signaling proteins. Once cells released morphogens, they can migrate to the surrounding. Then, the 3D-bioprinted hydrogel matrix supplies mechanical and architectural signals that direct the cells for alignment. The cells’ mobility may be influenced by various micro-environmental situations, including the geometry of the construct and the hydrophilicity of the material. These factors need to be optimized to create scaffolds with geometries and stiffnesses similar to human tissues or organs. Some essential parameters of bio-inks are applied in 3D-bioprinting are as follows:115
(1) The rheology of bio-ink, such as viscosity, plays a pivotal role in the printing process. For example, when the viscosity of bio-ink is increased more than what it should be, it acts as a gel and leads to cell death, nozzle clogging, and the ejection of self-supporting filamentous frameworks. The initial viscosity of bio-ink should help the uniform distribution of cells and facilitate their mixing process without a sharp reduction in cell viability.
(2) For extrusion-based printing, bio-inks should possess low viscosity to enable the facile flow of bio-ink through the bioprinter with a narrow-sized nozzle. Meanwhile, after printing, the bio-ink must have the ability to maintain the 3D structure without shrinkage or collapse.
(3) The gelation time of bio-inks should be optimized not only to help the self-supportive networks but also to prevent a nonuniform distribution of cells and nozzle plugging.
(4) The surface tension of bio-inks should be optimized to allow better detachment from the tip of the nozzle and gentle filament extrusion.
(5) The ability of hydrogels to contain water is crucial for cell viability and readily transportation of nutrients, oxygen, and waste exchange.
(6) The extrudate/filament diameter is related to the rheology and flow rate of the bio-ink, the nozzle diameter, and the extrusion pressure.
3.2. Biomimicry
Cell modeling is possible by the printing of cell-laden bio-inks; nevertheless, following extracellular generation, digestion, and the deterioration of 3D-printed scaffolds and growth of encapsulated cells are all problems that need thoughtful consideration. Commonly applied cell-laden bio-inks have various innate drawbacks, including limited interactions of cells, migration, partial growth, and settlement of immobilized cells within the bio-ink network for better recapitulation of native tissue.116 The possibility of such problems emerging increases upon increase of bio-ink concentration and viscosity. The both homo- and hetero-cells-laden chitosan-based bio-inks exhibit excellent biomimetic properties due to an inability to immobilize an exogenous network. Chitosan-based 3D-bioprinted scaffolds recapitulate the real tissue with high exactness and maintain cell morphology and stability over long periods.108 Microcarrier-laden bio-inks also enable excellent interactions between cells because of an intrinsic extreme cell density. Recent investigations have proved that chitosan-based bio-inks could be excellent applicants for 3D-bioprinting demands.117
3.3. Biodegradability and mechanical stability
Degradation of bio-inks directly depends on their composition, element concentration, added particles, temperature, and other external conditions. External stimuli including enzymes, environment pH value, electrical and magnetic fields, and temperature can influence the external-responsive polymers and readily dissolve them, which changes the 3D-printed structure. The biodegradation degree of the scaffolds plays a pivotal role in cell-laden constructs and restricts the selection of bio-ink composition, because the cells should implant within the 3D scaffolds with improved biodegradability which could reshape their environment.118,119
According to the literature, the biodegradation of chitosan-based scaffolds is mainly influenced by the concentration, molecular weight, degree of deacetylation, and swelling behavior of chitosan.120 In PBS/lysozyme solutions, due to the low tendency of amine groups to lysozyme, highly deacetylated chitosan degraded at a low rate, which was determined by gravimetric degradation techniques.121
Chitosan-based bio-inks possess adequate mechanical properties to keep encapsulated cells alive and provide a suitable microenvironment similar to the native tissue which is gradually replaced by the extracellular matrix generated by the cells.122 Indeed, in the first stage of neo-tissue creation, cell accumulates do not possess sufficient mechanical stability; subsequently, they will be able to aid each other using cell–cell adhesion mediated by cadherin accompanied by extracellular matrix deposition. Nanoparticles and microcarriers could play a role as an environment for cells and ensure the structural stability of the bioprinted constructs. The mechanical characteristics of the bioprinted scaffolds affect their permeability and diffusion properties for the required oxygen, nutrient, and waste exchange.10
3.4. Immunogenicity
In the accurate preparation and effective implantation of bioprinted chitosan scaffolds for various medical demands, immunogenicity regularly poses a significant barrier by causing an immune response. The implantation of inappropriate bio-inks can cause a series of infections. Recent in vivo and in vitro studies show that 3D-printed scaffolds composed of biopolymers such as chitosan complexes cause a low-level immune response in conjunction with a high-level cell attachment and excellent cell viability.11 3D-printed structures created with bio-ink composed of hydroxybutyl chitosan/oxidized chondroitin sulfate activated only a minimum amount of pro-inflammatory gene expression of macrophages and restricted intense immune responses within a week for RAW 264.7 cell line samples.123
3.5. Feasibility and affordability
Indeed, recent reports related to bioprinting of hydrogels indicate the practicability and possibility of various chitosan composites for utilization in a wide range of biomedical engineering sub-branches. Crosslinking mechanisms of chitosan intensively influenced the bioprinting of hydrogels and selecting of the suitable crosslinking methods capable of facilitating bioprinting processes.10 The excellent ability of printed chitosan-containing constructs to adapt to human tissue/organs, and the improved efficacy of cells and hydrophobic/hydrophilic drug delivery, have made them excellent candidates for clinical trials. A large number of in vivo studies on 3D-printable chitosan-based bio-inks proved that these materials could be the key to answering difficulties in tissue engineering.10,13,124
While a few kinds of bio-based polymer (e.g., collagen, fibrin, Matrigel) are expensive, most bio-inks, especially those that are chitosan-based and those made of a combination of natural and synthetic polymers (e.g., PEG, Pluronic F-127, microcarriers) are affordable for 3D-bioprinting demands. Chitosans with various molar masses, deacetylation degrees, and reasonable prices are available on the market. Moreover, cell-laden bio-inks, especially ECM-based bio-inks, are more costly to prepare and need very advanced techniques for their preparation and storage.95 Indeed, bio-inks consist of plentiful cells and the number of cells is mostly related to their size and the rate of extracellular matrix deposition as well. The growth of cells in the required large quantities is not only not cost-efficient but also time-consuming and labor-intensive. Decellularized matrix-based extracellular bio-inks are not cheap because a significant amount of real tissue is required to form a low amount of bio-ink (Fig. 4).10
 |
| Fig. 4 Characteristics of excellent bio-inks and 3D-printed structures. (A) Shows the cell encapsulation process in the bio-ink, preparation for 3D-bioprinting, and finally, an SEM image of a Y79 cell-laden 3D-printed alginate/pluronic-based scaffold.125 (B) Microphotographs of HaCaT cells seeded on 3D-printed chitosan structures with a chitosan film at the base and with Neutral Red staining after 20 days. (B. 4) Microphotographs of Nhdf and HaCaT cells after 5 weeks seeding them together on a 3D chitosan construct with a film of chitosan at the base upon Neutral Red staining.126 (C. 1) The combination of the biodegradation and tissue regeneration mechanism of cell-laden 3D structures. (C. 2) The biodegradation and extracellular matrix replacement of HeLa cells encapsulated in a hyaluronic-acid-based hollow fiber after 15 days. (C. 3) Presents fiber-shaped tissue regeneration of red-fluorescing HeLa cells coated with green-fluorescing 10T1/2 cells after biodegradation of scaffolds.127 | |
3.6. Advantage of chitosan bio-inks
An “ideal bio-ink” for 3D-bioprinting is a bio-based material with excellent (a) printability, possessing characteristics which make a material printable (e.g., appropriate viscosity, crosslinking mechanisms), must be able to withstand forces applied during the printing process and have a suitable structural post-printing stability, (b) bioactivity (biocompatibility, high post-printing cell viability, encouragement of cellular adhesion, growth and proliferation, and good porosity to foster nutrient transportation), and (c) mechanical properties (strong enough to withstand forces experienced by native tissue, resilient and biodegradable).128
Selection of the right bio-ink for the 3D-bioprinting process requires biomaterials with optimized specific characteristics (e.g., physical parameters). Thus, successful implementation of 3D-bioprinting processes requires a properly optimized rheological profile of the utilized bio-ink. A solution with too high viscosity cannot be printed properly without applying excessive pressure, and subsequently will block the extrusion discharge tips, leading to a breakdown of the printing process. However, printing with very low viscosity bio-ink will also fail, as the desired shapes cannot be obtained with sufficient accuracy and longevity. Therefore, to control surface-tension-driven flows to avoid droplet formation driven by surface-tension and drawing straight filaments from the bio-ink solutions, a viscous material with appropriate viscosity is required. According to the literature, bio-inks with viscosity between 30 mPa.s and 6 × 107 mPa.s are needed to apply micro-extrusion bioprinting techniques.30,70 At the same time, the concentration of additives in the bio-ink matrix determines the crosslink density of the resulting network. This could result in controlled mechanical properties, resulting in controlled in vivo degradation.
Besides other noticeable characteristics of excellent bio-inks, especially those used in 3D extruder-based bioprinting systems, they have a highly hydrated, physiological structure to ensure cell viability, to avoid drying, and to preserve the cell membrane integrity against various types of mechanical damage during the different steps of the printing process (e.g., shear stress and abnormal pressures).
Among all 3D-bioprintable bio-inks, only a number have obtained approval from the Food and Drug Administration (FDA), a federal agency of the United States, which is necessary for them to be applied for medical use. Fortunately, the application of chitosan-based biomaterials is commonly accepted for use in drug delivery and tissue engineering. Nowadays, understanding the requirements for FDA approval, however, requires more appropriate precautions in additional clinical trials involving chitosan-based drug carriers. Thus, a limited number of companies such as West Pharmaceutical Services, Inc. are currently working on trials for new drug delivery systems based on chitosan's derivatives to develop FDA-approved drug carriers.129 A broad viscosity range, various routes to crosslinking, and controllable mechanical properties of chitosan-based bio-inks by introducing numerous types of reinforcing additives allow for tailoring chitosan-based bio-inks to be easily printable. Moreover, chitosan-based hydrogels have shown excellent cell viability and high bioactivity. The combination of these versatile combinable and adjustable parameters allows chitosan-based bio-inks to reach the top of the list of ink candidates for 3D-bioprinting applications.
In the following discussion, the authors have tried to give readers a more comprehensive insight into the full potential of chitosan-based bioprinted platforms by focusing on the individual characteristics and applications in the context of 3D scaffolds. Collating such information will ensure a deep understanding of interactions between the cells and the chitosan matrix, and it will help to build new regenerative approaches based on chitosan-based 3D-bioprinted artificial tissues. In Table 3, a comprehensive list of 3D-printed scaffolds with different printing approaches and applications in the biomedical field are tabulated to present an overview of a vast number of publications regarding the use of chitosan-based bio-inks in recent years.
Table 3 Recent paper related to printed chitosan-based inks/bio-inks, 3D-bioprinting characteristics, and their applications
Bio-ink |
3D-bioprinting method/process conditions |
Goal/application |
Ref. |
Chitosan |
Extrusion-based bioprinting |
To prepare scaffold with high flexibility and organized microfiber networks to promote cell growth |
34
|
PCL-DA/PEG-DA/chitosan |
Visible light 3D-printing |
Promoting cell adhesion and cell differentiation onto a new platform |
130
|
Chitosan/gelatin 2.5–7.5% |
Extrusion of different percentage composites |
Fibroblasts grew well but fidelity was low |
131
|
Collagen/chitosan |
Extrusion and printing |
For axon regeneration and neurological recovery |
132
|
Chitosan and PEG-diacrylate |
SLA with Irgacure 819 |
Poor resolution of features, weak mesenchymal stromal cell (MSC) engraftment/survival |
31
|
Hepatocytes combined with gelatin/alginate/chitosan hybrid system |
Double-nozzle low-temperature deposition |
Liver physiological simulation systems |
133
|
Chitosan and raffinose in acetic acid |
Printed at −14 °C, gelation in KOH solution, macrofibre size, pore size of 200 μm |
Keratinocytes and fibroblasts |
126 and 134 |
Polyelectrolyte/chitosan/gelatin |
Using a 6-dispensible regenHU 3D-bioprinter, printed onto a 27 °C bed |
Skin tissue regeneration |
135
|
Chitosan/PVA/HA |
The crosslinked fluid was sprayed on the scaffold, printing speed 10 mm/s |
Hydroxyapatite-based scaffolds for hard-tissue engineering |
136
|
Collagen/chitosan into gelatin |
Binder jetting technique, collagen/chitosan combination bioprinted into dry gelatin |
Poor resolution, good growth of neural stem cells, acceptable degradation rate (90% of the network in 12 weeks) |
137
|
Chitosan/gelatin/HA |
Extrusion of different percentage composites |
P3 bone mesenchymal stem cell (BMSC)-loaded scaffold for osteochondral tissue regeneration |
138
|
Bioceramic (brushite)/chitosan |
Multi-jet 3D-printing |
A bioceramic scaffold utilized as a drug delivery agent for bone tissue regeneration |
139
|
Chitosan-coated alginate |
Printing of CaCl2 through a coaxial extrusion method and crosslinking by EDC and genipin |
Hepatocyte (HepaRG) culture, anisotropic mechanical properties of a scaffold |
140
|
Alginate, xanthan, chitosan, gelatin, κ-carrageenan, and GelMA |
Extrusion of layer-by-layer of Kca2 and GelMA10 with separated syringes |
Study of characteristics and 3D-printability |
141
|
Gelatin/chitosan |
Extruding the polymer at the ambient temperature associated with vacuum freeze-drying |
Increase growing of stem cells |
142
|
PLA/chitosan, PLA grafted maleic anhydride/chitosan |
— |
Nontoxic antibacterial scaffold for tissue engineering applications |
143
|
Hydroxybutyl chitosan |
Extrusion of hydroxybutyl chitosan |
Thermoresponsive gel, chondrocytes, grew, cartilage tissue, and cardiac fibroblasts |
144 and 145 |
Chitosan-g-oligo |
SLA 3D-printing |
Treating spinal cord injuries and other neuronal degenerative diseases |
146
|
BMSC-laden gelatin/sodium alginate/carboxymethyl chitosan |
Homogeneous plotting temperature controlled at 15 °C, printing speed 1 to 10 mm s−1 |
Antibacterial scaffold for bone mesenchymal stem cell (BMSC) delivery |
98
|
Chitosan-g-oligolactides |
Two photon-induced microstereolithography (laser stereolithography) |
Study of the characterization and mechanical properties of scaffolds |
147
|
Chitosan, PCL diacrylate, PEG-diacrylate |
SLA 3D-printing |
Faster growth of fibroblasts (L929) |
130
|
Chitosan/HA/glioma stem cell |
3D-printing via extrusion-based bioprinting |
Cancer treatment |
148 and 149 |
CPC containing chitosan/dextran/BSA |
Extrusion of cement by 3D plotting system BioScaffolder |
Loading of the CPC paste, loaded protein, better efficiency of growth factors |
150
|
Silver-loaded/lactose-modified chitosan coated on bisphenol-A-di methacrylate and triethyleneglycol dimethacrylate/E-glass |
Inkjet-based printing |
It could be used as an antibacterial implant for bone tissue reformation |
151
|
N-Carboxyethyl chitosan–oxidized dextran |
Direct-write or extrusion of hollow hydrogel fibers |
Printing different-scale cost-efficient vasculatures |
152
|
Chitosan coated on PLA 3D-printed scaffold |
Extrusion-based printing at 200 °C, cylindrical shape design with pore sizes of 0.3 × 0.3 mm2 |
Tissue engineering: to promote growth of human fibroblast cells |
106
|
Chitosan-coated hydroxyapatite composite |
Extrusion-based bioprinting with printing air pressure of 0.35 MPa and printing speed 8 mm s−1 |
Using as drug (rhodamine B and bovine serum albumin) carrier agent |
153
|
Chitosan and its allyl substituted derivatives |
SLA bioprinting with a printing speed of 1.5 m s−1 |
No application mentioned |
33
|
N,O-Carboxymethyl chitosan-Ca2+-polyphosphate complex |
Printing was performed at 25 °C using a pressure of 1.4 bar and a printing speed of 18 mm s−1 |
Alternative tissue-engineering solutions |
154
|
Carboxymethyl (CMC)-chitosan |
Direct-write printing of CMC following ionic crosslinking |
Human neural development |
155
|
Polycaprolactone/chitosan |
Electrohydrodynamic (E-jet) 3D-printing |
To promote regeneration of cartilage tissue, blood vessels, and skin using human embryonic stem cell-derived fibroblasts cells |
156
|
Chitosan-coated 5-FU loaded-alginate tablet |
Printing of CaCl2 with 5-FU loaded-alginate through a hot extrusion method |
Controlled drug delivery |
157
|
Chitosan complexation with serum proteins |
Extruded by a printing rate of 150 mm min−1 |
Facile cell encapsulation can be performed, study of the mechanical stability of the prepared scaffold |
122
|
Tricalcium phosphate-chitosan/collagen |
SFF printing, plasma-sterilization, low-temperature gas plasma |
Enhancement of bone tissue formation and bone parts replacement |
158
|
Chitosan/eggshell membrane-derived calcium phosphate |
Extrusion-based printing via layer-by-layer deposition |
Bone graft application |
159
|
Chitosan, chitosan/pectin, chitosan/genipin |
Extrusion of chitosan-based bio-inks into sodium hydroxide solution |
Promoting osteoblast proliferation and mineralization |
|
Hyaluronate/chitosan/adipic acid dihydrazide/ATDC5 chondrocyte |
3D-printing via extrusion-based bioprinting |
Potential application in tissue engineering |
160
|
Chitosan–gelatin |
3D-printing using microreplication and freeze-drying techniques |
Highly porous scaffold with average 100 μm pore size suitable for hepatocyte culture |
161
|
Quaternized chitosan-grafted polylactide-co-glycolide/hydroxyapatite scaffold (PLGA/HA/HACC) |
— |
To boost the regeneration of impaired bone tissue |
162
|
4. Chitin/chitosan
In 1811, Henri Braconnot introduced the term fongine for the very first time, which was later referred to chitin in 1823, when Odier extracted it from the elytrum of the cockchafer beetle. This word is taken from the Greek word χιτών (chitón), meaning coat of armor. Indeed, Braconnot and Odier are credited as the finders of chitin from natural resources, which is well known these days as the first superabundant marine polysaccharide, and also it has taken second place among all existing polysaccharides in nature.163 Chitin is a structural polysaccharide that is almost always associated with proteins.164 Charles Rouget successfully designed and performed a process in 1859 in order to hydrolyse the acetamide group of chitin by boiling it in concentrated KOH to achieve acetate ions and an –NH2 group in order to produce a polymer with improved characteristics.165 Later, this product was called chitosan by Felix Hoppe-Seyler in 1894. Generally, the deacetylation degree is determined by the ratio of glucosamine to the N-acetylated glucosamine units existing in the structure of chitosan. So far, three major deacetylation processes of chitin have been reported: (a) chemical deacetylation, (b) enzymatic deacetylation, and (c) microwave-assisted deacetylation (see Fig. 5). Deacetylation of chitin, the linear amino polysaccharide, at various levels leads to chitosan, a semi-synthetic commercial amino polysaccharide, with different properties; however, owing to the possibility of depolymerization and side reactions, the product is rarely completely deacetylated (100%). The standard deacetylation degree of chitosan for biomedical demands should be around 75 to 98%, as provided by the pharmaceutical industries. Various sources of chitin and, subsequently, chitosan exist in nature from animal, fungi, and plant sources. Importantly, on an industrial scale, the two primary resources of chitosan are referred to as crustaceans and fungal mycelia.166
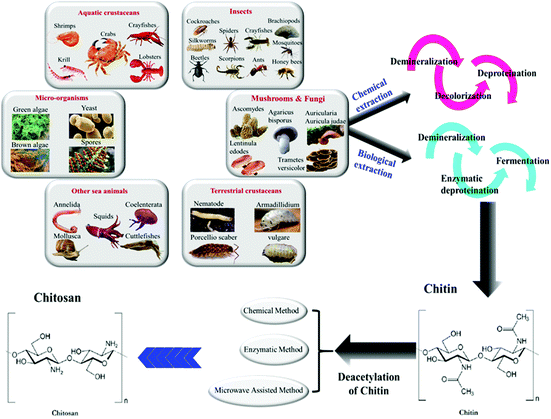 |
| Fig. 5 Discovered resources of chitin and chitosan.169,170 In this graphic, six primary natural resources of chitin from both animal and vegetable sources are illustrated. Chitin is mainly extracted from these resources through a chemical or biological extraction method, including series processes of demineralization, deproteination (chemical or enzymatic), decolorization, and fermentation; however, using different resources requires different orders of these steps. As the last step, chitosan is produced from chitin by deacetylation via chemical, enzymatic, or microwave-assisted methods. | |
4.1. Animal resources
The predominant source of chitosan, crustaceans, stems from parts of marine species like shrimp shells, crab shells and the shells of freshwater lobster (crayfish), etc. It is commercially feasible to extract chitosan from seafood waste, in particular if it involves carotenoid recovery (e.g., crustacean shells). Insect cuticles as in honeybees and crayfishes, terrestrial crustaceans including Annelida, and squid pens, etc. are other animal resources for the extraction of chitin/chitosan (see Fig. 5).167,168
4.2. Vegetable and fungi resources
Besides the animal sources, chitin can be found in some vegetable resources such as mushroom envelopes, green algae cell walls, or yeasts (see Fig. 5). In general, applications of vegetable-extracted chitosan for biomedical demands have been prioritized over crustacean because their healthcare privilege and less allergenic side effects as well. Furthermore, mushroom and other fungi-based sources of chitin provide several advantages, guaranteeing that the resulting chitosan has more constant properties. Indeed, chitosan obtained from mushrooms usually has a narrower molecular mass distribution than sea-animal-based resources, and it can also vary in molecular weight as well as in terms of the deacetylation degree.166
5. Chitosan-based inks for 3D-bioprinting
5.1. Challenges and limitations of 3D-bioprinting of chitosan-based inks
Although great strides in mimicking native tissues by chitosan have been taken so far and many successes have been achieved, there is still a great deal of effort necessary to create artificial organs which work as human spare parts. To deal with this challenge, inspite of bioprinting techniques, the characteristics of bio-ink are required to be optimized. For instance, the concentration of hydrogels in bio-ink solutions, the molar mass of the applied hydrogels for the preparation of ink, along with the final chemical composition of the printing solution, which is mainly affected by additional agents (e.g., reinforced additives, polymers, nanoparticles, etc.), are indispensable factors controlling the printability of bio-inks. Nowadays, chitosan is available on the market with various molar masses (low, medium, and high molar mass) and a wide range of deacetylation degrees. Using chitosan with different molar masses as a precursor for bioprinting will lead to different printing results; to be more precise, printing 3D patterns using low molar mass chitosan will result in superior dimensional resolution, printing fidelity, and more mechanically rigid constructs.31
The deacetylation degree is defined as the proportion of existing glucosamine to N-acetylated glucosamine in the chitosan structure. Furthermore, chitosan is known as a pH-sensitive biopolymer, soluble in weakly acidic solutions, especially in acetic acid. The solvent pH value (acid/water volume ratio of the solvent) controls solubility of chitosan and viscosity of the chitosan hydrogel solution (e.g., a more viscous hydrogel is achieved in solutions with higher acid to water ratios). Needless to say, both the deacetylation degree of chitosan and the pH value of the solvent medium directly affect the solubility of chitosan, and the solubility directly influences mechanical/rheological properties of chitosan hydrogel solution such as its viscosity; flexibility; reactivity; and heat conductivity, and indirectly affects the characeristics of the final printed platform in terms of its swelling, and tensile strength; the pore volume, porosity, and its specific surface area. Moreover, it controls the biological characteristics by modifying adsorption, antioxidant, bioavailability, and bioactivity properties as well.31,171
3D-bioprinting of dilute solutions not only decreases the printability of the utilized inks, but also the prepared 3D structures have poor mechanical stability and low printing fidelity. On the other hand, 3D constructs printed with viscous bio-inks and higher crosslinker densities have a better chance of higher printing fidelity using 3D-bioprinted devices. This entails making use of bio-inks with high viscosity, which are not recommended for biomedical applications (especially as implants, oral delivery systems, etc.) because of their low bioactivity (e.g., serious biocompatibility problems).60,109,172 The density of living cells in the bio-ink also affects the viscosity of the hydrogel. Suspended cells within the matrix of the bio-ink, along with the other existing ingredients, tend to aggregate and precipitate. Aggregates also may cause blocking of the nozzle, which can additionally lead to excessive mechanical stress on the cells, causing the above-discussed decreases in cell viability, and non-uniform droplets.
The gelation rate of bio-inks is another crucial parameter, involved in the shape stability/instability of 3D-printed platforms. Pure chitosan solutions take too long to form gel (ca. 10 min). Therefore, initially, insufficient mechanical behavior and inadequate bioprintability made chitosan an unsuitable bio-ink for the production of complex 3D frameworks when applied in its pure form.10 Fortunately, nowadays, excellent progress has been made in new, practical methods of in situ crosslinking of bio-inks, such as aerogel crosslinking, and printing in a crosslinker pool. Emerging effective natural crosslinkers (e.g., genipin for crosslinking of chitosan-based bio-inks), molecular weight, controlling printing temperature, and various additives (e.g., various types of nanoparticles and salts), together could help to improve the gelation speed of chitosan-based bio-inks, up to a point.173
The development of bioprinting techniques also plays a significant role in paving the way to an improved preparation of 3D networks with higher resolution and higher printing speed. Hydrogels capable of 3D-bioprinting through different bioprinting methods are needed to fulfill specific requirements; for example, generally, hydrogels with a viscosity below 10 mPa.s are welcome for the inkjet 3D-bioprinting process.30 In addition, inkjet-based bioprinting requires a homogeneous solution with appropriate viscosity, as a higher viscosity means that an inkjet printer requires more energy to eject a microdrop, which may damage the laden cells. Furthermore, bio-inks with too high a viscosity may not only impede the sufficient transport of oxygen and nutrition to the cells but also reduce cell motility. On the other hand, only high-viscosity structures can maintain their structure and shapes for hours.174 One of drawbacks of chitosan for inkjet-based bioprinting uses is its too high a viscosity. The addition of additives can tackle this problem, but it is not an ideal solution, as useful additives such as surface-active substances can adversely affect the survival of cells.
According to thermogravimetric results (TGA), polysaccharide chains like chitosan start to decompose at temperatures >230 °C;175,176 therefore, adding reinforcing additives such as magnetic particles, carbon-based materials, and other nanoparticles should be performed at lower temperatures. In SLA-based bioprinters, which uses UV light as the energy source for proceeding with the printing process, if they exceed the predetermined threshold of the required photo-initiator concentration, the radiation may cause serious damage to the viability of encapsulated living cells and to cell adhesion as well.
After successful bioprinting of chitosan bio-ink, still there are a number of challenges and factors which should be considered. The 3D construct networks, due to adsorption of moisture (hydration) and other possibilities, have an excellent potential for swelling.25 Subsequently, porosity and pore volumes may dramatically decline mainly because of the struts’ post-processing shrinkage (channels may be plugged, or pore diameters could be smaller than required). Anticipating these phenomena in designing 3D patterns before the printing process, and implementing viscous bio-inks with a higher density of crosslinker, could reduce the damage.32
5.2. Evolution of chitosan derivatives for 3D-bioprinting
There is no question that the main parameters that are directly involved in the development of 3D-bioprinting are (a) printability (e.g., printing fidelity, resolution, construct, and shape stability) and (b) cell encapsulation (e.g., cell viability, proliferation, differentiation, and tissue formation).177 Like 3D-printing, 3D-bioprinting is affected by many other parameters such as mechanical strength and elasticity, shear-thinning properties, and also biocompatibility. Increasing demands for new and efficient bio-inks induced extensive research on material properties to identify their unsuitable characteristics and find an ideal bio-ink for 3D-bioprinting applications.25,93
Chitosan, as a prominent biopolymer, with some modification, meets almost all these requirements, and in order to achieve more adequate bio-inks, a wide range of chitosan-based materials has emerged in recent years. Firstly, neat chitosan bio-ink was used to produce 3D scaffolds, and with the passage of time, researchers have developed a variety of chitosan derivatives to be 3D-printed for in vivo body use. So far, many published reports have validated that both physical and chemical approaches are applicable to crosslink the polysaccharide chains of chitosan.30
In the last few years, extensive application of extrusion-based methods (“direct-write” techniques) to prepare chitosan-based 3D scaffolds is a sign of its significant benefits for potential tissue engineering applications, mostly owing to its clear-cut processing approach leading to the appearance of simplicity and greater diversity of this technique.152,155 Direct-write compatible bio-inks have been developed for diverse uses from micro stents to cellular scaffolding. Generally, incorporation of cellular inclusion may also be taken into account, and owing to the harmfulness of curing agents, biological components are usually introduced in a separated step. For instance, by adding silk particles to a chitosan scaffold, not only a 5-fold increase in compressive modulus will occur, but also it leads to enhancing the accuracy of the bioprinting process and the stability of the prepared scaffolds as well.178 Extrusion of chitosan–HA and silk into sodium hydroxide/ethanol and a methanol bath, respectively, is a case in point. In recent reports, in order to facilitate crosslinking processes shortly after the time of writing, jammed soft granular gels were utilized for better printing in a direct-write fashion, also letting colloidal systems and cells remain supportless inside the gel solution. Currently, chitosan-based biomaterials are widely crosslinked by NaOH in extrusion-based bioprinting (EBB) methods for bioprinting perfusable vessel-like microfluidic channels.179 At the same time, a hollow tubular framework was achieved by coaxial nozzle 3D-printers, where the polymer from the outside core and the crosslinker solution from the inner core were co-printed.152 Laser-based printing (especially SLA) mostly requires bio-inks with photosensitive properties and limited cytotoxicity of additives, such as photo-crosslinkers, which might lead to possible cell or DNA damage along with the lasers’ UV/near to UV light.180 So, the application of this method for the preparation of chitosan-based 3D patterns has had only limited success.31,140
So far, many types of research associated with the bioprinting of marine polysaccharide biopolymers such as chitosan, alginate, agarose, and their blends have been reported. In this regard, a multi-nozzle 3D-bioprinting process working in low pressure/ambient temperature operational conditions was designed to be applied for the deposition of bioprintable polymers such as alginate and living cells simultaneously.
To minimize the cell viability reduction caused by mechanical forces and deformations during bioprinting, the rheology of bio-ink was precisely examined.181 The results of mechanical tests have showed an influence of the following parameters on cell viability: the geometry of the nozzle, pressure dispensing, material concentration, and material flow rate.182,183 Campos et al.44,184 have performed a full study on the impact of bioprinted agarose-based materials, from pure agarose to different blend forms of it with collagen and chitosan polymers, on human MSCs and the differentiation of cells into the osteogenic or adipogenic lineage. In this report, a live/dead cell viability above 95% survival was unconditionally observed even after three weeks. The findings validated that the cells tend to differentiate in entirely different ways in stiff collagen and soft agarose matrix. In a rigid collagen matrix, for instance, the division of the cells happens in the osteogenic lineage, while in a soft agarose matrix, the cells primarily vary in adipogenic tissues. In another study, Demirtaş et al., for the very first time, could successfully prepare a 3D-printed platform based on a blend of cells with the composition of chitosan and hydroxyapatite (HA). In this way, the properties of 3D-printed MC3T3-E1 cell-laden chitosan and alginate hydrogel plus their composite form with nanostructured bone-like (HA) particles, which were bioprinted through an extruder-based approach, were analyzed and compared in full. The outcome of their research indicated that cell proliferation, cell survival, and osteogenic differentiation were appreciably promoted with the existence of hydroxyapatite in the alginate and chitosan hydrogel structure.30
Han and Yan185 tried to control the thermo-reversible sol–gel transition property of a self-healing supramolecular chitosan-based hydrogel using various amounts of 2D sheets of graphene oxide (GO) through electrostatic interactions between them. By varying the fraction of GO, which acts as the crosslinker, in the chitosan matrix and also the operational temperature, the gelation could be tuned over a wide range. The outcomes of their work show that, at room temperature, a mixture containing 0.2 wt% GO and 8 wt% chitosan still remained a solution; however, it will become a gel by increasing the GO amount to 0.3 wt%. In fact, the storage modulus G′ increased more with higher concentrations of chitosan and GO. Also, they successfully synthesized an optimized room temperature chitosan/GO composite, which could be utilized in various fields such as biomedical and environmental engineering. Hu et al.186 introduced a new type of electroactive biopolymer by crosslinking of chitosan with aniline pentamer (AP) through a mixture of acetic acid, dimethyl sulfoxide, and dimethylformamide solution. In this report, considerable PC-12 differentiation was reported on platforms containing AP; however, using pure chitosan samples leads to only low cell differentiation after five days. Complex networks were developed by the cells that were observed on both prepared platforms with 4.9% and 9.5% AP. In this study, the sample with around 5 wt% AP exhibited the best performance, in terms of cell differentiation; further increases of the AP amount lead to significantly worse results in PC-12 cell differentiation. Lee and coworkers122 showed the capability of chitosan–catechol to be used as a bio-ink for 3D-printing. Chitosan amino groups are changed by a reaction with the catechol's carboxylic acid. The utilization of chitosan–catechol bio-ink led to 3D constructs in regular culture media with quick complexation with serum proteins. Furthermore, the combination of metal/catechol, including small amounts of vanadyl ions in a ratio to catechol of 0.0005, significantly improved the mechanical stability and bioprintability of embedded-cell bio-inks and also illustrated almost 90% of cell viability. In the presence of vanadium, the resultant conjugated biopolymer undergoes ionotropic gelation, creating a bio-ink that is extrudable.
5.3. Crosslinking of chitosan
To improve the properties of polymers, in particular their mechanical stability, they usually are crosslinked through one of the (a) chemical (e.g., Schiff base formation, covalent crosslinking), (b) enzymatic and (c) physical (e.g., ionic, photo and thermal crosslinking) crosslinking approaches.10,187 Undoubtedly, many features of hydrogel-based scaffolds, including degradability, rate of drug release from the matrix of the bio-ink, viability, proliferation, migration of embedded cells, and release speed of loaded agents such as growth factors and biological agents, will be affected after a crosslinking process. Crosslinking of chitosan will result in higher structural stability and will provide the living cells and other biological agents with more effective environmental conditions. Covalent and ionic crosslinking (e.g., polyelectrolyte solution and multivalent ions) as chemical methods are the most crucial techniques for interconnecting chitosan polysaccharide chains.188
From a bioprinting perspective (Table 4), due to the soft and moldable nature of bio-inks, most of these solutions need to be in situ crosslinked during the printing process to be capable of maintaining their structural integrity and designed 3D shape. Usually, the selection of a suitable crosslinking strategy depends on the rheological properties of the utilized bio-ink.60 In this regard, direct interactions between chitosan chains through their complexes with other polymers such as PVA, alginate, and, more importantly, gelatin, are another common way to achieve physical chitosan-based hydrogels with elevated rheological properties.136,138,140 Indeed, considerable enhancement in the bioactivity of chitosan, as well as an improvement in cell migration and cell adhesion, is the result of an appropriate combination of chitosan and gelatin; moreover, the hydrophilicity of gelatin improves water retention and leads to promotion of the transportation of oxygen and nutrients to the embedded cells. Accordingly, by raising the concentration of gelatin in chitosan/gelatin/genipin blends, the created environment helps to improve cell proliferation.188 Using polyaldehydes (e.g., o-phthaldialdehyde) and, more importantly, glutaraldehyde to react with the amino groups of chitosan represents another popular approach to chitosan hydrogels, albeit with the possibility of existing cell cytotoxicity, so this method has been rarely utilized for the cross-linking of chitosan-based bio-inks for biomedical demands.189–191 Likewise, using enzymes for the gelation of chitosan-based hydrogels through enzymatic cross-linking has been reported,192,193 but it is not a popular approach for 3D-bioprinting and bioprinting applications.
Table 4 An overlook of printing principles and strategies for crosslinking of utilized bio-inks for extrusion-based 3D-bioprinting of cell-laden filaments as the building blocks68,194
Crosslinking methods for 3D-bioprinting |
Chitosan 3D-bioprintability |
Method |
Ref. |
|
✓ |
To improve the viscosity of bio-ink, and thus, to enhance the accuracy of the bioprinting |
123
|
|
No data |
With the aid of complete temperature control of the bioprinting process, is mostly credited for pre-bioprinting of some thermoresponsive bio-inks and is applicable for or post-bioprinting |
195
|
|
✓ |
To immediately introduce crosslinker along with bio-inks by spraying crosslinker solution on printed filaments |
141
|
|
✓ |
Using a dual-needle or core–shell nozzle (coaxial needle) to extrude crosslinker and bio-ink solution simultaneously and to stabilize the printed filaments |
140
|
|
No data |
Immersing the printing area into a pool of crosslinker agent; thus printed bio-ink, which possess a higher density in comparison to crosslinker, extrusion is performed underwater and the written stent is held in the bath until the printing is over; the temperature and viscosity of the crosslinking batch has a significant impact on bioprinting process |
195
|
|
No data |
Make use of crosslinker to create aerosol conditions to enhance quick bio-ink gelation on the printing surface |
196
|
5.3.1. Crosslinking with naturally derived materials.
Day by day, the application of natural-based materials for crosslinking of a wide array of hydrogels is, because of a smaller number of noxious by-products and the provision of more cell viability, enjoying full attention among researchers. Nowadays, genipin has attracted a great deal of interest in the preparation of chitosan-based 3D-bioprinted constructs. Indeed, there are many reports related to the use of genipin as a natural crosslinker for crosslinking of chitosan-based gels and chitosan/gelatin hydrogels as well. The in vivo cell cytotoxicity assay of genipin, which was carried out on mice, has indicated excellent results (1000 times less toxic) in comparison with glutaraldehyde, as one of the most popular classical chitosan crosslinkers, owing to genipin's bioderived nature.197 High mechanical stability of the prepared gelatin/chitosan/genipin 3D structure is found after 24 hours of the crosslinking process at ambient temperature, proving that this material could be a great candidate for segment replacement in load-bearing and calcified human tissues, such as menisci and cartilage.
5.3.2. Photo-crosslinking using UV light.
Photo-crosslinking has developed as a great strategy to tackle numerous bioprinting problems as the illumination is minimally invasive. In addition, spatiotemporal control of the light application makes it feasible to crosslink living cell-containing hydrogels, while cell viability still remains excellent. The crucial factor in utilizing photo-crosslinking in bioprinting-based preparation is the wavelength of the exposure light. The wavelength regime, which is usually UV light, is essential to ensure integrity of the structure in the 3D-printed structure. Therefore, an imminent challenge in improving new crosslinking is minimizing the light exposure during printing scaffolds. Some studies have shown that chitin and chitosan speed up wound healing and the repair process. To date, several grades of chitin and chitosan are commercialized for wound dressing, but they are mostly combined with polyester or cotton in the form of granules, filaments, or sponges composites. Moreover, chitosan hydrogel, as an occlusive dressing, is capable of contributing to healing of the impaired tissue, in suitable humid healing surroundings.7,198
Continuous UV light irradiation has been commonly used to start the photo-crosslinking of printed bio-inks, in particular in inkjet-based printers. The BioPen has been introduced in recent years to decrease the overall exposure to UV light.199 This is accomplished by projecting the illumination to the particular point at which the bio-ink has already been extruded. The BioPen is capable of 3D-bioprinting line-shaped cell-laden UV-crosslinked bio-ink for the surgical reconstruction of tissues.200 Among all extrusion-based strategies, continuous crosslinking can be considered as the simplest one; however, severe over-exposure, which can impair the cell, has to be avoided when UV light is utilized. The application of this method for chitin and chitosan hydrogels has been limited to producing 2D scaffolds. Although some reports have justified the use of a photo-crosslinking approach to generate 3D constructs,16,201,202 according to the best knowledge of the authors, there are no data on the preparation of chitosan-based 3D-bioprinted scaffolds through a photo-crosslinking methodology.7
5.4. Optimization of chitosan bio-ink rheology
As already mentioned in this study, excellent bioprinting directly depends on the rheological characteristics of the bio-ink. Therefore, the bio-ink should possess printable viscosity, which enables extrusion through the very tiny nozzles at low pressures (<4 bars). Designing bio-inks from an appropriate selection of biopolymers can fulfill the rheological requirements for 3D-bioprinting that include non-Newtonian viscoelastic behavior, typically accompanied by high elasticity, signified by a high storage modulus G′ (as a function of the angular frequency), at high shear rates
, and high viscosity at low shear rates γ.115 Mostly for successful extrusion-based bioprinting, control of bio-ink viscosity as one of the significant parameters, which affects the printability, depends on controlling the temperature or shear-thinning for various printing techniques. It is evident that bio-inks must be in the liquid phase and possess uniform composition in conjunction with the desired viscosity and shear-thinning properties for preventing nozzle clogging. Bio-ink viscosity affects the 3D-bioprinting procedure mainly at the dispenser tip, the most critical spot at the time of extrusion.93
High mechanical stability, superior capability to protect the cells, and elevated ability to precisely design pore shape and size in 3D scaffolds and printed implants need optimized viscoelastic behavior. Therefore, various copolymers and methods are applied to change and optimize the rheological properties of chitosan-based bio-inks and prepare them for 3D-bioprinting. The relatively high viscosity of chitosan-based bio-inks not only prolongs their printing time to several hours (depending on the demands) but might also lead to nozzle clogging of small-diameter nozzle-based printing techniques. In addition, low bio-ink viscosity causes a failure to obtain a 3D-printed construct with precisely defined dimensions. Demirtaş et al.30 studied and optimized the rheological and thermal gelation of chitosan and chitosan/hydroxyapatite hydrogels while using them as cell-laden bio-inks for bone tissue regeneration. The mentioned hydrogels exhibit a temperature-induced gelation behavior since fast heating leads to a quick increase in storage modulus G′. In detail, following the first increase in G′, at the optimum temperature, the moduli became constant. For temperature sweep tests, the hydrogels were heated up from a specific temperature to a fixed predetermined temperature, in order to identify the G′ and loss modulus G′′. Dynamic-mechanical measurements with a rotational rheometer as a function of frequency were applied to assess the bio-inks’ viscosity and modulus functions. During thermal gelation at body temperature, the G′ of chitosan increased 13-fold, while for chitosan/hydroxyapatite a 29-fold increase was observed. By increasing the oscillation frequency, the storage moduli increased steadily for all bio-inks, which is typical of rather elastic shear-thinning materials. The loss moduli for all bio-inks were lower than the storage moduli, which means that the elastic nature of the materials dominates, which is typical of viscoelastic gels. In addition, adding glycerol phosphate disodium salt and NaOH to the chitosan bio-inks raised the viscosity of the hydrogels as a result of internal gelation. Increasing shear rates γ led to a reduction of dynamic viscosity η for all bio-inks. Fig. 6 illustrates an overview of the distance between the existing bio-inks and an ideal one.
 |
| Fig. 6 The biofabrication window. From the more conventional bio-inks, there has been a major shift in confrontation considering the correlation between the type and biocompatibility of biomaterials (top left and bottom right).194,203,204 | |
5.5. Mussel-inspired chitosan derivatives
In oceans, special proteins are released by mussels and utilized as a bioglue for sticking to a surface. These proteins contain moieties, which are known as DOPA. Most prominently, the adhesion of these materials to rocks and other substrates under harsh oceanic conditions (e.g., destructive waves, tide-dependent water levels, leading to submersion and exposure to atmospheric conditions following a regular pattern) has been assigned to the existence of the catechol groups available in DOPA.205 Regardless of the exact chemistry of these materials, their versatility is caused by ‘catechol’ moieties in the structure of the proteins.122,205
The modification of chitosan chains with catechol units can open new doors in the medical field and be considered as an effective bioadhesive polymer for biomedical demands (see Fig. 7). Drug and gene delivery, as well as cancer thermotherapy, are listed as the most attractive fields of interest of mussel-inspired chitosan-based biomaterials in biomedical engineering. Moreover, many reports have been conducted related to the application of mussel-inspired chitosan-based structures for hemostatic materials, tissue engineering, and biosensors as well. Using mussel-inspired chemistry to modify chitosan-based bio-inks leads to the high promotion of chitosan's water solubility even for neutral aqueous solutions, and will result in improved mechanical stability, enhanced tissue adhesion, and excellent biocompatibility.206 However, recent research on functionalized chitosan–catechol materials has demonstrated that the use of glycol/chitosan–catechol instead of chitosan–catechol as a hemostatic agent had a better result. Seminal research, published by Park et al.,207 deals with making use of mussel-inspired chemistry for the preparation of a 3D-printed-chitosan construct applicable for a wide array of uses in the biomedical field. Using ethylene glycol groups gives chitosan–catechol improved anti-biofouling properties, making it a promising alternative product from cellular toxicity and in vivo skin irritation aspects.
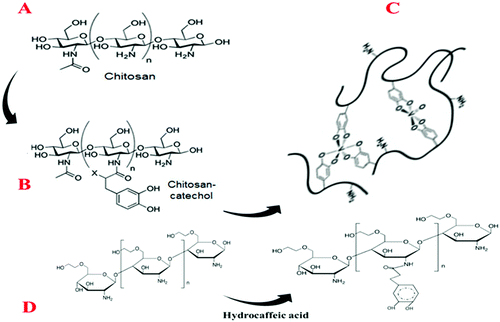 |
| Fig. 7 Schematic illustration of the molecular structure of (A) chitosan, (B) chitosan–catechol, (C) chitosan–catechol complex with vanadyl(IV) ions and (D) glycol chitosan–catechol.8,122,204 | |
Inspired by mussel-chemistry, Lee et al.122 tried to make use of an incorporated vanadyl ion/chitosan–catechol combination, as a bio-ink, for successful 3D-bioprinting through a direct writability technique. They found that the presence of a small number of vanadyl ions in the mixture of catechol-functionalized chitosan could greatly increase its printability, mechanical properties, and also it indicated acceptable cell viability. This methodology for the preparation of a cell-encapsulated chitosan-based bio-ink in fetal bovine serum (FBS) in culture medium justified that it could be an excellent approach for the manufacturing of efficient 3D bio-ink crosslinked without any external stimuli. They also showed that the bioprinting process in a medium containing 20% FBS could achieve more stable 3D networks (see Fig. 7). In another study, Wang et al.100 suggested a straightforward mussel-inspired strategy to load Ag nanoparticles and construct chitosan–polyurethane coatings to endow polyethersulfone with dual antibacterial features. This conveys the possibility of preparing various hybrid biomaterials with particular functionalities. Existing Ag nanoparticles as an antibacterial agent could appreciably elevate the antimicrobial properties of prepared chitosan–catechol 3D structures.
6. Biomedical applications
3D networks using chitosan-based bio-inks have a significant number of applications in tissue engineering (from hard to soft tissues), advanced biosensors, and drug delivery, which are considered to be the most conspicuous branches of biomedical engineering. In the following discussions, the authors of this work have given a comprehensive insight into the printing of the various chitosan-based 3D scaffolds applicable in each mentioned section.
6.1. Tissue engineering
6.1.1. Bone tissue.
Wang et al.208 used a mixture of ethanol and sodium hydroxide (NaOH) to facilitate gelation of chitosan and chitosan–hydroxyapatite composites prepared by a robotic dispensing rapid prototyping mechanism. In this technique, inks are extruded into a dispensing solution via a Teflon-lined nozzle and made a 3D construct with interconnected pores and high cell attachment. The role of NaOH is to maintain the shape of cuboid construct under hydrostatic pressure. At a high concentration of NaOH, rapid precipitation and low mobility of chitosan assist attachment of a small layer, while in low concentrations the shape fidelity and perception kinetics are changed. They found the factors involved in the preparation of the scaffolds, such as the initial height, flow rate, and pressure, which are firmly dependent on ink viscosity. Therefore, by monitoring and controlling the involved factors in various compositions they achieved an optimum composition. The degree of the constructs’ biocompatibility was analyzed by in vivo bone-cell culturing. The SEM images taken three weeks after cell seeding exhibited excellent adhesion, healthy morphology, and homogeneous cell distribution.
Demirtaş et al.30 illustrated that cells could be blended and printed with chitosan solution and a composite of the solution with nanostructured bone-like hydroxyapatite (HA). Analysis of cell proliferation and viability revealed that, using bio-solutions for printing, cells can be viable after printing. In addition, it is proved that chitosan, as a bio-printing solution, is printable. Moreover, it is shown that the existence of HA in chitosan hydrogel can enhance cell survival and osteogenic differentiation.
Wang et al.100 suggested new hydroxyethyl chitosan-based scaffolds and cellulose. HECS/CEL has a porous framework which facilitates chemical crosslinking, freeze-drying, and silica-leaching approaches, such that by generating macro- and micropores using freeze-drying, it makes possible particulate porogen procedures. Bioanalysis illustrated that, since the HECS/CEL scaffolds are contemplated to be an excellent medium for applying in bone tissue engineering, they can aid the spreading, attachment, proliferation, and viability of osteoblastic MC3T3-E1 cells. Wang et al.208 investigated beta-glycerophosphate as an osteogenic medium supplement, which is utilized as a weak foundation for the simultaneous gelation of pure chitosan at physiological temperatures and pH values. However, DNA content decreased by 50% within 12 days in chitosan. When collagen was used, DNA content increased two times. The presence of chitosan boosted osterix in both media with or without osteogenic supplements. Chitosan–collagen composites are potentially useful for cell delivery and encapsulation, or can be considered as in situ gelling materials for tissue amendment.209–211 Because cell viability must be maintained when the gel is prepared, the injectable biomaterials’ cytocompatibility is a crucial factor in cell encapsulation.212 Collagen and chitosan, which are nature-derived materials, have been investigated widely because of their capability of integration with adjacent ECM and supporting cell growth.213–215 It was illustrated that the high viability of cells in chitosan gels without additional components connected to the β-GP was inhabited by fibro-chondrocytes. Wang et al.208 reported, however, that calcium was deposited after a while. However, calcium secretion took place because of cell necrosis, which was reflected in decrease in DNA content over time. They reported higher expression, higher calcium content, and incremented ALP activity upon increasing the chitosan content in the composite of chitosan–collagen.
6.1.2. Cartilage tissue.
Li et al.123 3D-printed a homogeneous pore-sized, cell-laden implant by hydroxybutyl chitosan/oxidized chondroitin sulfate-based ink in various architectures and applied it for regeneration of cartilage tissue. In vivo and in vitro studies disclosed that the proposed implant had anti-inflammatory activity, good ability for cell delivery, non-cytotoxicity, high mechanical properties similar to cartilage tissue, and biocompatibility. The controlled shape and capability of multifunctionality besides its other characteristics made it a suitable applicant for cartilage tissue regeneration.
In a similar work on cartilage tissue regeneration, Morris et al.31 prepared an ear-shaped, interconnected, uniform, controlled internal pore-size (50 μm) scaffold through 3D-bioprinting of chitosan and polyethylene glycol diacrylate ink. They optimized its mechanical properties, printability, and cell attachment ability through monitoring and manipulating the involved operational parameters such as feed-ratios, photo-initiator concentrations, and chitosan molecular weight. They found that the best ratios of chitosan to PEGDA were between 5
:
1 and 10
:
1. Needless to say, the presence of chitosan imparts hydrophilicity and good swelling properties to the scaffold (see Fig. 8). Ye et al.216 studied infrapatellar fat pad adipose stem cells embedded in a chitosan-based 3D-printed construct and studied its capacity in cartilage tissue engineering. The in vitro survey revealed that embedded cells in the construct network in the chondrogenic environment hindered the emergence of cartilaginous-shape tissue. However, in the non-chondrogenic environment, fibroblastic morphology emerged. They found that scaffold pore size impacts on cell adhesion and proliferation. Their obtained outcomes proved that the presence of TGFβ3 and BMP6 in the scaffold architecture remarkably improved the repair process of damaged cartilage.
 |
| Fig. 8 Chitosan-based 3D structures used in bone and cartilage regeneration. (A.a1) 3D-printed quaternized chitosan (HACC)-grafted polylactide-co-glycolide (P)/hydroxyapatite (HA) construct (P/HA/HACC) applied for bone regeneration of a femoral shaft bone defect made in a rat (A.a2) and femoral condyle local bone defect made in a white rabbit (A.a3). (A.b) X-ray image of the right femoral condyle of a rabbit at week 4 and week 8 after implantation of (P/HA/HACC) scaffold with remarkable regeneration after week 8. (A.c) Bone volume regenerated per total bone volume for all the scaffolds after 8 weeks of implantation. (A.d) Lateral X-ray image of the left femoral shaft defects of a rat; bone regeneration after 8 weeks shown by the blue arrow. (A.e) Bone volume regenerated per total bone volume at week 2, week 4, and week 8 after the implantation of various 3D-printed scaffolds.162 (B.a) 3D-printed, ear-shaped low molar mass chitosan/polyethylene glycol diacrylate with a ratio of 1 : 7.5. (B.b) SEM image of the mentioned scaffolds clearly presenting the micropores of the construct. (B.c and B.d) Images with low and high magnification of the scaffold (H&E stained), showing an excellent cell distribution.30 | |
Reed et al.217 applied an amalgamation of directional freezing and 3D-printing to the controlled design of micro/macro-interconnected pores and channels of an acellular chitosan–alginate construct. This 3D scaffold not only possesses high compression resistance and acceptable swelling ability but also inhibits the denaturation process of loaded drug and protects encapsulated growth factors due to its preparation procedure in a neutral pH environment. Such constructs with lamellar pores parallel and perpendicular struts were used to recapitulate the real cartilage tissue better, showing improved swelling absorption, and accelerated cell migration at their full height and diameter, as well as promoting the regeneration cycle.
Madihally et al.117 mixed gelatin with chitosan in a mixture of deionized water and hydrochloric acid for facile and inexpensive 3D-bioprinting. Gelation ensures the enhancement of cell/chitosan interactions for application as a highly efficient thermogelling bio-ink. Subsequently, to improve its characteristics and to form hydrogels at 37 °C, they added β-glycerophosphate and cells to the ink. They concluded that a cooling mixture, vacuum degassing, and centrifugation could prevent printer clogging due to undesired gelation, the presence of substantial contamination, agglomeration, and aggregation. The authors found that a reduction in fiber size, enhancement of the rheology of the solution, and acceleration of the gelation process occurred with increments in the chitosan concentration.
6.1.3. Neural system and vascular network.
Vascular network and neural system repairs are the most challenging issues and inseparable parts of tissue regeneration. Bio-nature scaffolds such as chitosan-based 3D-printed networks have exhibited a high potential for regeneration of the neural and vascular systems. Gu et al.155 designed a new, printable bio-ink based on an agarose/alginate/carboxymethyl-chitosan complex for application in neural tissue regeneration. The prepared bio-ink possesses outstanding features such as nontoxicity, low-time gelation, elevated adhesive properties, an excellent environment for cell migration and proliferation, high stability, and a suitable porous medium for encapsulating cells. Adding carboxymethyl-chitosan to the alginate based-ink enhanced the permeability, porosity, hydrophilicity, and cell growth/viability of a 3D-printed scaffold. Besides, they applied silver particles to optimize the rheology of the bio-ink. Such 3D scaffolds not only facilitate the repair and operation of human neural systems but they can also be tailored to be applied for other tissue regeneration demands.
Zhang et al.177 used a 3D-bioprinter to create microfluidic cellular vessel-like channels with chitosan/alginate ink through direct printing. The delicate channels with a wall thickness of <200 μm were crosslinked by NaOH solution. An increase in the chitosan concentration (>4%) decreased cell viability, owing to the need for more printing pressure due to increasing solution viscosity. However, a low chitosan concentration (2%) led to weak mechanical properties. Accordingly, solutions with a chitosan concentration in the range of 2.5 to 3% were selected as optimum for bioprinting. The polymer dispensing rate had a direct relationship with wall thickness. Overall, the channels made by chitosan and alginate had excellent cell viability and could be applied for vessel network regeneration.
6.1.4. Skin tissue.
Distinctive characteristics of chitosan, such as hydrophilicity, biodegradability, and hemostasis features, have made this biopolymer a suitable candidate for skin regeneration demands.218 Moreover, chitosan enhanced wound healing by imparting fibroblast growth, making it an appropriate environment for introducing polymorphonuclear leukocytes into damaged tissue.219,220 Moreover, good swelling properties of chitosan-based 3D scaffolds ensure cell viability and a reduction in scar formation. Ng et al.131 optimized the healing and antimicrobial characteristics of chitosan-gelatin complexes for 3D-bioprinting of robust skin wound dressings. They selected chitosan instead of collagen due to its excellent printability and ready functionalization ability. Chitosan facilitated inflammatory cell migration, nutrient and oxygen penetration and resulted in cytokine formation, which accelerates wound healing.221 The carboxylate group of gelatin reacted with an ammonium group in the chitosan and formed a polyelectrolyte ink, which had a gelation temperature below room temperature. The rheological properties, storage capacity, biocompatibility, and physical and chemical stability of this polyelectrolyte ink were comprehensively surveyed. A high ink viscosity led a sharp reduction in cell proliferation and viability and, ultimately, to cell death.
Long et al.220 embedded lidocaine hydrochloride as a medicine for accelerating wound healing in extrusion-based 3D-printed chitosan/pectin structures. The printed structures exhibit improved printability and strong wet-adhesion to the skin. Due to the hydrophilicity and high porosity of the printed ink, the construct provided an excellent microenvironment similar to the real tissue for exchanging nutrients, oxygen, and wastes. Hafezi et al.124 designed a wound dressing based on genipin-crosslinked chitosan, plasticized by PEG and glycerol. By 3D-bioprinting, they controlled the geometry and the interconnectivity of the printed porous medium. The nontoxicity of the printed scaffold was confirmed by 90% viability of the skin fibroblast cell lines after two days. The in vitro study of the designed dressing exhibits acceptable swelling and ability for drug release, enhanced biocompatibility, cell attachment, and proliferation.
Thein-Han et al.105 described a favorable cellular response for buffalo embryonic stem cells encapsulated in 3D composite scaffolds consisting of biodegradable chitosan–gelatin for tissue engineering based on stem cells. The biological reaction of cell growth on the constructs suggested that mixing gelatin in the chitosan enhanced cellular efficacy. 3D-printed chitosan–gelatin scaffolds can be considered to be excellent contenders for tissue engineering. The outcomes depicted that the constructs of the skin from this proposed method had high biocompatibility. In strongly visualized GFP-expressing cell–chitosan–gelatin scaffolding systems, both cell proliferation and cell spreading are shown to be superior compared with mere chitosan. A correlation, including cell viability and replication, shows that a combination of gelatin and chitosan improves the potency of cells. Studies including analyses by fluorescence and flow cytometry and histological observations of the structures implicate a pluripotency of the polygonally connected cells after 28 days culture time. The research emphasizes that chitosan–gelatin scaffolds are excellent 3D constructs for cell beds and application in tissue regeneration.
A composite of hyaluronic acid, collagen, and chitosan with a new crosslinking procedure (a mixture of different crosslinking approaches) has been employed by Zhang et al.221 to create a bio-ink to make 3D cell-laden builds using 3D bioprinting for skin tissue engineering. Fibroblasts were distributed into the bio-ink to generate 3D cell-laden living constructs. A bio-ink composition of chitosan of 8 wt%, hyaluronic acid of 0.5 wt%, and 10 wt% of collagen was selected to make skin-imitating constructs. The morphology of the constructs has a layered structure like human skin. The tensile strength for chitosan/HA/collagen (CS/HA/Col) was 29.9 kPa, and this value for CS/HA/Col–tyrosinase was 39.1 kPa. The Young's modulus for CS/HA/Col was 0.170 MPa, and for CS/HA/Col–tyrosinase was 0.313 MPa. These values show that CS/HA/Col–tyrosinase proposed an enhanced mechanical strength that is similar to human skin (1.46 ± 0.26 MPa (ref. 222)) because of chemically-induced crosslinking using tyrosinase. The rate of degradation of CS/HA/Col was 81.99%. This value for CS/HA/Col–tyrosinase in thirty-five days was 55.34%.
In comparison with a non-crosslinked CS/HA/Col scaffold, a tyrosinase pre-chemical crosslinked hydrogel (CS/HA/Col–tyrosinase) shows a significantly slower degradation of these biocompatible constructs of the skin, featuring a high cell survival rate. The outcomes depicted that the constructs of the skin from this proposed method have good mechanical features and biocompatibility. Moreover, with increase in the tyrosinase concentration the mechanical strenght increases and degradation rate decreases. Hence, the new crosslinking method (by tyrosinase) is more desirable. Fig. 9 shows examples of how chitosan-based bio-inks could be effective for the regeneration of soft tissues such as neural and skin tissue.
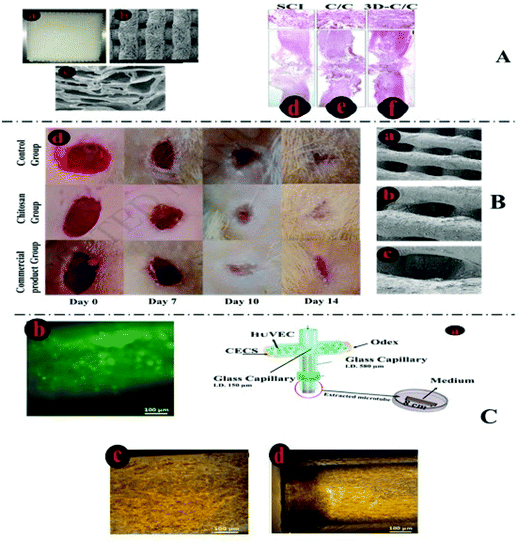 |
| Fig. 9 Chitosan-based 3D-printed structures for soft tissue regeneration. (A.a) 3D structure made by collagen/chitosan bio-ink. (A.b and A.c) SEM image of the 3D construct, exhibiting interconnected pores with a specific shape and diameter. This bio-ink was applied by two different methods: freeze-drying (C/C) and 3D-bioprinting (3D–C/C). HE analysis shows that 3D-printed scaffolds could repair the injured tissue and made smaller cavities and a more linearly ordered structure in comparison with C/C (A.d, e, f).132 (B.a, b, c) SEM image of seeded 3D chitosan scaffolds supporting normal human dermal fibroblasts (NHDF) and the human keratinocyte cell line HaCaT. (B.d) Wound healing images in the modified and non-modified groups.126 (C.a) Microfluidics-based synthesis of a 3D, cell-laden chitosan structure in a glass capillary. (C.b) Fluorescent microscopic image of viable human umbilical vein endothelial cells inside the tubular microgels at day 0. On the third day (C.c), the HUVECs exhibited spiny structures and started to disperse through the pipes, and after a week, the cells survived on the inner face of the tubular microgels (C, d).152 | |
6.2. Drug delivery
3D-printing methodologies and applying various compositions of 3D-printable ink allow for overcoming the existing shortcomings such as burst and off-target release of drugs. An effective technique is magnetizing printed constructs by adding magnetic particles. For instance, magnetic particles like Fe3O4, Fe2O3, CoFe2O4, MnFe2O4, and ZnFe2O4 are widely used in biomedical technology because of their remote operating potential, mobility, active movement, accurate target localization abilities, and external control on the cargo carriers.
In an intriguing work, a high-resolution 3D-printed magnetic micro-swimmer-shaped methacrylamide chitosan crosslinked by PEG was applied to carry doxorubicin as a chemotherapeutic drug using a photocleavable crosslinker and controlled release of the drug by an external light stimulus. The movement, direction, and path of the micro-swimmers can be monitored and controlled. The presence of chitosan in the bio-ink ensures the biocompatibility and biodegradability of the carrier over a span of 204 h.223
Yan et al.224 3D-printed carboxymethyl chitosan with snake gourd root/astragalus polysaccharide patches in various structures (circular, square, and rectangular) by a melt-extruding 3D-printer and surveyed their controlled-release ability of bovine serum albumin for possible diabetes therapy. Their observation justified that the shapes of the utilized patches influenced their efficiency; to be more precise, the circular patches exhibited a higher release rate than square and rectangular patches. Besides, the net drug release in the acidic region was more pronounced than the alkaline region. The development of Schiff bases between the (–NH2) on carboxymethyl chitosan and the aldehyde groups on oxidized polysaccharides throughout the gelation procedure induces a sharp decrease in the toxicity of the printed constructs and increases the viability and cell proliferation.
Since direct ink writing offers easy composition and encapsulation of hydrophobic/hydrophilic drugs or proteins into 3D-printed constructs, Sommer et al.225 applied this technique to 3D-bioprinting of chitosan-modified silica-nanoparticle-stabilized emulsion, which contains hydrophobic compounds in the specific location of its structure, helping the controlled release of the drugs (see Fig. 10). In another report, 3D-printed bioceramic scaffolds coated with chitosan hydrochloride enable the transport and delivery of bioactive molecules such as rhBMP-2, heparin, and vancomycin with minimum biological activity reduction in printed shapes in comparison with the initial state before the printing process. The presence of the chitosan hydrochloride not only increased the biocompatibility of the carrier but also caused the change in the profile release of vancomycin from 1st order burst release to 0th order continual release in the range of 0.68 to 0.96% h−1.139
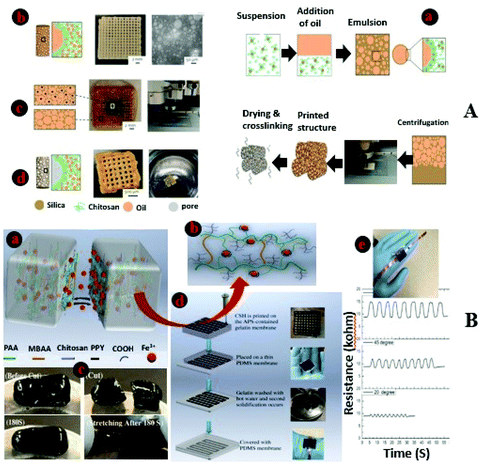 |
| Fig. 10 Drug-delivery and sensor applications of chitosan-based 3D-printed constructs. (A.a) Preparation pathway of silica–chitosan complex soft materials by 3D-bioprinting of stable emulsions. The oil droplets were applied to encapsulate hydrophobic drugs or to model pores after the structural consolidation. (A.b) 3D-printed structure with non-volatile oil–water emulsion stabilized by chitosan–silica for carrying both hydrophobic and hydrophilic compounds.228 (B.a–c) Healing process of a self-healing, chitosan-based material, which was used for 3D-printing on an APS-contained gelatin membrane to create a sensor (B.d). Resistance variation of the attached sensor on the index finger was assessed as the finger was subjected to repeated bending and relaxing from 20°, 45°, and 90° (B.e). | |
6.3. Biosensors
Recently, preparation of conductive constructs as sensors for application in health monitoring systems and biomimetic prostheses has gained significant attention. 3D-printing of sensors imparts efficient sensing ability and tailors nanocomposite mechanical and electrical characteristics.226,227 In a seminal work, Darabi et al.228 3D-printed a chitosan-based, conductive, self-healing hydrogel with improved stretchability (1500%) and repairability in 2 minutes under ambient conditions by appropriate, two-step physical/chemical crosslinking for human motion detection. The fast self-healing property of the printed structure stems from the strong ionic interactions between ferric ions, NH groups of polypyrrole, –OH and NH2 groups of chitosan, and carboxylic groups of poly(acrylic acid). This amazing sensor can record and monitor the pulse rate, breathing rate, and biceps and finger flexion by conductivity changing under compression. In another report, Wu et al. 3D-printed a mixture of chitosan and CNT with high resolution (30 μm) to achieve a structure that was self-healing at room temperature when exposed to water vapor. The synthesized magnetic sensor could retain its electrical and mechanical characteristics after the repair process. 3D constructs with various pore shapes (diamond and square) after neutralization in an alkaline environment (sodium hydroxide) exhibit remarkable stretchability and flexibility. A high conductivity (∼1450 S m−1), stretchability (strain at break ∼180%), and flexibility of the printed material make it an excellent applicant for identifying defects in human elbow movements.
7. Other applications of chitosan
7.1. Water treatment
To fight the upcoming water crisis, removing toxic organic compounds and heavy metals from wastewater has the utmost priority among environmentalists.229 Applying biocompatible materials with maximum efficiency, as well as maintaining their nontoxic and eco-friendly features, lies at the center of attention.230 The presence of (–OH) and (–NH2) in the main chain of chitosan and its capability to mix with synthetic polymers to form fibers through electrospinning increased its utilization in wastewater treatment demands.231,232 In recent years, the number of publications on applying chitosan with different compositions and shapes for the removal of nitrate, phosphate, heavy metals, and dye molecules from aqueous medium has skyrocketed.233–235 Nevertheless, there has been only one report of 3D-printed chitosan-based adsorbents for application in the removal of the pollutants from water, owing to the relatively lower demands of dimensional precision and the high costs associated with 3D-bioprinting. In this work, Zhou et al.235 synthesized a PLA@GO/chitosan bionic filter with a 3D-bioprinting and double-freezing methodology. They designed an efficient, biocompatible filter with the help of 3D-bioprinting for the remediation of crystal violet in aqueous solution (see Fig. 12a–e).
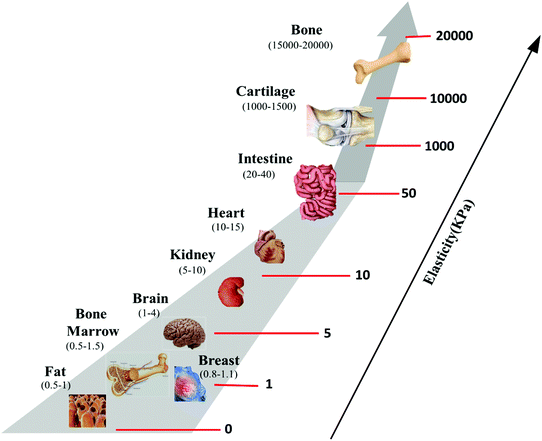 |
| Fig. 11 Variations in human tissue stiffness. The suitable stiffness for cartilage tissue regeneration is in the range of 1 to 10 MPa.239 | |
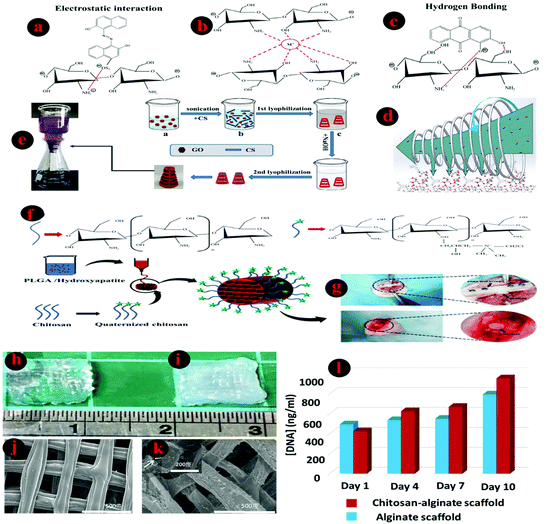 |
| Fig. 12 Other applications of chitosan in 3D-printed structures. (a–c) Mechanism of adsorption of organic and heavy metal ions with chitosan from aqueous media. (d and e) Schematic bionic construct design for effective crystal violet uptake assisted by a 3D-printed polylactic acid-graphene oxide/chitosan filter.235 (f and g) Application of chitosan in the coating of 3D structures like PLGA/hydroxyapatite to enhance cell viability and attachment for bone regeneration demands. (h and i) Image of 3D scaffold alginate and chitosan-coated alginate scaffold, respectively. (j and k) SEM images of alginate- and chitosan-coated alginate scaffolds, respectively. (l) Higher cell viability of the chitosan-coated in comparison with bare alginate scaffold using DNA as a cell sample.237 | |
7.2. Chitosan coatings on 3D-printed structures
Chitosan has been broadly applied as a coating on various 3D-printed constructs to enhance their functionality and cell attachment as well as to provide them with ability to immobilization of biological molecules such as growth factors and different drugs. For instance, Lee et al.236 enhanced mouse bone marrow MSC growth by indirect 3D-printed chitosan. First, a 3D gelatin model was printed and infiltrated by PCL; subsequently, gelatin was dissolved in an aqueous medium, and the surviving materials covered with chitosan. In the end, the PCL was dissolved in the chloroform, and a 3D chitosan structure was produced. Lin et al.237 applied chitosan to coat a 3D-printed alginate fiber, which was loaded with diclofenac to increase biocompatibility, bone-cell proliferation ability, and to enhance the drug release profile. The adsorption/desorption behavior of diclofenac enhanced with chitosan, and therefore remarkably restricted interleukin-6 and tumor necrosis factor-α discharge from macrophages. Ainola et al.238 utilized glutaraldehyde crosslinked electrospun chitosan/PEO to support a 3D-printed PCL grid for chondrogenesis (i.e., the development process of cartilage tissue). This supporting coat improved cell/matrix interactions and chondrogenesis. Wu et al.156 designed PLA/CS, and PLA-g-MA/CS complexes with a height of 123 μm and width of 21 μm through a 3D-bioprinting technique, and subsequently characterized and surveyed their biological activities such as antimicrobial properties. The authors also observed that the printed structures exhibit improved stiffness (elastic modulus), and tensile strength of 13.93 MPa and 1.47 MPa, respectively. Stiffness (elastic modulus) represents the amount of force required to deform a material to a certain extent elastically. Fig. 11 depicts the stiffness of various human tissues/organs with their determined elastic modulus.
Since the presence of a vast amount of living cells is needed for successful 3D-bioprinting, induced pluripotent stem cells are considered as ideal candidates for this matter, so that many scientists have applied this technique in recent years.239 Wong et al.240 could preserve and grow a large amount of stem cell markers without a significant reduction in their proliferation speed in chitosan sheets. Moreover, they embedded cells in a biodegradable, 3D-printed polyurethane construct and studied its impact on cell viability, but they did not survey the effect of the chitosan/polyurethane printed structure.
Also, PLGA/hydroxyapatite was printed by Yang et al.241 and covalently covered with quaternized chitosan. Due to the presence of quaternized chitosan in the printed scaffold it showed antimicrobial and osteoconductive properties. In vivo studies on several rabbit femoral condyle defects and many rat femoral defects showed a significant increase in the volume of regenerated bones. Furthermore, the scaffold deterioration rate occurred similar to the progression of infection, impacting the bone generation in the infected bone models. Tsai et al.242 3D-printed titanium scaffolds by a selective laser melting (SLM) process and then applied chitosan/magnesium–calcium silicate as a coating agent to add the bioactivity and other needed characteristics to the printed scaffold, such as hydrophilicity, mechanical stability, cell attachment, proliferation and more similarity with real bone tissue. The in vivo study showed that the coated 3D construct had a high potential for bone regeneration.
8. Future directions of 3D bioprinting based on chitosan
The accuracy and similarity of 3D bio-printed tissues/organs depend on the constructional signals of the environmental medium. In this regard, 3D bio-printing outdoes old-fashioned tissue engineering in many terms owing to its extraordinary ability to integrate various intricate architectures with improved mechanical properties and high alignment resolution in conjunction with great cell/cell sustainability to make an artificial tissue very close to real ones. However, current reports show that 3D bioprinting could not mimic sophisticated organs with complex vascular or neural networks (e.g., heart and brain) similar to real organs.243,244 Furthermore, since present studies on finding high-efficient medicines and lasting cures for sophisticated cancerous tumors or chronic wounds are mainly based on animal model investigations or monolayer cell cultures, which possess different gene expression, cell function, vascular network, epigenetics, cell/cell and cell/matrix interaction compared with human 3D tissues, more accurate answers will have to be found solely by analyzing the efficiency of the designed materials and drugs on 3D models sufficiently similar to real organs.245–247 Therefore, the advent of microfluidics in biomedical engineering triggered developing organ-on-a-chip systems to remedy the disadvantages of traditional techniques (e.g., gel confinement and lithography) for creating complicated organ/tissue models with a high extent of similarity to the architectural, microenvironmental and functional complexity of real organs on the way to achieve more efficient diagnostics, and therapies.69,248 As mentioned before, while 3D-bioprinters can produce complex organs with precise control over spatial factors, they still suffer from restrictions such as printing resolution, ideal bio-ink composition and properties, and limited co-printing ability of different cell types. On the other hand, organ-on-a-chip platforms not only ensure that designed structures have physiological, mechanical, and chemical cues in vitro but also have the ability to implement multiple experiments on a single chip (unlike many single animal experiments).249 Organ-on-a-chip devices mostly consist of microchannels and sophisticated architecture to simulate the structure of native organs/tissues. This technology is not only applied for mechanistic surveys but also used as proof of concept for drug-analyzing investigations. However, they suffer from fully controlling soft tissue-like construct characteristics. Scientists firmly believe that a combination of lab-on-a-chip and 3D-bioprinting could tackle the existing disadvantages that both techniques are facing and providing a unique methodology for recapitulating more sophisticated organs with high exactness.4,244–251Fig. 13 shows some advantages of combining 3D-bioprinting and organ-on-a-chip approaches.70,183,250–258
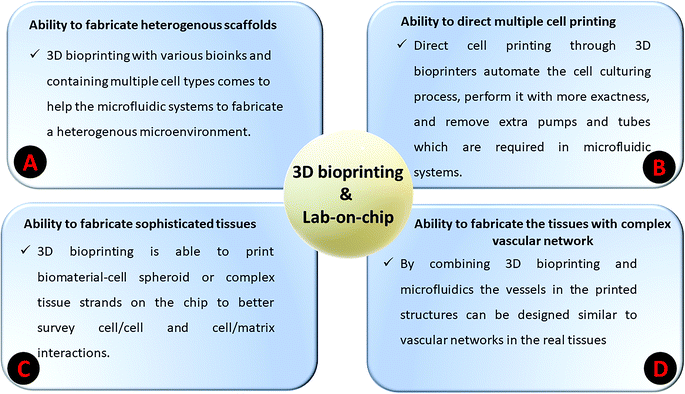 |
| Fig. 13 Benefits of combining 3D-bioprinting with organ-on-a-chip technology. | |
Recently, applications of chitosan in chips, microfluidic devices, biosensors, and sophisticated tissues like neural networks are established by a few scientists.161,259–262 Distinctive characteristics of chitosan such as the ability to prepare films and nanofiber shapes, biocompatibility and biodegradability, elevated biological activity with nontoxicity could make it an excellent choice for application as a matrix for immobilizing biomolecules, enzymes, various cells, nanoparticles, etc. on microfluidics-based chips.263,264 Wang et al.265 electrodeposited chitosan films with various thicknesses in a freestanding microfluidic channel made by dry SU-8 sheet as a polymer with high chemical stability, to enhance its biological activity through capillary effects for the immobilization of enzymes. Oxygen plasma treatment was used to change the hydrophobic characteristics of the SU-8 surface to hydrophilic and facilitate the adhesion and penetration of chitosan solution to the channel, which is applied to create a compact portable biosensor. In terms of diagnosis and in-depth cellular assessment, carboxyl betaine acrylamide functionalized electrospun chitosan nanofiber was coated with HA for utilization in a microfluidic chip for the uptake and discharge of circulating tumor cells by Wang et al.265 Their prepared platform exhibited high capability (91%) in uptake of A549, a human lung cancer cell line. Since circulating tumor cells were found in patients with lung and breast cancer, their investigation could open the door to a better diagnosis of cancer. While 3D-bioprinting for organ-on-a-chip technology has been performed for various bio-ink compositions in different areas (see Table 5), according to the best knowledge of the authors, chitosan-based structures have not yet been implemented in this technique. Hence, it is a new and challenging topic for bioengineering scientists to use and survey the possible future applications of 3D-bioprinted chitosan-based bio-inks for organ-on-a-chip approaches.
Table 5 Various bio-inks applied in 3D-bioprinting for lab-on-a-chip
Microfluidic chip substrate |
Bio-ink composition |
Bioprinting method |
Application and description |
Ref. |
Polydimethylsiloxane (PDMS) |
Cell suspended in biopolymer media |
Extrusion-based bioprinting with multi-nozzle tips |
Cancer therapy; investigating drug metabolism on MDA-MB-231 cells (human breast adenocarcinoma) |
266
|
Poly-methyl methacrylate (PMMA) |
Mixture of gelatin and fibrinogen |
Extrusion-based |
Albumin adsorption analyzing, and cyclosporine A testing on a prepared kidney tissue model |
267
|
PDMS |
Cells suspended in medium |
Extrusion-based bioprinting with four-nozzle tips |
Study of cancer cells in a co-cultured microfluidic media |
249
|
Glass and PDMS |
Alginate |
Extrusion-based |
Study of in vivo human response to drug administrations |
181
|
PDMS |
Cell suspended in biopolymer medium |
Droplet-based |
Investigation of anti-cancer drug responses of cancer cells |
268
|
PMMA and PDMS |
Mixture of gelatin and GelMA |
Extrusion-based |
In vitro drug analysis using a liver-on-a-chip platform |
269
|
Polycaprolactone (PCL) |
Tracheal mucosa extracellular matrix |
Direct 3D-bioprinting |
Preparation of in vitro airway coupled with a naturally-derived blood vessel |
270
|
— |
Collagen (type I) |
Extrusion-based (with two coaxial nozzles) |
Preparation of a 3D vascular network |
271
|
Stereolithography resin |
GelMA |
Inkjet-based |
Study of cancer cells in a co-cultured microfluidic platform |
272
|
Glass |
Gelatin fibrinogen |
Extrusion-based (with four nozzles) |
Preparation of thick vascularized tissues through the printing of bone marrow and neonatal dermal fibroblasts stem cells |
273
|
4D-bioprinting, as an updated version of 3D-bioprinting, imparts dynamic behavior to the bioprinted scaffolds under external stimuli like water, light, temperature, pH, biological activity, or magnetic and electric fields, by adding time as the fourth dimension.274 4D bioprinting has already been applied in many biomedicine fields like biosensors, bioactuators and biorobotics, and tissue engineering, and so far, many attempts have been made at 4D bioprinting of bio-inks based on various polymeric complexes.275,276 Of course, considering the possible effects of a dynamic reshaping of 4D scaffolds on cell viability and proliferation, the combination of smart cell-laden bio-inks composed of natural polymers like chitosan with nanoparticles could result in the preparation of complex dynamic tissue constructs as a future perspective in medical applications of 4D bioprinting.277 In this regard, drug delivery, tissue engineering, cancer therapy, and wastewater purification using 4D printed grafts could be considered as a new application perspective of chitosan-based biomaterials. Moreover, there is enthusiasm and a need for the synthesis of rapidly self-healing scaffolds for a wide range of requests in biomedicine due to their extreme mechanical/physical stabilities and their long lifetime after induction of structural wounds.88 The characteristic of self-healing constructs is that they sense external alterations and assimilate to them by changing their features and how they work. It is a proven fact that this wonderful characteristic of a scaffold enormously depends on the mechanical robustness of the precursors.278 According to previous reports, prepared chitosan-based self-healing constructs could not completely fulfill the mechanical strength requirements for the biomedical engineering industries. However, many different mechanisms and procedures, such as adding magnetic and conductive nanoparticles to their composition, have been applied to increase the self-healing and mechanical properties.279–282 Lately, rapid, room temperature conductive chitosan-based frameworks have been prepared for biomedical engineering fields.228
9. Commercialization
Fortunately, the commercialization of chitosan polymers with a broad range of deacetylation degrees and molecular weights paves the way for a wide range of requirements, including regenerative medicine and biomedical engineering. Although the application of chitosan for wound healing and dietary demands has been approved by the FDA and some European regulatory agencies, it has not succeeded yet in gaining FDA approval for cancer therapy, gene delivery, and drug delivery uses.283,284 Unique features of chitosan, including nontoxicity, affordability (abundancy and cost-efficient factors), excellent bioactivity and mechanical properties drives researchers to keep using of chitosan in cancer research and delivery systems. Accordingly, many published papers and patented chitosan-based materials have been reported.190 The introduction of an injectable pre-gelled paste, called BST-Gel®, based on a chitosan/mineral composite, for cartilage tissue repair is a case in point.285 Reaxon®, SlimMED™, and ChitoDot® are other examples of commercial chitosan-based patented products that are applicable for different functionalities from peripheral nerve repair to treatment of overweight and antibacterial hemostatic dressings to stop bleeding, respectively.286–288 Lately, a new methacrylate chitosan-based ink was invented to prepare a biodegradable tissue 3D construct utilized as a quick healing agent for small to large bone fractures.289,292 So far, many patented chitosan-based inks/bio-inks for different functionalities have been manufactured all around the world, giving new hope for the fast-burgeoning commercialized chitosan-based 3D scaffolds for biomedical applications.290,291
10. Conclusions
Conventional 3D scaffolds such as gels and fibrous matrices applied in tissue regeneration have failed to recapitulate native tissues with high exactness mostly due to suffering from a non-uniform distribution of structural pores, which restricts the ease of oxygen penetration, exchange of food and wastes. On the other hand, 3D bioprinting as a low-cost technique can offer facile preparation of sophisticated human organs and tissues holding vascular systems, neural networks, or osteochondral tissue with high resolution, anatomical similarities, and functional dynamics identical to the real tissues. Among all suitable materials for bio-inks (e.g., combinations of various synthetic and biopolymers along with a wide array of microcarriers and nanoparticles), chitosan-based bio-inks as biodegradable and affordable inks with an elevated degree of printability, cytocompatibility, biocompatibility, cell attachment/proliferation/migration, antimicrobial activity, and mechanical stability have been captured a lot of attentions. Chitosan-based bio-inks have been applied to prepare high-resolution 3D patterns with tailorable pore volume size, interconnected lamellae, and the capability to support the lineage commitment of encapsulated cells for utilization in bone, cartilage, vascular systems, neural networks, and skin tissue regeneration. Despite the fact that many challenges still remain in the use of chitosan for creating more complex 3D constructs, mainly due to chitosan's nature and the restrictions in currently existing bioprinting techniques. A plethora of attempts have been made to improve the printability of chitosan-based bio-inks, and, finally, to gain FDA approval for the utilization of these 3D-printed scaffolds in tissue engineering and drug delivery demands. Furthermore, chitosan-based 3D-printed structures exhibited extreme potential to take up high amounts of hazardous contamination such as organic pollutants and heavy metal ions from wastewater pathways and for the adsorption/desorption of hydrophilic/hydrophobic drugs by different mechanisms for environmental purification and drug delivery demands as well. Finally, in this study, the possible open door for 3D-bioprinting of chitosan for organ-on-a-chip systems in order to recapitulate complex organs containing sophisticated vascular or neural networks with high fidelity is pointed out.
Statement of contributions
A.T., M.T., and M. K. Y. equally contributed to the writing of the original manuscript. M.R.S., F.J.S., P.Z., S.H.R., J.D.R. and G.N. contributed to the search and also drafting the manuscript. M.R.S., M.M. and U.S.S. supervised the project, edited the manuscript, and approved the final version.
Conflicts of interest
The authors declare that they have no competing interests.
References
- I. Matai, G. Kaur, A. Seyedsalehi, A. McClinton and C. T. Laurencin, Progress in 3D bioprinting technology for tissue/organ regenerative engineering, Biomaterials, 2020, 226, 119536 CrossRef CAS PubMed.
- B.-J. de Gans, P. C. Duineveld and U. S. Schubert, Inkjet Printing of Polymers: State of the Art and Future Developments, Adv. Mater., 2004, 16(3), 203–213, DOI:10.1002/adma.200300385.
- E. Tekin, P. J. Smith and U. S. Schubert, Inkjet printing as a deposition and patterning tool for polymers and inorganic particles, Soft Matter, 2008, 4(4), 703–713, 10.1039/B711984D.
- S. Das and B. Basu, An Overview of Hydrogel-Based Bioinks for 3D Bioprinting of Soft Tissues, J. Indian Inst. Sci., 2019, 1–24 Search PubMed.
-
N. Ahmad, P. Gopinath and R. Dutta, 3D printing technology in nanomedicine, Elsevier, 2019 Search PubMed.
- Y. S. Zhang,
et al., Bioprinting 3D microfibrous scaffolds for engineering endothelialized myocardium and heart-on-a-chip, Biomaterials, 2016, 110, 45–59 CrossRef CAS.
- S. Knowlton, B. Yenilmez, S. Anand and S. Tasoglu, Photocrosslinking-based bioprinting: examining crosslinking schemes, Bioprinting, 2017, 5, 10–18 CrossRef.
- L. Valot, J. Martinez, A. Mehdi and G. Subra, Chemical insights into bioinks for 3D printing, Chem. Soc. Rev., 2019, 48(15), 4049–4086 RSC.
- R. Seyedmahmoud,
et al., Three-Dimensional Bioprinting of Functional Skeletal Muscle Tissue Using Gelatin Methacryloyl-Alginate Bioinks, Micromachines, 2019, 10(10), 679 CrossRef.
- M. Hospodiuk, M. Dey, D. Sosnoski and I. T. Ozbolat, The bioink: a comprehensive review on bioprintable materials, Biotechnol. Adv., 2017, 35(2), 217–239 CrossRef CAS PubMed.
- S. V. Murphy, A. Skardal and A. Atala, Evaluation of hydrogels for bio–printing applications, J. Biomed. Mater. Res., Part A, 2013, 101(1), 272–284 CrossRef PubMed.
- K. Karzyński,
et al., Use of 3D bioprinting in biomedical engineering for clinical application, Med. Stud./Stud. Med., 2018, 34(1), 93–97 CrossRef.
-
T. J. Kean and M. Thanou, Utility of chitosan for 3D Printing and Bioprinting, in Sustainable Agriculture Reviews 35, Springer, 2019, pp. 271–292 Search PubMed.
- B. Duan, State-of-the-art review of 3D bioprinting for cardiovascular tissue engineering, Ann. Biomed. Eng., 2017, 45(1), 195–209 CrossRef.
- O. R. Young, F. Berkhout, G. C. Gallopin, M. A. Janssen, E. Ostrom and S. Van der Leeuw, The globalization of socio-ecological systems: an agenda for scientific research, Global Environ. Change, 2006, 16(3), 304–316 CrossRef.
- R. F. Pereira and P. J. Bártolo, 3D bioprinting of photocrosslinkable hydrogel constructs, J. Appl. Polym. Sci., 2015, 132(48), 42458 CrossRef.
- X. Wang,
et al., 3D bioprinting technologies for hard tissue and organ engineering, Materials, 2016, 9(10), 802 CrossRef.
- M. Shabnam,
et al., Chitosan in Biomedical Engineering: A Critical Review, Curr. Stem Cell Res. Ther., 2019, 14(2), 93–116, DOI:10.2174/1574888X13666180912142028.
- S. Manouchehri,
et al., Electroactive bio-epoxy incorporated chitosan-oligoaniline as an advanced hydrogel coating for neural interfaces, Prog. Org. Coat., 2019, 131, 389–396 CrossRef CAS.
- B. Bagheri,
et al., Self-gelling electroactive hydrogels based on chitosan–aniline oligomers/agarose for neural tissue engineering with on-demand drug release, Colloids Surf., B, 2019, 184, 110549 CrossRef CAS PubMed.
- M. A. Salati,
et al., Agarose-Based Biomaterials: Opportunities and Challenges in Cartilage Tissue Engineering, Polymers, 2020, 12(5), 1150 CrossRef CAS PubMed.
- M. K. Yazdi,
et al., Agarose-based biomaterials for advanced drug delivery, J. Controlled Release, 2020, 523–543 CrossRef.
- P. Zarrintaj,
et al., Agarose-based biomaterials for tissue engineering, Carbohydr. Polym., 2018, 187, 66–84 CrossRef CAS PubMed.
- Y. Sun, Y. You, W. Jiang, Q. Wu, B. Wang and K. Dai, Generating ready-to-implant anisotropic menisci by 3D-bioprinting protein-releasing cell-laden hydrogel-polymer composite scaffold, Appl. Mater. Today, 2020, 18, 100469 CrossRef.
- M. Sahranavard, A. Zamanian, F. Ghorbani and M. H. Shahrezaee, A critical review on three dimensional-printed chitosan hydrogels for development of tissue engineering, Bioprinting, 2020, 17, e00063 CrossRef.
- B. Bagheri,
et al., Tissue engineering with electrospun electro-responsive chitosan-aniline oligomer/polyvinyl alcohol, Int. J. Biol. Macromol., 2020, 147, 160–169 CrossRef CAS.
- G. Mahmodi,
et al., From microporous to mesoporous mineral frameworks: An alliance between zeolite and chitosan, Carbohydr. Res., 2020, 489, 107930 CrossRef PubMed.
-
S. Tarassoli, Z. Jessop, S. Kyle and I. Whitaker, Candidate bioinks for 3D bioprinting soft tissue, in 3D Bioprinting for Reconstructive Surgery, Elsevier, 2018, pp. 145–172 Search PubMed.
- C. Liu,
et al., Bioprinted chitosan and hydroxyapatite micro-channels structures scaffold for vascularization of bone regeneration, J. Biomater. Tissue Eng., 2017, 7(1), 28–34 CrossRef.
- T. T. Demirtaş, G. Irmak and M. Gümüşderelioğlu, A bioprintable form of chitosan hydrogel for bone tissue engineering, Biofabrication, 2017, 9(3), 035003 CrossRef.
- V. B. Morris, S. Nimbalkar, M. Younesi, P. McClellan and O. Akkus, Mechanical properties, cytocompatibility and manufacturability of chitosan: PEGDA hybrid-gel scaffolds by stereolithography, Ann. Biomed. Eng., 2017, 45(1), 286–296 CrossRef.
- C. R. Almeida, T. Serra, M. I. Oliveira, J. A. Planell, M. A. Barbosa and M. Navarro, Impact of 3-D printed PLA-and chitosan-based scaffolds on human monocyte/macrophage responses: unraveling the effect of 3-D structures on inflammation, Acta Biomater., 2014, 10(2), 613–622 CrossRef CAS.
-
T. Akopova, et al., Solid state synthesis of chitosan and its unsaturated derivatives for laser microfabrication of 3D scaffolds, in IOP Conf. Ser. Mater. Sci. Eng., 2015, vol. 87, p. 012079 Search PubMed.
- Q. Wu, M. Maire, S. Lerouge, D. Therriault and M. C. Heuzey, 3D printing of microstructured and stretchable chitosan hydrogel for guided cell growth, Adv. Biosyst., 2017, 1(6), 1700058 CrossRef.
- R. Gauvin,
et al., Microfabrication of complex porous tissue engineering scaffolds using 3D projection stereolithography, Biomaterials, 2012, 33(15), 3824–3834 CrossRef CAS.
- D. Chimene, K. K. Lennox, R. R. Kaunas and A. K. Gaharwar, Advanced bioinks for 3D printing: a materials science perspective, Ann. Biomed. Eng., 2016, 44(6), 2090–2102 CrossRef.
- P. Koti, N. Muselimyan, E. Mirdamadi, H. Asfour and N. A. Sarvazyan, Use of GelMA for 3D printing of cardiac myocytes and fibroblasts, J. 3D Print. Med., 2019, 3(1), 11–22 CrossRef CAS.
- M. Guvendiren, J. Molde, R. M. Soares and J. Kohn, Designing biomaterials for 3D printing, ACS Biomater. Sci. Eng., 2016, 2(10), 1679–1693 CrossRef CAS PubMed.
- E. Axpe and M. L. Oyen, Applications of alginate-based bioinks in 3D bioprinting, Int. J. Mol. Sci., 2016, 17(12), 1976 CrossRef.
- A. Tabriz, M. Hermida, N. Leslie and W. Shu, Three-dimensional bioprinting of complex cell laden alginate hydrogel structures, Biofabrication, 2015, 7, 45012 CrossRef.
- S. Khalil and W. Sun, Bioprinting endothelial cells with alginate for 3D tissue constructs, J. Biomech. Eng., 2009, 131(11), 111002 CrossRef PubMed.
- S. Das,
et al., Bioprintable, cell-laden silk fibroin–gelatin hydrogel supporting multilineage differentiation of stem cells for fabrication of three-dimensional tissue constructs, Acta Biomater., 2015, 11, 233–246 CrossRef CAS PubMed.
- S. Chawla, A. Kumar, P. Admane, A. Bandyopadhyay and S. Ghosh, Elucidating role of silk-gelatin bioink to recapitulate articular cartilage differentiation in 3D
bioprinted constructs, Bioprinting, 2017, 7, 1–13 CrossRef.
- D. F. Duarte Campos,
et al., The stiffness and structure of three-dimensional printed hydrogels direct the differentiation of mesenchymal stromal cells toward adipogenic and osteogenic lineages, Tissue Eng., Part A, 2015, 21(3–4), 740–756 CrossRef CAS.
- Z. Zheng,
et al., 3D bioprinting of self–standing silk–based bioink, Adv. Healthcare Mater., 2018, 7(6), 1701026 CrossRef.
- J. B. Costa, J. Silva-Correia, J. M. Oliveira and R. L. Reis, Fast setting silk fibroin bioink for bioprinting of patient–specific memory–shape implants, Adv. Healthcare Mater., 2017, 6(22), 1701021 CrossRef PubMed.
- C. M. Smith,
et al., Three-dimensional bioassembly tool for generating viable tissue-engineered constructs, Tissue Eng., 2004, 10(9–10), 1566–1576 CrossRef CAS.
- M. Müller, J. Becher, M. Schnabelrauch and M. Zenobi-Wong, Nanostructured Pluronic hydrogels as bioinks for 3D bioprinting, Biofabrication, 2015, 7(3), 035006 CrossRef.
- A. P. Haring,
et al., Process-and bio-inspired hydrogels for 3D bioprinting of soft free-standing neural and glial tissues, Biofabrication, 2019, 11(2), 025009 CrossRef CAS PubMed.
- Y. Zhao,
et al., Three-dimensional printing of Hela cells for cervical tumor model in vitro, Biofabrication, 2014, 6(3), 035001 CrossRef CAS PubMed.
- C. Norotte, F. S. Marga, L. E. Niklason and G. Forgacs, Scaffold-free vascular tissue engineering using bioprinting, Biomaterials, 2009, 30(30), 5910–5917 CrossRef CAS.
- T. Xu, C. A. Gregory, P. Molnar, X. Cui, S. Jalota, S. B. Bhaduri and T. Boland, Viability and electrophysiology of neural cell structures generated by the inkjet printing method, Biomaterials, 2006a, 27, 3580–3588 Search PubMed.
- T. A. Ahmed, E. V. Dare and M. Hincke, Fibrin: a versatile scaffold for tissue engineering applications, Tissue Eng., Part B, 2008, 14(2), 199–215 CrossRef CAS PubMed.
- A. Skardal, J. Zhang and G. D. Prestwich, Bioprinting vessel-like constructs using hyaluronan hydrogels crosslinked with tetrahedral polyethylene glycol tetracrylates, Biomaterials, 2010, 31(24), 6173–6181 CrossRef CAS PubMed.
- L. Pescosolido,
et al., Hyaluronic acid and dextran-based semi-IPN hydrogels as biomaterials for bioprinting, Biomacromolecules, 2011, 12(5), 1831–1838 CrossRef CAS PubMed.
- L. Hockaday,
et al., Rapid 3D printing of anatomically accurate and mechanically heterogeneous aortic valve hydrogel scaffolds, Biofabrication, 2012, 4(3), 035005 CrossRef CAS PubMed.
- X. Cui, K. Breitenkamp, M. Finn, M. Lotz and D. D. D'Lima, Direct human cartilage repair using three-dimensional bioprinting technology, Tissue Eng., Part A, 2012, 18(11–12), 1304–1312 CrossRef CAS.
- I. T. Ozbolat, Scaffold-based or scaffold-free bioprinting: competing or complementing approaches?, J. Nanotechnol. Eng. Med., 2015, 6(2), 024701 CrossRef.
- H. Su, C.-A. H. Price, L. Jing, Q. Tian, J. Liu and K. Qian, Janus particles: design, preparation, and biomedical applications, Mater. Today Bio, 2019, 4, 100033 CrossRef CAS PubMed.
- Q. Wu, D. Therriault and M.-C. Heuzey, Processing and properties of chitosan inks for 3D printing of hydrogel microstructures, ACS Biomater. Sci. Eng., 2018, 4(7), 2643–2652 CrossRef CAS.
-
C. B. Highley, 3D Bioprinting Technologies, in 3D Bioprinting in Medicine, Springer, 2019, pp. 1–66 Search PubMed.
-
D. Tan, A. Nokhodchi and M. Maniruzzaman, 3d and 4d printing technologies: innovative process engineering and smart additive manufacturing, in 3D and 4D Printing in Biomedical Applications: Process Engineering and Additive Manufacturing, 2019, pp. 25–52 Search PubMed.
- J. Jang, J. Y. Park, G. Gao and D.-W. Cho, Biomaterials-based 3D cell printing for next-generation therapeutics and diagnostics, Biomaterials, 2018, 156, 88–106 CrossRef CAS PubMed.
- N. Ashammakhi,
et al., Advancing frontiers in bone bioprinting, Adv. Healthcare Mater., 2019, 8(7), 1801048 CrossRef PubMed.
- G. Gao and X. Cui, Three-dimensional bioprinting in tissue engineering and regenerative medicine, Biotechnol. Lett., 2016, 38(2), 203–211 CrossRef CAS PubMed.
- X. Cui, D. Dean, Z. M. Ruggeri and T. Boland, Cell damage evaluation of thermal inkjet printed Chinese hamster ovary cells, Biotechnol. Bioeng., 2010, 106(6), 963–969 CrossRef CAS.
- Z. Galliger, C. D. Vogt and A. Panoskaltsis-Mortari, 3D bioprinting for lungs and hollow organs, Transl. Res., 2019, 211, 19–34 CrossRef.
-
L. Ouyang, Study on Microextrusion-based 3D Bioprinting and Bioink Crosslinking Mechanisms, Springer, 2019 Search PubMed.
- H.-G. Yi, H. Lee and D.-W. Cho, 3D printing of organs-on-chips, Bioengineering, 2017, 4(1), 10 CrossRef PubMed.
- S. V. Murphy and A. Atala, 3D bioprinting of tissues and organs, Nat. Biotechnol., 2014, 32(8), 773–785 CrossRef CAS.
- Y.-J. Choi, H.-G. Yi, S.-W. Kim and D.-W. Cho, 3D cell printed tissue analogues: a new platform for theranostics, Theranostics, 2017, 7(12), 3118 CrossRef CAS.
- H. Cui, M. Nowicki, J. P. Fisher and L. G. Zhang, 3D bioprinting for organ regeneration, Adv. Healthcare Mater., 2017, 6(1), 1601118 CrossRef PubMed.
- N. Bhattacharjee, A. Urrios, S. Kang and A. Folch, The upcoming 3D-printing revolution in microfluidics, Lab Chip, 2016, 16(10), 1720–1742 RSC.
- G. Cidonio,
et al., Osteogenic and angiogenic tissue formation in high fidelity nanocomposite Laponite-gelatin bioinks, Biofabrication, 2019, 11(3), 035027 CrossRef CAS.
- Y. P. Singh, A. Bandyopadhyay and B. B. Mandal, 3D Bioprinting Using Cross-Linker-Free Silk–Gelatin Bioink for Cartilage Tissue Engineering, ACS Appl. Mater. Interfaces, 2019, 11(37), 33684–33696 CrossRef CAS.
- A. Mazzocchi, M. Devarasetty, R. Huntwork, S. Soker and A. Skardal, Optimization of collagen type I-hyaluronan hybrid bioink for 3D bioprinted liver microenvironments, Biofabrication, 2018, 11(1), 015003 CrossRef.
- O. Jeon, Y. B. Lee, T. J. Hinton, A. W. Feinberg and E. Alsberg, Cryopreserved cell-laden alginate microgel bioink for 3D bioprinting of living tissues, Mater. Today Chem., 2019, 12, 61–70 CrossRef CAS.
- J. Yin, M. Yan, Y. Wang, J. Fu and H. Suo, 3D bioprinting of low-concentration cell-laden gelatin methacrylate (GelMA) bioinks with a two-step cross-linking strategy, ACS Appl. Mater. Interfaces, 2018, 10(8), 6849–6857 CrossRef CAS.
- P. Apelgren,
et al., In vivo human cartilage formation in three-dimensional bioprinted constructs with a novel bacterial nanocellulose bioink, ACS Biomater. Sci. Eng., 2019, 5(5), 2482–2490 CrossRef CAS.
-
E. Abelardo, Synthetic material bioinks, in 3D Bioprinting for Reconstructive Surgery, Elsevier, 2018, pp. 137–144 Search PubMed.
- Z. Wu, X. Su, Y. Xu, B. Kong, W. Sun and S. Mi, Bioprinting three-dimensional cell-laden tissue constructs with controllable degradation, Sci. Rep., 2016, 6(1), 1–10 CrossRef.
- F. P. Melchels,
et al., Hydrogel-based reinforcement of 3D bioprinted constructs, Biofabrication, 2016, 8(3), 035004 CrossRef CAS PubMed.
- W. Jia,
et al., Direct 3D bioprinting of perfusable vascular constructs using a blend bioink, Biomaterials, 2016, 106, 58–68 CrossRef CAS.
- Z. Shariatinia and A. Mazloom-Jalali, Chitosan nanocomposite drug delivery systems designed for the ifosfamide anticancer drug using molecular dynamics simulations, J. Mol. Liq., 2019, 273, 346–367 CrossRef CAS.
-
B. dos Santos Rodrigues, S. Lakkadwala, D. Sharma and J. Singh, Chitosan for gene, DNA vaccines, and drug delivery, in Materials for Biomedical Engineering, Elsevier, 2019, pp. 515–550 Search PubMed.
- H. Guan, D. Gong, Y. Song, B. Han and N. Zhang, Biosensor composed of integrated glucose oxidase with liposome microreactors/chitosan nanocomposite for amperometric glucose sensing, Colloids Surf., A, 2019, 574, 260–267 CrossRef CAS.
- A. Sadeghi, F. Moztarzadeh and J. A. Mohandesi, Investigating the effect of chitosan on hydrophilicity and bioactivity of conductive electrospun composite scaffold for neural tissue engineering, Int. J. Biol. Macromol., 2019, 121, 625–632 CrossRef CAS PubMed.
- X. Zhang,
et al., Dual-responsive nanoparticles based on chitosan for enhanced breast cancer therapy, Carbohydr. Polym., 2019, 221, 84–93 CrossRef CAS.
- S. Niu,
et al., A novel chitosan-based nanomedicine for multi-drug resistant breast cancer therapy, Chem. Eng. J., 2019, 369, 134–149 CrossRef CAS.
- M. Ul-Islam,
et al., Development of three-dimensional bacterial cellulose/chitosan scaffolds: Analysis of cell-scaffold interaction for potential application in the diagnosis of ovarian cancer, Int. J. Biol. Macromol., 2019, 137, 1050–1059 CrossRef CAS.
- V. Medawar-Aguilar,
et al., Serological diagnosis of Toxoplasmosis disease using a fluorescent immunosensor with chitosan-ZnO-nanoparticles, Anal. Biochem., 2019, 564, 116–122 CrossRef PubMed.
- J. Groll,
et al., Biofabrication: reappraising the definition of an evolving field, Biofabrication, 2016, 8(1), 013001 CrossRef PubMed.
- S. Derakhshanfar, R. Mbeleck, K. Xu, X. Zhang, W. Zhong and M. Xing, 3D bioprinting for biomedical devices and tissue engineering: A review of recent trends and advances, Bioact. Mater., 2018, 3(2), 144–156 CrossRef PubMed.
- A. Mazzocchi, S. Soker and A. Skardal, 3D bioprinting for high-throughput screening: Drug screening, disease modeling, and precision medicine applications, Appl. Phys. Rev., 2019, 6(1), 011302 Search PubMed.
- J. Gopinathan and I. Noh, Recent trends in bioinks for 3D printing, Biomater. Res., 2018, 22(1), 11 CrossRef.
-
R. Goy, D. de Britto and O. Assis, A review of the antimicrobial activity of chitosan, PolÌmer CiÍnc, ed: Tecnol, 2009 Search PubMed.
- G. Cruz,
et al., Antimicrobial activity of chitosan composites against bacterial strains isolated from goat meat and cheese, J. Pharm. Health Care Sci., 2019, 1173(1), 012005 CAS.
- J. Huang,
et al., BMSCs-laden gelatin/sodium alginate/carboxymethyl chitosan hydrogel for 3D bioprinting, RSC Adv., 2016, 6(110), 108423–108430 RSC.
- D. Chimene, R. Kaunas and A. K. Gaharwar, Hydrogel bioink reinforcement for additive manufacturing: a focused review of emerging strategies, Adv. Mater., 2020, 32(1), 1902026 CrossRef CAS PubMed.
- Y. Wang, J. Qian, N. Zhao, T. Liu, W. Xu and A. Suo, Novel hydroxyethyl chitosan/cellulose scaffolds with bubble-like porous structure for bone tissue engineering, Carbohydr. Polym., 2017, 167, 44–51 CrossRef CAS PubMed.
- S. Bose, S. Vahabzadeh and A. Bandyopadhyay, Bone tissue engineering using 3D printing, Mater. Today, 2013, 16(12), 496–504 CrossRef CAS.
- M. A. Aronow, L. C. Gerstenfeld, T. A. Owen, M. S. Tassinari, G. S. Stein and J. B. Lian, Factors that promote progressive development of the osteoblast phenotype in cultured fetal rat calvaria cells, J. Cell. Physiol., 1990, 143(2), 213–221 CrossRef CAS PubMed.
- Y. Huang, S. Onyeri, M. Siewe and A. Moshfeghian, In vitro characterization of chitosan–gelatin scaffolds for tissue engineering, Biomaterials, 2005, 7616–7627 CrossRef CAS.
- S. Muench,
et al., Printable ionic liquid-based gel polymer electrolytes for solid state all-organic batteries, Energy Storage Mater., 2020, 25, 750–755 CrossRef.
- W. Thein-Han, J. Saikhun, C. Pholpramoo, R. Misra and Y. Kitiyanant, Chitosan–gelatin scaffolds for tissue engineering: Physico-chemical properties and biological response of buffalo embryonic stem cells and transfectant of GFP–buffalo embryonic stem cells, Acta Biomater., 2009, 5(9), 3453–3466 CrossRef CAS PubMed.
- J. Wang,
et al., Surface entrapment of chitosan on 3D printed polylactic acid scaffold and its biomimetic growth of hydroxyapatite, Compos. Interfaces, 2019, 26(5), 465–478 CrossRef CAS.
- A. Blaeser, D. F. Duarte Campos, U. Puster, W. Richtering, M. M. Stevens and H. Fischer, Controlling shear stress in 3D bioprinting is a key factor to balance printing resolution and stem cell integrity, Adv. Healthcare Mater., 2016, 5(3), 326–333 CrossRef CAS.
- T. Billiet, B. V. Gasse, E. Gevaert, M. Cornelissen, J. C. Martins and P. Dubruel, Quantitative Contrasts in the Photopolymerization of Acrylamide and Methacrylamide–Functionalized Gelatin Hydrogel Building Blocks, Macromol. Biosci., 2013, 13(11), 1531–1545 CrossRef CAS PubMed.
- P. Chavanne,
et al., 3D printed chitosan/hydroxyapatite scaffolds for potential use in regenerative medicine, Biomed. Eng./Biomed. Tech., 2013, 58(SI-1-Track-C), 4069 Search PubMed.
- L. T. Saldin, M. C. Cramer, S. S. Velankar, L. J. White and S. F. Badylak, Extracellular matrix hydrogels from decellularized tissues: structure and function, Acta Biomater., 2017, 49, 1–15 CrossRef CAS PubMed.
- S. Yuan, D. Strobbe, J.-P. Kruth, P. Van Puyvelde and B. Van der Bruggen, Super-hydrophobic 3D printed polysulfone membranes with a switchable wettability by self-assembled candle soot for efficient gravity-driven oil/water separation, J. Mater. Chem. A, 2017, 5(48), 25401–25409 RSC.
- H. Montazerian,
et al., Permeability and mechanical properties of gradient porous PDMS scaffolds fabricated by 3D-printed sacrificial templates designed with minimal surfaces, Acta Biomater., 2019, 96, 149–160 CrossRef CAS PubMed.
- S. Kyle, Z. M. Jessop, A. Al-Sabah and I. S. Whitaker, Biofabrication: ‘Printability’ of Candidate Biomaterials for Extrusion Based 3D Printing: State-of-the-Art (Adv. Healthcare Mater. 16/2017), Adv. Healthcare Mater., 2017, 6(16), 1700264 CrossRef PubMed.
- E. DeSimone, K. Schacht, T. Jungst, J. Groll and T. Scheibel, Biofabrication of 3D constructs: Fabrication technologies and spider silk proteins as bioinks, Pure Appl. Chem., 2015, 87(8), 737–749 CAS.
- S. Chawla, S. Midha, A. Sharma and S. Ghosh, Silk–based bioinks for 3D bioprinting, Adv. Healthcare Mater., 2018, 7(8), 1701204 CrossRef.
- S. Van Belleghem,
et al., Hybrid 3D Printing of Synthetic and Cell–Laden Bioinks for Shape Retaining Soft Tissue Grafts, Adv. Funct. Mater., 2020, 30(3), 1907145 CrossRef CAS PubMed.
- K. D. Roehm and S. V. Madihally, Bioprinted chitosan-gelatin thermosensitive hydrogels using an inexpensive 3D printer, Biofabrication, 2017, 10(1), 015002 CrossRef.
- J. E. Trachtenberg, P. M. Mountziaris, J. S. Miller, M. Wettergreen, F. K. Kasper and A. G. Mikos, Open–source three–dimensional printing of biodegradable polymer scaffolds for tissue engineering, J. Biomed. Mater. Res., Part A, 2014, 102(12), 4326–4335 Search PubMed.
- G. D. Nicodemus and S. J. Bryant, Cell encapsulation in biodegradable hydrogels for tissue engineering applications, Tissue Eng., Part B, 2008, 14(2), 149–165 CrossRef CAS.
- M. Rinaudo, Chitin and chitosan: properties and applications, Prog. Polym. Sci., 2006, 31(7), 603–632 CrossRef CAS.
- A. Lončarević, M. Ivanković and A. Rogina, Lysozyme-induced degradation of chitosan: the characterisation of degraded chitosan scaffolds, J. Tissue Repair Regener., 2017, 1(1), 12 Search PubMed.
- D. Lee,
et al., Chitosan-catechol: a writable bioink under serum culture media, Biomater. Sci., 2018, 6(5), 1040–1047 RSC.
- C. Li,
et al., Controllable fabrication of hydroxybutyl chitosan/oxidized chondroitin sulfate hydrogels by 3D bioprinting technique for cartilage tissue engineering, Biomed. Mater., 2019, 14(2), 025006 CrossRef CAS PubMed.
- F. Hafezi, N. Scoutaris, D. Douroumis and J. Boateng, 3D printed chitosan dressing crosslinked with genipin for potential healing of chronic wounds, Int. J. Pharm., 2019, 560, 406–415 CrossRef CAS PubMed.
- P. Shi, W. Y. Yeong, A. Laude and E. Y. S. Tan, Hybrid three-dimensional (3D) bioprinting of retina equivalent for ocular research, Int. J. Bioprint., 2017, 138–146 CAS.
- C. Intini,
et al., 3D-printed chitosan-based scaffolds: An in vitro study of human skin cell growth and an in vivo wound healing evaluation in experimental diabetes in rats, Carbohydr. Polym., 2018, 199, 593–602 CrossRef CAS.
- M. Khanmohammadi, S. Sakai and M. Taya, Fabrication of single and bundled filament-like tissues using biodegradable hyaluronic acid-based hollow hydrogel fibers, Int. J. Biol. Macromol., 2017, 104, 204–212 CrossRef CAS PubMed.
- A. Parak, P. Pradeep, L. C. du Toit, P. Kumar, Y. E. Choonara and V. Pillay, Functionalizing bioinks for 3D bioprinting applications, Drug Discovery Today, 2019, 24(1), 198–205 CrossRef CAS.
- M. Yadav, P. Goswami, K. Paritosh, M. Kumar, N. Pareek and V. Vivekanand, Seafood waste: a source for preparation of commercially employable chitin/chitosan materials, Bioresour. Bioprocess., 2019, 6(1), 8 CrossRef.
- Y.-L. Cheng and F. Chen, Preparation and characterization of photocured poly (ε-caprolactone) diacrylate/poly (ethylene glycol) diacrylate/chitosan for photopolymerization-type 3D printing tissue engineering scaffold application, Mater. Sci. Eng., C, 2017, 81, 66–73 CrossRef CAS.
- W. L. Ng, W. Y. Yeong and M. W. Naing, Polyelectrolyte gelatin-chitosan hydrogel optimized for 3D bioprinting in skin tissue engineering, Int. J. Bioprint, 2016, 2(1), 53–62 CAS.
- Y. Sun,
et al., 3D printing collagen/chitosan scaffold ameliorated axon regeneration and neurological recovery after spinal cord injury, J. Biomed. Mater. Res., Part A, 2019, 107(9), 1898–1908 CrossRef CAS PubMed.
- S. Li, Z. Xiong, X. Wang, Y. Yan, H. Liu and R. Zhang, Direct fabrication of a hybrid cell/hydrogel construct by a double-nozzle assembling technology, J. Bioact. Compat. Polym., 2009, 24(3), 249–265 CrossRef CAS.
- L. Elviri,
et al., Highly defined 3D printed chitosan scaffolds featuring improved cell growth, Biomed. Mater., 2017, 12(4), 045009 CrossRef PubMed.
- W. L. Ng, W. Y. Yeong and M. W. Naing, Development of polyelectrolyte chitosan-gelatin hydrogels for skin bioprinting, Procedia CIRP, 2016, 49, 105–112 CrossRef.
- N. M. Ergul,
et al., 3D printing of chitosan/poly (vinyl alcohol) hydrogel containing synthesized hydroxyapatite scaffolds for hard-tissue engineering, Polym. Test., 2019, 79, 106006 CrossRef CAS.
- F. Fu,
et al., Magnetic resonance imaging-three-dimensional printing technology fabricates customized scaffolds for brain tissue engineering, Neural Regener. Res., 2017, 12(4), 614 CrossRef PubMed.
- X. Hu,
et al., 3D Bio-printing of CS/Gel/HA/Gr hybrid osteochondral scaffolds, Polymers, 2019, 11(10), 1601 CrossRef CAS PubMed.
- E. Vorndran, U. Klammert, A. Ewald, J. E. Barralet and U. Gbureck, Simultaneous immobilization of bioactives during 3D powder printing of bioceramic drug–release matrices, Adv. Funct. Mater., 2010, 20(10), 1585–1591 CrossRef CAS.
- C. Colosi,
et al., Rapid prototyping of chitosan-coated alginate scaffolds through the use of a 3D fiber deposition technique, J. Mater. Chem. B, 2014, 2(39), 6779–6791 RSC.
- H. Li, Y. J. Tan, S. Liu and L. Li, Three-dimensional bioprinting of oppositely charged hydrogels with super strong interface bonding, ACS Appl. Mater. Interfaces, 2018, 10(13), 11164–11174 CrossRef CAS PubMed.
- H. Chen, Y. Liu, Z. Jiang, W. Chen, Y. Yu and Q. Hu, Cell–scaffold interaction within engineered tissue, Exp. Cell Res., 2014, 323(2), 346–351 CrossRef CAS PubMed.
- C.-S. Wu, Modulation, functionality, and cytocompatibility of three-dimensional printing materials made from chitosan-based polysaccharide composites, Mater. Sci. Eng., C, 2016, 69, 27–36 CrossRef CAS PubMed.
- X. Wang, C. Wei, B. Cao, L. Jiang, Y. Hou and J. Chang, Fabrication of multiple-layered hydrogel scaffolds with elaborate structure and good mechanical properties via 3D printing and ionic reinforcement, ACS Appl. Mater. Interfaces, 2018, 10(21), 18338–18350 CrossRef CAS.
- Y. Tsukamoto, T. Akagi, F. Shima and M. Akashi, Fabrication of orientation-controlled 3D tissues using a layer-by-layer technique and 3D printed a thermoresponsive gel frame, Tissue Eng., Part C, 2017, 23(6), 357–366 CrossRef CAS PubMed.
-
K. Bardakova, et al., 3D printing biodegradable scaffolds with chitosan materials for tissue engineering, in IOP Conf Ser: Mater Sci Eng., 2018, vol. 347, p. 012009 Search PubMed.
- D. Fan, U. Staufer and A. Accardo, Engineered 3D Polymer and Hydrogel Microenvironments for Cell Culture Applications, Bioengineering, 2019, 6(4), 113 CrossRef CAS PubMed.
- K. Wang, F. M. Kievit, A. E. Erickson, J. R. Silber, R. G. Ellenbogen and M. Zhang, Culture on 3D chitosan–hyaluronic acid scaffolds enhances stem cell marker expression and drug resistance in human glioblastoma cancer stem cells, Adv. Healthcare Mater., 2016, 5(24), 3173–3181 CrossRef CAS PubMed.
- B. A. Lindborg,
et al., A chitosan-hyaluronan-based hydrogel-hydrocolloid supports in vitro culture and differentiation of human mesenchymal stem/stromal cells, Tissue Eng., Part A, 2015, 21(11–12), 1952–1962 CrossRef CAS PubMed.
- A. R. Akkineni, Y. Luo, M. Schumacher, B. Nies, A. Lode and M. Gelinsky, 3D plotting of growth factor loaded calcium phosphate cement scaffolds, Acta Biomater., 2015, 27, 264–274 CrossRef CAS PubMed.
- S. Nganga,
et al., Inkjet printing of Chitlac-nanosilver—a method to create functional coatings for non-metallic bone implants, Biofabrication, 2014, 6(4), 041001 CrossRef CAS PubMed.
- J. Jung, K. Kim, S. C. Choi and J. Oh, Microfluidics-assisted rapid generation of tubular cell-laden microgel inside glass capillaries, Biotechnol. Lett., 2014, 36(7), 1549–1554 CrossRef CAS PubMed.
- S. Chen, Y. Shi, Y. Luo and J. Ma, Layer-by-layer coated porous 3D printed hydroxyapatite composite scaffolds for controlled drug delivery, Colloids Surf., B, 2019, 179, 121–127 CrossRef CAS PubMed.
- W. E. Müller,
et al., A new printable and durable N, O-carboxymethyl chitosan–Ca2+–polyphosphate complex with morphogenetic activity, J. Mater. Chem. B, 2015, 3(8), 1722–1730 RSC.
- Q. Gu,
et al., Functional 3D neural mini–tissues from printed gel–based bioink and human neural stem cells, Adv. Healthcare Mater., 2016, 5(12), 1429–1438 CrossRef CAS PubMed.
- Y. Wu,
et al., Fabrication and evaluation of electrohydrodynamic jet 3D printed polycaprolactone/chitosan cell carriers using human embryonic stem cell-derived fibroblasts, J. Biomater. Appl., 2016, 31(2), 181–192 CrossRef CAS PubMed.
- M. Osorio, E. Martinez, T. Naranjo and C. Castro, Recent Advances in Polymer Nanomaterials for Drug Delivery of Adjuvants in Colorectal Cancer Treatment: A Scientific-Technological Analysis and Review, Molecules, 2020, 25(10), 2270 CrossRef CAS PubMed.
- K. Haberstroh,
et al., Bone repair by cell–seeded 3D–bioplotted composite scaffolds made of collagen treated tricalciumphosphate or tricalciumphosphate–chitosan–collagen hydrogel or PLGA in ovine critical–sized calvarial defects, J. Biomed. Mater. Res., Part B, 2010, 93(2), 520–530 CrossRef PubMed.
- P. Dadhich,
et al., A simple approach for an eggshell-based 3D-printed osteoinductive multiphasic calcium phosphate scaffold, ACS Appl. Mater. Interfaces, 2016, 8(19), 11910–11924 CrossRef CAS PubMed.
- S. W. Kim, D. Y. Kim, H. H. Roh, H. S. Kim, J. W. Lee and K. Y. Lee, Three-dimensional bioprinting of cell-laden constructs using polysaccharide-based self-healing hydrogels, Biomacromolecules, 2019, 20(5), 1860–1866 CrossRef CAS PubMed.
- N. J. Vickers, Animal communication: when I'm calling you, will you answer too?, Curr. Biol., 2017, 27(14), R713–R715 CrossRef CAS PubMed.
- Y. Yang,
et al., Dual-functional 3D-printed composite scaffold for inhibiting bacterial infection and promoting bone regeneration in infected bone defect models, Acta Biomater., 2018, 79, 265–275 CrossRef CAS PubMed.
- G. Crini, Historical review on chitin and chitosan biopolymers, Environ. Chem. Lett., 2019, 1–21 Search PubMed.
-
M. Thanou, et al., Renewable resources for functional polymers and biomaterials: polysaccharides, proteins and polyesters, Royal Society of Chemistry, 2011 Search PubMed.
- C. Rouget, Des substances amylacées dans les tissus des animaux, spécialement des Articulés (chitine), Comp. Rend., 1859, 48, 792–795 Search PubMed.
- F. Croisier and C. Jérôme, Chitosan-based biomaterials for tissue engineering, Eur. Polym. J., 2013, 49(4), 780–792 CrossRef CAS.
- N. M. Mahmoodi, M. Oveisi, A. Taghizadeh and M. Taghizadeh, Synthesis of pearl necklace-like ZIF-8@chitosan/PVA nanofiber with synergistic effect for recycling aqueous dye removal, Carbohydr. Polym., 2020, 227 DOI:10.1016/j.carbpol.2019.115364.
- G. Mahmodi, P. Zarrintaj, A. Taghizadeh, M. Taghizadeh, S. Manouchehri, S. Dangwal, A. Ronte, M. R. Ganjali, J. D. Ramsey and S.-J. Kim, From microporous to mesoporous mineral frameworks: An alliance between zeolite and chitosan, Carbohydr. Res., 2020, 489 DOI:10.1016/j.carres.2020.107930.
-
N. Morin-Crini, E. Lichtfouse, G. Torri and G. Crini, Fundamentals and applications of chitosan, in Sustainable Agriculture Reviews 35, Springer, 2019, pp. 49–123 Search PubMed.
-
S. Ahmed and S. Ikram, Chitosan: derivatives, composites and applications, John Wiley & Sons, 2017 Search PubMed.
- J. C. Roy, F. Salaün, S. Giraud, A. Ferri, G. Chen and J. Guan, Solubility of chitin: solvents, solution behaviors and their related mechanisms, Solubility Polysaccharides, 2017, 10, 110–130 Search PubMed.
- A. Tirella, A. Orsini, G. Vozzi and A. Ahluwalia, A phase diagram for microfabrication of geometrically controlled hydrogel scaffolds, Biofabrication, 2009, 1(4), 045002 CrossRef CAS PubMed.
- F. Ganji and M. Abdekhodaie, A.-Ramazani SA J Sol—Gel, Sci. Technol., 2007, 42, 47–53 CAS.
- R. R. Jose, M. J. Rodriguez, T. A. Dixon, F. Omenetto and D. L. Kaplan, Evolution of bioinks and additive manufacturing technologies for 3D bioprinting, ACS Biomater. Sci. Eng., 2016, 2(10), 1662–1678 CrossRef CAS PubMed.
-
B. Lim, C. Poh, C. Voon and H. Salmah, Rheological and thermal study of chitosan filled thermoplastic elastomer composites, in Applied Mechanics and Materials, Trans Tech Publ, 2015, vol. 754, pp. 34–38 Search PubMed.
- A. Kohut, A. Voronov and W. Peukert, An effective way to stabilize colloidal particles dispersed in polar and nonpolar media, Langmuir, 2007, 23(2), 504–508 CrossRef CAS PubMed.
- Y. Zhang, Y. Yu and I. T. Ozbolat, Direct bioprinting of vessel-like tubular microfluidic channels, J. Nanotechnol. Eng. Med., 2013, 4(2), 0210011–0210017 Search PubMed.
- J. Zhang,
et al., 3D printing of silk particle-reinforced chitosan hydrogel structures and their properties, ACS Biomater. Sci. Eng., 2018, 4(8), 3036–3046 CrossRef CAS PubMed.
- C. Marques, G. Diogo, S. Pina, J. M. Oliveira, T. Silva and R. Reis, Collagen-based bioinks for hard tissue engineering applications: a comprehensive review, J. Mater. Sci.: Mater. Med., 2019, 30(3), 1–12 CrossRef CAS PubMed.
- R. Chang, J. Nam and W. Sun, Direct cell writing of 3D microorgan for in vitro pharmacokinetic model, Tissue Eng., Part C, 2008, 14(2), 157–166 CrossRef CAS PubMed.
- S. Khalil and W. Sun, Biopolymer deposition for freeform fabrication of hydrogel tissue constructs, Mater. Sci. Eng., C, 2007, 27(3), 469–478 CrossRef CAS.
- P. S. Gungor-Ozkerim, I. Inci, Y. S. Zhang, A. Khademhosseini and M. R. Dokmeci, Bioinks for 3D bioprinting: an overview, Biomater. Sci., 2018, 6(5), 915–946 RSC.
- C. Christou, K. Philippou, T. Krasia-Christoforou and I. Pashalidis, Uranium adsorption by polyvinylpyrrolidone/chitosan blended nanofibers, Carbohydr. Polym., 2019, 219, 298–305 CrossRef CAS PubMed.
- J. Hu,
et al., Electroactive aniline pentamer cross-linking chitosan for stimulation growth of electrically sensitive cells, Biomacromolecules, 2008, 9(10), 2637–2644 CrossRef CAS PubMed.
- D. Han and L. Yan, Supramolecular Hydrogel of Chitosan in the Presence of Graphene Oxide Nanosheets as 2D Cross-Linkers, ACS Sustainable Chem. Eng., 2014, 2(2), 296–300 CrossRef CAS.
- C. A. Murphy, J. B. Costa, J. Silva-Correia, J. M. Oliveira, R. L. Reis and M. N. Collins, Biopolymers and polymers in the search of alternative treatments for meniscal regeneration: state of the art and future trends, Appl. Mater. Today, 2018, 12, 51–71 CrossRef.
- M. Nourbakhsh,
et al., Fabricating an electroactive injectable hydrogel based on pluronic-chitosan/aniline-pentamer containing angiogenic factor for functional repair of the hippocampus ischemia rat model, Mater. Sci. Eng., C, 2020, 117, 111328 CrossRef CAS PubMed.
- N. Islam, H. Wang, F. Maqbool and V. Ferro, In vitro enzymatic digestibility of glutaraldehyde-crosslinked chitosan nanoparticles in lysozyme solution and their applicability in pulmonary drug delivery, Molecules, 2019, 24(7), 1271 CrossRef CAS PubMed.
- G. Kumar, J. Bristow, P. Smith and G. Payne, Enzymatic gelation of the natural polymer chitosan, Polymer, 2000, 41(6), 2157–2168 CrossRef CAS.
- E. Hafemann, R. Battisti, C. Marangoni and R. A. Machado, Valorization of royal palm tree agroindustrial waste by isolating cellulose nanocrystals, Carbohydr. Polym., 2019, 218, 188–198 CrossRef CAS PubMed.
- J.-P. Méricq, D. Bouyer, D. Wlodarczyk, L. Soussan and C. Faur, Modeling of enzymatic chitosan gelation: Tools for monitoring chitosan gelation and urea hydrolysis kinetics, React. Funct. Polym., 2019, 144, 104337 CrossRef.
- A. M. Heimbuck,
et al., Development of Responsive Chitosan–Genipin Hydrogels for the Treatment of Wounds, ACS Appl. Bio Mater., 2019, 2(7), 2879–2888 CrossRef CAS.
-
X. Du, 3D bio-printing review, in IOP Conf. Ser.: Mater. Sci. Eng., 2018, vol. 301, p. 012023 Search PubMed.
- S. Kyle, Z. M. Jessop, A. Al-Sabah and I. S. Whitaker, Printability’ of candidate biomaterials for extrusion based 3D printing: state–of–the–art, Adv. Healthcare Mater., 2017, 6(16), 1700264 CrossRef PubMed.
-
I. T. Ozbolat, 3D bioprinting: fundamentals, principles and applications, Academic Press, 2016 Search PubMed.
- K. Obara,
et al., Photocrosslinkable chitosan hydrogel containing fibroblast growth factor-2 stimulates wound healing in healing-impaired db/db mice, Biomaterials, 2003, 24(20), 3437–3444 CrossRef CAS.
- C. D. O'Connell,
et al., Development of the Biopen: a handheld device for surgical printing of adipose stem cells at a chondral wound site, Biofabrication, 2016, 8(1), 015019 CrossRef PubMed.
- S. Duchi,
et al., Handheld co-axial bioprinting: application to in situ surgical cartilage repair, Sci. Rep., 2017, 7(1), 1–12 CrossRef CAS PubMed.
- J. Lammel-Lindemann, I. A. Dourado, J. Shanklin, C. A. Rodriguez, L. H. Catalani and D. Dean, Photocrosslinking-based 3D printing of unsaturated polyesters from isosorbide: A new material for resorbable medical devices, Bioprinting, 2020, 18, e00062 CrossRef.
- G. Choi and H. J. Cha, Recent advances in the development of nature-derived photocrosslinkable biomaterials for 3D printing in tissue engineering, Biomater. Res., 2019, 23(1), 18 CrossRef PubMed.
-
D. Thomas, Z. M. Jessop and I. S. Whitaker, 3D bioprinting for reconstructive surgery: techniques and applications, Woodhead Publishing, 2017 Search PubMed.
-
S. Kyle, Z. Jessop, S. Tarassoli, A. Al-Sabah and I. Whitaker, Assessing printability of bioinks, in 3D Bioprinting for Reconstructive Surgery, Elsevier, 2018, pp. 173–189 Search PubMed.
- A. I. Neto,
et al., Nanostructured polymeric coatings based on chitosan and dopamine–modified hyaluronic acid for biomedical applications, Small, 2014, 10(12), 2459–2469 CrossRef CAS PubMed.
- H. Ji, S. Hong and H. Lee, Bio-inspired adhesive catechol-conjugated chitosan for biomedical applications: A mini review [J], Acta Biomater., 2015, 27, 101–115 CrossRef PubMed.
- E. Park, J. Lee, K. M. Huh, S. H. Lee and H. Lee, Toxicity–Attenuated Glycol Chitosan Adhesive Inspired by Mussel Adhesion Mechanisms, Adv. Healthcare
Mater., 2019, 8(14), 1900275 CrossRef PubMed.
- R. Wang,
et al., Mussel-inspired chitosan-polyurethane coatings for improving the antifouling and antibacterial properties of polyethersulfone membranes, Carbohydr. Polym., 2017, 168, 310–319 CrossRef CAS PubMed.
- E. Park,
et al., Toxicity-attenuated glycol chitosan adhesive inspired by mussel adhesion mechanisms, Adv. Healt. Mat., 2019, 8(14), 1900275 CrossRef PubMed
https://doi.org/10.1002/adhm.201900275
.
- L. Wang and J. P. Stegemann, Thermogelling chitosan and collagen composite hydrogels initiated with β-glycerophosphate for bone tissue engineering, Biomaterials, 2010, 31(14), 3976–3985 CrossRef CAS PubMed.
- J. Si, Y. Yang, X. Xing, F. Yang and P. Shan, Controlled degradable chitosan/collagen composite scaffolds for application in nerve tissue regeneration, Polym. Degrad. Stab., 2019, 166, 73–85 CrossRef CAS.
- S. Ji, E. Almeida and M. Guvendiren, 3D bioprinting of complex channels within cell-laden hydrogels, Acta Biomater., 2019, 95, 214–224 CrossRef CAS PubMed.
- A. G. Sontyana, A. P. Mathew, K.-H. Cho, S. Uthaman and I.-K. Park, Biopolymeric in situ hydrogels for tissue engineering and bioimaging applications, Tissue Eng. Regener. Med., 2018, 15(5), 575–590 CrossRef PubMed.
- A. Gilarska, J. Lewandowska-Łańcucka, W. Horak and M. Nowakowska, Collagen/chitosan/hyaluronic acid–based injectable hydrogels for tissue engineering applications–design, physicochemical and biological characterization, Colloids Surf., B, 2018, 170, 152–162 CrossRef CAS PubMed.
- C. Arakawa, R. Ng, S. Tan, S. Kim, B. Wu and M. Lee, Photopolymerizable chitosan–collagen hydrogels for bone tissue engineering, J. Tissue Eng. Regener. Med., 2017, 11(1), 164–174 CrossRef CAS PubMed.
- R. Patel,
et al., Effect of dietary advanced glycation end products on mouse liver, PLoS One, 2012, 7(4), e35143 CrossRef CAS.
- S. Ahmed, A. Ali and J. Sheikh, A review on chitosan centred scaffolds and their applications in tissue engineering, Int. J. Biol. Macromol., 2018, 116, 849–862 CrossRef CAS PubMed.
- K. Ye, R. Felimban, k. Traianedes, S. E. Moulton, G. G. Wallace, J. Chung, A. Quigley, P. F. Choong and D. E. Myers, Chondrogenesis of infrapatellar fat pad derived adipose stem cells in 3D printed chitosan scaffold, PloS one, 2014, 9(6) DOI:10.1371/journal.pone.0099410.
- S. Reed, G. Lau, B. Delattre, D. D. Lopez, A. P. Tomsia and B. M. Wu, Macro-and micro-designed chitosan-alginate scaffold architecture by three-dimensional printing and directional freezing, Biofabrication, 2016, 8(1), 015003 CrossRef PubMed.
- H. Ueno, M. Murakami, M. Okumura, T. Kadosawa, T. Uede and T. Fujinaga, Chitosan accelerates the production of osteopontin from polymorphonuclear leukocytes, Biomaterials, 2001, 22(12), 1667–1673 CrossRef CAS PubMed.
- A. Rasool, S. Ata and A. Islam, Stimuli responsive biopolymer (chitosan) based blend hydrogels for wound healing application, Carbohydr. Polym., 2019, 203, 423–429 CrossRef CAS PubMed.
- J. Long, A. E. Etxeberria, A. V. Nand, C. R. Bunt, S. Ray and A. Seyfoddin, A 3D printed chitosan-pectin hydrogel wound dressing for lidocaine hydrochloride delivery, Mater. Sci. Eng., C, 2019, 104, 109873 CrossRef CAS PubMed.
-
H. Zhang, K. Yang, G. Liu, S. Zhu, R. Yin and W. Zhang, 3D bioprinting of multi-biomaterial/crosslinked bioink for skin tissue engineering, in Front. Bioeng. Biotechnol. Conference Abstract: 10th World Biomaterials Congress, 2016 Search PubMed.
- J. van Kuilenburg, M. A. Masen and E. van der Heide, Contact modelling of human skin: What value to use for the modulus of elasticity?, Proc. Inst. Mech. Eng., Part J., 2013, 227(4), 349–361 CrossRef.
- U. Bozuyuk, O. Yasa, I. C. Yasa, H. Ceylan, S. Kizilel and M. Sitti, Light-triggered drug release from 3D-printed magnetic chitosan microswimmers, ACS Nano, 2018, 12(9), 9617–9625 CrossRef CAS PubMed.
- J. Yan, Y. Wang, X. Zhang, X. Zhao, X. Ma, X. Pu, Y. Wang, F. Ran, Y. Wang and F. Leng, Snakegourd root/Astragalus polysaccharide hydrogel preparation and application in 3D printing, Int. J. Biol. Macromol., 2019, 121, 309–316 CrossRef CAS PubMed.
- M. R. Sommer, L. Alison, C. Minas, E. Tervoort, P. A. Rühs and A. R. Studart, 3D printing of concentrated emulsions into multiphase biocompatible soft materials, Soft Matter, 2017, 13(9), 1794–1803 RSC.
- T. H. Van Osch, J. Perelaer, A. W. de Laat and U. S. Schubert, Inkjet printing of narrow conductive tracks on untreated polymeric substrates, Adv. Mater., 2008, 20(2), 343–345 CrossRef CAS.
- S. Wünscher, R. Abbel, J. Perelaer and U. S. Schubert, Progress of alternative sintering approaches of inkjet-printed metal inks and their application for manufacturing of flexible electronic devices, J. Mater. Chem. C, 2014, 2(48), 10232–10261, 10.1039/C4TC01820F.
- M. A. Darabi,
et al., Skin–inspired multifunctional autonomic–intrinsic conductive self–healing hydrogels with pressure sensitivity, stretchability, and 3D printability, Adv. Mater., 2017, 29(31), 1700533 CrossRef PubMed.
- J. Xiong, Ø. Borg, E. A. Blekkan and A. Holmen, Hydrogen chemisorption on rhenium-promoted γ-alumina supported cobalt catalysts, Catal. Commun., 2008, 9(14), 2327–2330 CrossRef CAS.
- M. Rajamani and K. Rajendrakumar, Chitosan-boehmite desiccant composite as a promising adsorbent towards heavy metal removal, J. Environ. Manage., 2019, 244, 257–264 CrossRef CAS PubMed.
- G. Sargazi,
et al., Chitosan/polyvinyl alcohol nanofibrous membranes: towards green super-adsorbents for toxic gases, Heliyon, 2019, 5(4), e01527 CrossRef PubMed.
-
M. B. Kasiri, Application of chitosan derivatives as promising adsorbents for treatment of textile wastewater, in The Impact and Prospects of Green Chemistry for Textile Technology, Elsevier, 2019, pp. 417–469 Search PubMed.
- S. Sessarego, S. C. Rodrigues, Y. Xiao, Q. Lu and J. M. Hill, Phosphonium-enhanced chitosan for Cr(VI) adsorption in wastewater treatment, Carbohydr. Polym., 2019, 211, 249–256 CrossRef CAS PubMed.
- J. Desbrières and E. Guibal, Chitosan for wastewater treatment, Polym. Int., 2018, 67(1), 7–14 CrossRef.
- G. Zhou,
et al., Three-dimensional polylactic acid@ graphene oxide/chitosan sponge bionic filter: highly efficient adsorption of crystal violet dye, Int. J. Biol. Macromol., 2018, 113, 792–803 CrossRef CAS PubMed.
- J. Lee, B. Choi, B. Wu and M. Lee, Customized biomimetic scaffolds created by indirect three-dimensional printing for tissue engineering. Biofabrication, Biofabrication, 2013, 5(4), 045003 CrossRef CAS PubMed.
- H. Y. Lin, T. W. Chang and T. K. Peng, Three–dimensional plotted alginate fibers embedded with diclofenac and bone cells coated with chitosan for bone regeneration during inflammation, J. Biomed. Mater. Res., Part A, 2018, 106(6), 1511–1521 CrossRef CAS PubMed.
- M. Ainola,
et al., A bioactive hybrid three-dimensional tissue-engineering construct for cartilage repair, J. Biomater. Appl., 2016, 30(6), 873–885 CrossRef CAS PubMed.
- A. M. Handorf, Y. Zhou, M. A. Halanski and W.-J. Li, Tissue stiffness dictates development, homeostasis, and disease progression, Organogenesis, 2015, 11(1), 1–15 CrossRef PubMed.
- C.-W. Wong, Y.-T. Chen, C.-L. Chien, T.-Y. Yu, S.-P. Rwei and S.-h. Hsu, A simple and efficient feeder-free culture system to up-scale iPSCs on polymeric material surface for use in 3D bioprinting, Mater. Sci. Eng., C, 2018, 82, 69–79 CrossRef CAS PubMed.
- Y. Yang,
et al., Anti-infective efficacy, cytocompatibility and biocompatibility of a 3D-printed osteoconductive composite scaffold functionalized with quaternized chitosan, Acta Biomater., 2016, 46, 112–128 CrossRef CAS PubMed.
- C.-H. Tsai, C.-H. Hung, C.-N. Kuo, C.-Y. Chen, Y.-N. Peng and M.-Y. Shie, Improved bioactivity of 3D printed porous titanium alloy scaffold with chitosan/magnesium-calcium silicate composite for orthopaedic applications, Materials, 2019, 12(2), 203 CrossRef CAS PubMed.
- N. Noor, A. Shapira, R. Edri, I. Gal, L. Wertheim and T. Dvir, Tissue Engineering: 3D Printing of Personalized Thick and Perfusable Cardiac Patches and Hearts (Adv. Sci. 11/2019), Adv. Sci., 2019, 6(11), 1970066 CrossRef.
- G. D. Brusko, E. M. Cox and M. Y. Wang, 3-dimensional printing a novel framework for spinal cord injury repair, Neurosurgery, 2019, 85(2), E192–E193 CrossRef PubMed.
- S.-L. Ryan,
et al., Drug discovery approaches utilizing three-dimensional cell culture, Assay Drug Dev. Technol., 2016, 14(1), 19–28 CrossRef CAS PubMed.
- Q. Pi,
et al., Digitally tunable microfluidic bioprinting of multilayered cannular tissues, Adv. Mater., 2018, 30(43), 1706913 CrossRef PubMed.
- D. E. Ingber, Reverse engineering human pathophysiology with organs-on-chips, Cell, 2016, 164(6), 1105–1109 CrossRef CAS PubMed.
- Q. Yang, Q. Lian and F. Xu, Perspective: fabrication of integrated organ-on-a-chip via bioprinting, Biomicrofluidics, 2017, 11(3), 031301 CrossRef PubMed.
- K. Fetah,
et al., The emergence of 3D bioprinting in organ-on-chip systems, Prog. Biomed. Eng., 2019, 1(1), 012001 CrossRef.
- J. Ma, Y. Wang and J. Liu, Bioprinting of 3D tissues/organs combined with microfluidics, RSC Adv., 2018, 8(39), 21712–21727 RSC.
- D.-H. T. Nguyen,
et al., Biomimetic model to reconstitute angiogenic sprouting morphogenesis in vitro, Proc. Natl. Acad. Sci. U. S. A., 2013, 110(17), 6712–6717 CrossRef CAS PubMed.
- D. Huh, G. Hamilton and D. Ingber,
Program B, From three-dimensional cell culture to organs-on-chips, Dongeun Trends Cell. Biol., 2011, 21, 745–754 CrossRef CAS.
- D. Choudhury, S. Anand and M. W. Naing, The arrival of commercial bioprinters–Towards 3D bioprinting revolution, Int. J. Bioprint., 2018, 4(2), 139 Search PubMed.
- I. T. Ozbolat and Y. Yu, Bioprinting toward organ fabrication: challenges and future trends, IEEE Trans. Biomed. Eng., 2013, 60(3), 691–699 Search PubMed.
- C. Colosi,
et al., Microfluidic bioprinting of heterogeneous 3D tissue constructs using low–viscosity bioink, Adv. Mater., 2016, 28(4), 677–684 CrossRef CAS PubMed.
- F. Yu,
et al., A perfusion incubator liver chip for 3D cell culture with application on chronic hepatotoxicity testing, Sci. Rep., 2017, 7(1), 1–16 CrossRef.
- F. Yu,
et al., On chip two-photon metabolic imaging for drug toxicity testing, Biomicrofluidics, 2017, 11(3), 034108 CrossRef.
- J. Rouwkema and A. Khademhosseini, Vascularization and angiogenesis in tissue engineering: beyond creating static networks, Trends Biotechnol., 2016, 34(9), 733–745 CrossRef CAS PubMed.
- P. H. Dykstra, V. Roy, C. Byrd, W. E. Bentley and R. Ghodssi, Microfluidic electrochemical sensor array for characterizing protein interactions with various functionalized surfaces, Anal. Chem., 2011, 83(15), 5920–5927 CrossRef CAS PubMed.
- X. Luo,
et al., Programmable assembly of a metabolic pathway enzyme in a pre-packaged reusable bioMEMS device, Lab Chip, 2008, 8(3), 420–430 RSC.
- X. Luo,
et al., Design optimization for bioMEMS studies of enzyme-controlled metabolic pathways, Biomed. Microdevices, 2008, 10(6), 899–908 CrossRef CAS PubMed.
- Y. Cheng,
et al., Electroaddressing functionalized polysaccharides as model biofilms for interrogating cell signaling, Adv. Funct. Mater., 2012, 22(3), 519–528 CrossRef CAS.
- Z. Wang, L. He, J. Lv and M. Kimura, Electrodeposition of thin chitosan membrane in freestanding SU-8 microfluidic channel for molecular addressing by capillary effect, Mater. Res. Express, 2019, 6(4), 045403 CrossRef.
- K. Balagangadharan, S. Dhivya and N. Selvamurugan, Chitosan based nanofibers in bone tissue engineering, Int. J. Biol. Macromol., 2017, 104, 1372–1382 CrossRef CAS.
- M. Wang, Y. Xiao, L. Lin, X. Zhu, L. Du and X. Shi, A microfluidic chip integrated with hyaluronic acid-functionalized electrospun chitosan nanofibers for specific capture and nondestructive release of CD44-overexpressing circulating tumor cells, Bioconjugate Chem., 2018, 29(4), 1081–1090 CrossRef CAS.
- Q. Hamid, C. Wang, Y. Zhao, J. Snyder and W. Sun, A three-dimensional cell-laden microfluidic chip for in vitro drug metabolism detection, Biofabrication, 2014, 6(2), 025008 CrossRef CAS.
- K. A. Homan,
et al., Bioprinting of 3D convoluted renal proximal tubules on perfusable chips, Sci. Rep., 2016, 6, 34845 CrossRef CAS PubMed.
- S. Mi,
et al., A novel controllable cell array printing technique on microfluidic chips, IEEE Trans. Biomed. Eng., 2019, 66(9), 2512–2520 Search PubMed.
- N. S. Bhise,
et al., A liver-on-a-chip platform with bioprinted hepatic spheroids, Biofabrication, 2016, 8(1), 014101 CrossRef PubMed.
- J. Y. Park,
et al., Development of a functional airway-on-a-chip by 3D cell printing, Biofabrication, 2018, 11(1), 015002 CrossRef.
- Q. Gao,
et al., 3D bioprinting of vessel-like structures with multilevel fluidic channels, ACS Biomater. Sci. Eng., 2017, 3(3), 399–408 CrossRef CAS.
- Q. Hamid, C. Wang, J. Snyder, S. Williams, Y. Liu and W. Sun, Maskless fabrication of cell-laden microfluidic chips with localized surface functionalization for the co-culture of cancer cells, Biofabrication, 2015, 7(1), 015012 CrossRef.
- L. E. Bertassoni,
et al., Hydrogel bioprinted microchannel networks for vascularization of tissue engineering constructs, Lab Chip, 2014, 14(13), 2202–2211 RSC.
- S. Bakarich, R. Gorkin III, M. in het Panhuis and G. M. Spinks, ACS Appl. Mater. Interfaces, 2014, 6, 15998–16006 CrossRef CAS PubMed.
- Y. Luo, X. Lin, B. Chen and X. Wei, Cell-laden four-dimensional bioprinting using near-infrared-triggered shape-morphing alginate/polydopamine bioinks, Biofabrication, 2019, 11(4), 045019 CrossRef CAS PubMed.
- A. Lapomarda and G. Vozzi, 4D Bioprinting as New Tissue Engineering Perspective, Biosci., Biotechnol. Res. Asia, 2019, 16(1), 15 Search PubMed.
-
I. Lukin, et al., Can 4D bioprinting revolutionize drug development?, Taylor & Francis, 2019 Search PubMed.
- F. Ding, H. Li, Y. Du and X. Shi, Recent advances in chitosan-based self-healing materials, Res. Chem. Intermed., 2018, 44(8), 4827–4840 CrossRef CAS.
- F. Gang,
et al., Robust magnetic double-network hydrogels with self-healing, MR imaging, cytocompatibility and 3D printability, Chem. Commun., 2019, 55(66), 9801–9804 RSC.
- X. Chen,
et al., Magnetic and self-healing chitosan-alginate hydrogel encapsulated gelatin microspheres via covalent cross-linking for drug delivery, Mater. Sci. Eng., C, 2019, 101, 619–629 CrossRef CAS.
- B. Guo, J. Qu, X. Zhao and M. Zhang, Degradable conductive self-healing hydrogels based on dextran-graft-tetraaniline and N-carboxyethyl chitosan as injectable carriers for myoblast cell therapy and muscle regeneration, Acta Biomater., 2019, 84, 180–193 CrossRef CAS.
- B. Fu, B. Cheng, X. Bao, Z. Wang, Y. Shangguan and Q. Hu, Self–healing and conductivity of chitosan–based hydrogels formed by the migration of ferric ions, J. Appl. Polym. Sci., 2019, 136(34), 47885 CrossRef.
- T. Kean and M. Thanou, Biodegradation, biodistribution and toxicity of chitosan, Adv. Drug Delivery Rev., 2010, 62(1), 3–11 CrossRef CAS.
- A. Babu and R. Ramesh, Multifaceted applications of chitosan in cancer drug delivery and therapy, Mar. Drugs, 2017, 15(4), 96 CrossRef.
-
C. Chaput and A. Chenite, Injectable in situ self-forming mineral-polymer hybrid composition and uses thereof, ed: Google Patents, 2014 Search PubMed.
-
J.-B. D'Huart and C. Dallas, Cactaceae-based formulation having the property of fixing fats and method for obtaining same, ed: Google Patents, 2004 Search PubMed.
-
V. G. Varanasi, A. Ilyas, P. R. Kramer, T. Azimaie, P. B. Aswath and T. Cebe, In vivo live 3D printing of regenerative bone healing scaffolds for rapid fracture healing, ed: Google Patents, 2019 Search PubMed.
-
S. McCarthy, B. McGrath and E. Winata, Absorbable tissue dressing assemblies, systems, and methods formed from hydrophilic polymer sponge structures such as chitosan, ed: Google Patents, 2012 Search PubMed.
-
J. K. Smith, A. C. Parker, J. A. Jennings, B. T. Reves and W. O. Haggard, Methods for producing a biodegradable chitosan composition and uses thereof, ed: Google Patents, 2017 Search PubMed.
- X. Liu,
et al., Responsive Materials: 3D Printing of Living Responsive Materials and Devices (Adv. Mater. 4/2018), Adv. Mater., 2018, 30(4), 1870021 CrossRef.
-
C. A. Custódio, A. del Campo, R. L. Reis and J. F. Mano, Smart instructive polymer substrates for tissue engineering, in Smart Polymers and their Applications, Elsevier, 2019, pp. 411–438 Search PubMed.
- A. Lee,
et al., 3D bioprinting of collagen to rebuild components of the human heart, Science, 2019, 365(6452), 482–487 CrossRef CAS.
Footnote |
† Mosen Taghizadeh, Ali Taghizadeh and Mohsen Khodadadi Yazdi equally contributed to this work. Masoud Mozafari currently at Lunenfeld-Tanenbaum Research Institute, Mount Sinai Hospital, University of Toronto, Toronto, Canada. |
|
This journal is © The Royal Society of Chemistry 2022 |
Click here to see how this site uses Cookies. View our privacy policy here.